-
PDF
- Split View
-
Views
-
Cite
Cite
Ahmed Ismail, Michael Riemann, Peter Nick, The jasmonate pathway mediates salt tolerance in grapevines, Journal of Experimental Botany, Volume 63, Issue 5, March 2012, Pages 2127–2139, https://doi.org/10.1093/jxb/err426
- Share Icon Share
Abstract
Salt stress is a major constraint for many crop plants, such as the moderately salt-sensitive economically important fruit crop grapevine. Plants have evolved different strategies for protection against salinity and drought. Jasmonate signalling is a central element of both biotic and abiotic stress responses. To discriminate stress quality, there must be cross-talk with parallel signal chains. Using two grapevine cell lines differing in salt tolerance, the response of jasmonate ZIM/tify-domain (JAZ/TIFY) proteins (negative regulators of jasmonate signalling), a marker for salt adaptation Na+/H+ EXCHANGER (NHX1), and markers for biotic defence STILBENE SYNTHASE (StSy) and RESVERATROL SYNTHASE (RS) were analysed. It is shown that salt stress signalling shares several events with biotic defence including activity of a gadolinium-sensitive calcium influx channel (monitored by apoplastic alkalinization) and transient induction of JAZ/TIFY transcripts. Exogenous jasmonate can rescue growth in the salt-sensitive cell line. Suppression of jasmonate signalling by phenidone or aspirin blocks the induction of JAZ/TIFY transcripts. The rapid induction of RS and StSy characteristic for biotic defence in grapevine is strongly delayed in response to salt stress. In the salt-tolerant line, NHX1 is induced and the formation of reactive oxygen species, monitored as stress markers in the sensitive cell line, is suppressed. The data are discussed in terms of a model where salt stress signalling acts as a default pathway whose readout is modulated by a parallel signal chain triggered by biotic factors downstream of jasmonate signalling.
Introduction
Soil salinity poses a serious problem worldwide, causing global costs equivalent to an estimated US$11 000 million per year (FAO, 2011). Salt-affected soils negatively affect both the ability of crops to take up water and the availability of micronutrients, while they increase the concentration of toxic ions to plants, and may degrade soil structure. At the cellular and molecular levels, salt stress causes membrane disorganization, metabolic toxicity, formation of reactive oxygen species (ROS), inhibition of photosynthesis, and reduced nutrient acquisition (for reviews, see Hasegawa et al., 2000; Zhu, 2002). Plants have evolved many mechanisms to survive under salinity such as selective accumulation or exclusion of ions, synthesis of compatible solutes, sequestering of ions into separate compartments, induction of antioxidative enzymes, and adaptive regulation of plant hormones such as abscisic acid (ABA), ethylene (ET), and jasmonates (JAs) (for a review, see Parida and Das, 2005).
JA signalling (for reviews, see Wasternack, 2007; Avanci et al., 2010) has been extensively studied, using biochemical and molecular genetical approaches, in Arabidopsis thaliana and other species (Browse, 2009; Chini et al., 2009; Fonseca et al., 2009; for reviews, see Memelink, 2009; Reinbothe, 2009; Schaller and Stintzi, 2009). (+)-7-iso-Jasmonoyl-l-isoleucine (JA-Ile) generated by the JAR1 (Jasmonate-Resistant 1) enzyme represents the endogenous bioactive form of JAs (Staswick and Tiryaki, 2004; Staswick, 2008; Fonseca et al., 2009). Similar to gibberellin and auxin signalling, JA-dependent gene activation involves hormone-induced degradation of a transcriptional repressor, the jasmonate ZIM/tify-domain (JAZ/TIFY) proteins (Vanholme et al., 2007). To date, 12 JAZ/TIFY genes have been identified in A. thaliana (Chini et al., 2007). The products of these JAZ/TIFY genes share two conserved domains, a ZIM/tify (zinc-finger protein expressed in inflorescence meristem) and a Jas (jasmonate-associated) domain. The ZIM/tify domain mediates homo- and heteromeric hormone-independent interactions between individual JAZ/TIFY proteins (Chini et al., 2009). In contrast, the Jas domain is required for hormone-dependent interactions of JAZ/TIFY with MYC2 and COI1 (Chini et al., 2007; Thines et al., 2007) and for nuclear localization (Grunewald et al., 2009). In response to environmental or developmental signals that stimulate the biosynthesis of JAs, the elevated levels of JA-Ile promote the interaction of JAZ/TIFY repressors with SCFCoI1-mediated ubiquitination and subsequently degrade JAZ/TIFY proteins via the 26S proteasome, which in turn releases MYC2 and probably other transcription factors. Activation of MYC2 induces transcription of early JA-responsive genes including JAZ/TIFY genes themselves. The newly synthesized JAZ/TIFY proteins dimerize with MYC2, restore the repression of MYC2, and turn the pathway off (for reviews, see Chico et al., 2008; Chung et al., 2009). They also recruit the Groucho/Tup1-type co-repressor TOPLESS (TPL) and TPL-related proteins (TPRs) through a Novel Interactor of JAZ/TIFY (NINJA). NINJA acts as a transcriptional repressor whose activity is mediated by a functional TPL-binding EAR repression motif, and both NINJA and TPL proteins function as negative regulators of JA responses (Pauwels et al., 2010).
JAs are involved in plant responses to several abiotic and biotic stresses such as wounding (mechanical stress), drought stress, salt stress, ozone, pathogen infection, and insect attack. In addition, JAs regulate many aspects of plant development and growth such as seed germination, fruit ripening, production of viable pollen, root growth, tendril coiling, photomorphogenesis, leaf abscission, and senescence (Creelman and Mullet, 1995, 1997a, b; Conconi et al., 1996; Rao et al., 2000; Riemann et al., 2003; Haga and Iino, 2004; Ma et al., 2006; Robson et al., 2010; Wang et al., 2011).
The activity of JA responses is regulated by antagonistic cross-talk with salicylic acid (SA) signalling (for reviews, see Lorenzo and Solano, 2005; Balbi and Devoto, 2008). SA and its acetylated form aspirin can suppress the JA-dependent response to wounding and pathogen or insect attack in A. thaliana and tomato (Peña-Cortès et al., 1993; Leon-Reyes et al., 2010). In addition to SA, inhibitors of JA biosynthesis have been used to suppress JA-responsive genes. The redox-active compound 1-phenylpyrazolidinone (phenidone) inhibits the activity of lipoxygenases (LOXs) by reducing their active form to an inactive form. This inhibits the octadecanoid pathways at an early stage, and, thus, plant defence (Bruinsma et al., 2010a, b).
Due to these manifold functions, JAs are considered as a master switch for the adaptation to biotic and abiotic factors (for a review, see Wasternack and Hause, 2003). This leads to the central question of specificity: whether the cellular response to biotic attack is different from the response to abiotic stress. For instance, many plants respond by a plant-specific form of programmed cell death termed the hypersensitive reaction (Jones and Dangl, 2006). This response would be meaningless in response to salt stress. Adaptation to salt stress, in turn, was shown to involve NHX1, the plant homologue of the yeast Na+/H+ exchanger, suggested to catalyse Na+ accumulation in vacuoles (Gaxiola et al., 1999). In concert with other members of this family of transporters, NHX1 plays crucial roles in pH regulation and K+ homeostasis, regulating processes from vesicle trafficking and cell expansion to plant development (for a review, see Rodríguez-Rosales et al., 2009). Despite this divergence of downstream responses, biotic and abiotic stress signalling overlap during their early stages. For instance, calcium channels activated by mechanic stimulation (a typical indicator for wounding) have been shown to mediate, in addition, gene activation (MYP9, WRKY1, PR1, and PR2) through the pep-13 elicitor derived from an oomycete pathogen (Gus-Mayer et al., 1998). In addition, changes in apoplastic pH, one of the fastest known responses to biotic attack (Felix et al., 1993), are also observed in response to abiotic stresses including drought (for a review, see Felle et al., 2001).
At what point does the abiotic stress signal diverge from defence-related signalling? Is this branching point upstream or downstream of JA activity? To address these questions, two grapevine cell lines that differ in their drought tolerance were used. One line was derived from the wild North American grape Vitis rupestris inhabiting sunny rocks and slopes and therefore used in viticulture as a drought-tolerant rootstock, and the other cell line was generated from Vitis riparia, a North American species growing in alluvial forests and therefore not adapted to drought. Using these two systems, the role of the JA pathway for salt tolerance was analysed. It is shown that the two cell lines differ in their sensitivity to salt stress, correlated with expression of grapevine JAZ/TIFY and rapid responses of apoplastic pH. Markers for JA signalling, defence, salt adaptation, and oxidative stress in combination with inhibitors of JA synthesis and signalling link salt tolerance with the activity of the JA pathway. It can be further shown that the salt-sensitive V. riparia line can be rendered salt tolerant by addition of exogenous JA. Early events are shared between salt-triggered and defence-related signalling, whereas the downstream pattern of gene expression differs, leading to a model of a JA-dependent default pathway triggered by salt stress that is modulated by a parallel pathway activated by pathogen-derived factors.
Materials and methods
Cell lines and treatments
Suspension cell cultures of V. rupestris and V. riparia generated from leaves (Seibicke, 2002) were used in this experiment. They were cultivated in liquid medium containing 4.3 g l−1 Murashige and Skoog salts (Duchefa, Haarlem, The Netherlands), 30 g l−1 sucrose, 200 mg l−1 KH2PO4, 100 mg l−1 inositol, 1 mg l−1 thiamine, and 0.2 mg l−1 2,4-dichlorophenoxyacetic acid (2,4-D), pH 5.8. Cells were subcultured weekly; 10 ml of stationary cells were inoculated into 30 ml of fresh medium in 100 ml Erlenmeyer flasks. The cell suspensions were incubated at 25 °C in the dark on an orbital shaker (KS250 basic, IKA Labortechnik, Staufen, Germany) at 150 rpm. To induce cellular responses, cultures were treated at day 5 after subcultivation with different concentrations of sodium chloride (NaCl) aqueous solution, (±)-jasmonic acid (JA) (Sigma-Aldrich, Germany) dissolved in ethanol (EtOH), acetylsalicylic acid (aspirin) (Sigma-Aldrich, Germany) dissolved in dimethylsulphoxide (DMSO), an aqueous solution of the inhibitor 1-phenylpyrazolidinone (phenidone) containing 0.1% polyoxy-ethylene-orbitan monolaurate (Tween-20) (both obtained from Sigma-Aldrich, Germany), or Harpin elicitor [Messenger®, EDEN Bioscience Corporation, Washington, USA; active ingredient: 3% (w/w) Harpin protein]. Negative controls contained the corresponding concentrations of solvent without the active ingredient.
Measurement and quantitative analysis of pH and PCV responses to salt stress
pH changes were followed using a pH meter (Schott handylab, pH 12) connected to a pH electrode (Mettler Toledo, LoT403-M8-S7/120) and recorded by a paperless recorder (VR06; MF Instuments GmbH, Albstadt-Truchtelfingen, Germany) at 1 s intervals. Before treatments, 2 ml of suspension cells (3–4 d after subcultivation) were pre-adapted on an orbital shaker for ∼90 min until the pH was stable. To test the effect of salt on pH, cells were treated with NaCl at 0, 10, 20, 30, 40, 50, 85, 120, 155, 200, and 300 mM for 1 h. To block the induction of apoplastic pH, cells were pre-treated with different concentration of GdCl3 or water for 2 min before the addition of salt.
The pH data were exported to Microsoft Office Excel by the data-acquisition software Observer II_V2.35 (MF Instruments GmbH). The data were fitted based on a Michaelis–Menten equation with TpH50 as Vmax, EC25 as Km, and the concentration of NaCl as [S]. TpH50 was the time required to reach 50% of the maximal pH response. Consequently, the equation yielded Km as an estimate for the concentration causing 25% of the maximal response (EC25).
The packed cell volume (PCV) as a measure of growth was recorded in response to different concentrations of NaCl (0–200 mM) added directly at subculture. In some experiments, JA (30 μM) was added. The increment in PCV was used as measure of the growth response. Each data point represents the mean from three measurements collected 4 d after subcultivation.
Cloning, sequencing, and sequence analysis of JAZ/TIFY genes
Three putative full-length cDNA clones coding for the grapevine homologues of AtJAZ1, 2, and 3 (TIFY10a, 10b, and 6b, respectively) were isolated from V. rupestris using full-length primers (Supplementary Table S1 available at JXB online). A high-fidelity PCR system (Phusion™ DNA Polymerase, NEB) was used with the following PCR parameters: 30 s template initial denaturation at 98 °C, 10 s template denaturation at 98 °C, 15 s primer annealing at 63, 64.1, and 64.1 °C, respectively, and 40 s primer extension at 72 °C for 35 cycles, with a final 5 min extension step at 72 °C. The isolated sequences were compared with the database sequence using the BLAST program (Altschul et al., 1997). The three putative grapevine JAZ/TIFY homologues were inserted into the pJet1.2 vector (CloneJET™ PCR Cloning Kit, Fermentas) and transformed into competent Escherichia coli DH5α according to the manufacturer’ protocol, yielding pJet1.2 JAZ1/TIFY10a, JAZ2/TIFY10b, or JAZ3/TIFY6b that were isolated using the Roti®-Prep Plasmid MINI (Carl Roth, Karlsruhe, Germany) kit according to the supplier’s protocol. The three inserts were sequenced by a commercial provider (GATC, Konstanz, Germany) and submitted to GenBank under the accession nos JF900329 (VrJAZ1/TIFY10a), JF900330 (VrJAZ2/TIFY10b), and JF900331 (VrJAZ3/TIFY6b). Predicted protein sequences were aligned and analysed using ClustalX (Jeanmougin et al., 1998), and GeneDoc (Nicholas and Nicholas, 1997), and the tree was visualized with the Treeview program (http://taxonomy.zoology.gla.ac.uk/rod/rod.html).
Quantification of gene expression
Aliquots (1.5 ml) of cells collected at day 5 after subcultivation were treated with different concentrations of NaCl (0–200 mM) for 30 min and 1 h, sedimented by low-speed centrifugation (3000 rpm; 1 min), and shock-frozen in liquid nitrogen. Samples were homogenized with steel beads (Tissue Lyser, Qiagen/Retsch, Germany), and total RNA extracted using the innuPREP Plant RNA Kit (analytikjena, Jena, Germany) following the manufacturer’s protocol. The extracted RNA was treated with a DNA-free DNase (Qiagen, Hildesheim, Germany) to remove potential contamination by genomic DNA. The mRNA was transcribed into cDNA using the M-MuLV RTase cDNA Synthesis Kit (New England BioLabs; Frankfurt am Main, Germany) according to the manufacturer’s instructions.
To study gene expression by reverse transcription-PCR (RT-PCR), transcripts were amplified by PCR primers (Supplementary Table S1 at JXB online) with 30 cycles of 1 min denaturation at 94 °C, 30 s annealing at 60 °C (except with MYC2 which was at 63 °C), and 1 min synthesis at 72 °C. The PCR products were separated by conventional agarose gel electrophoresis after visualization with SybrSafe (Invitrogen, Karlsruhe, Germany). Images of the gels were recorded on a MITSUBISHI P91D screen (Invitrogen) using a digital image acquisition system (SafeImage, Intas, Germany). The bands of the amplificons were quantified using the Image J software (http://rsbweb.nih.gov/ij/) and normalized relative to elongation factor 1α as internal standard. The results were plotted as fold increase of transcript abundance as compared with the untreated control. For the time course, cells were treated with 155 mM NaCl for 1, 3, and 6 h, and then RNA was extracted. JAZ1/TIFY10a, COI1, MYC2, NHX1, STILBENE SYNTHASE (StSy), and RESVERATROL SYNTHASE (RS) expression was measured by RT-PCR at each time point.
To test the effect of JA on the induction of the three putative JAZ/TIFY genes, cells were pre-treated with 0, 10, 20, 30, 40, and 50 μM JA for 1 h or with corresponding concentrations of EtOH as a solvent control. Alternatively, 1 mM aspirin (Peña-Cortès et al., 1993) was added 6 h prior to addition of NaCl, and cells were harvested 1 h later. As a solvent control, corresponding concentrations of DMSO were added instead of aspirin. In a third set of experiments, cells were pre-treated with 2 mM phenidone for 30 min (Bruinsma et al., 2010a) prior to addition of 155 mM NaCl and then harvested 1, 3, and 6 h later. As a control, cells were pre-treated with 0.1% Tween-20 as control 1 or phenidone as control 2 for 30 min before 0 mM NaCl was added for 1 h. All quantifications of gene expression represent the mean from at least three independent experimental series.
Detection of oxidative burst in response to salt stress
ROS were detected under salt stress using dihydroethidium (DHE) according to Tarpey et al. (2004). DHE detects essentially superoxide radicals (O2•−). In brief, 1 ml of suspension cells was stabilized for at least 1 h in the culture shaker, and then 30 μM DHE was added. Cells were incubated with the dye for 3–7 min in a dark chamber, on an orbital shaker at room temperature. They were washed three times in MS medium in a dark chamber, on an orbital shaker at room temperature. NaCl (155 mM) was added immediately before observing cells under a fluorescent microscope (AxioImager Z.1, Zeiss, Jena, Germany) equipped with an ApoTome microscope slider through the filter set 43 HE (excitation at 550 nm, beamsplitter at 570 nm, and emission at 605 nm) for up to 6 h.
Statistical analysis
Three biological replicates were analysed for each treatment. Mean values and the SEM were calculated using Microsoft Excel. P-values for differences between treatments were tested by a paired Student t-test.
Results
Growth of the two cell lines shows differential NaCl sensitivity
The reference system for the present study was two grapevine cell lines from genotypes that differ in their sensitivity to drought. The NaCl sensitivity in these two cell lines was therefore characterized; a dose–response curve of cell growth (monitored as the increment of PCV, ΔPCV) over different concentrations of NaCl was established for both V. rupestris and V. riparia cells (Fig. 1A). Since growth under control conditions was higher in V. rupestris as compared with V. riparia (18.41 ml as compared with 10.3 ml), the increment had to be normalized to the control value. Cells were treated with 0.5–200 mM NaCl for 4 d following the weekly subculture. At low concentrations of NaCl (0–50 mM) the ΔPCV was progressively decreased. In none of the cell lines was any significant growth observed for concentrations of NaCl exceeding 50 mM. In the physiologically relevant dose range between 10 mM and 50 mM NaCl, V. rupestris cells grew better than V. riparia cells. In this dose range, the curve for V. rupestris was shifted by a factor of ∼1.5 to higher concentrations. To investigate the role of JA in the growth rate at physiologically relevant concentrations of NaCl, 30 μM JA was added (Fig. 1B, C). JA in the absence of salt stress reduced growth by ∼20% in both cell lines as compared with the control. In V. rupestris, exogenous JA did not cause significant changes in the response to salt (Fig. 1B). However, in V. riparia, JA increased ΔPCV significantly (P=0.05) over the corresponding values measured for salt treatment without JA supplementation (Fig. 1C). This suggests that JA ameliorates the negative impact of salt stress on cell growth.

Response of cell growth to NaCl in V. rupestris and V. riparia cells. (A) Increment of packed cell volume (ΔPCV) as a measure of cell growth with different concentrations of NaCl. The growth of the untreated control is defined as 100%. Values represent means and standard errors from three measurements. (B and C) Effect of 30 μM JA on NaCl-dependent inhibition of cell growth in V. rupestris (B) or V. riparia (C). Values represent means and standard errors from three individual experiments. The grey values represent the combinations of the respective salt concentration with JA; arrows indicate the values obtained for the JA treatment in the absence of salt.
NaCl induces extracellular alkalinization
Extracellular alkalinization is one of the earliest defence-related responses (Felix et al., 1993) and was therefore used to monitor potential differences in the response of the two Vitis cell lines to NaCl (Fig. 2). In both cell lines, the pH increased rapidly and culminated within 10–20 min after the addition of NaCl, and subsequently decreased. A representative time course is shown for V. rupestris in Fig. 2A. At higher concentrations, the maximum was reached later than with lower concentrations. In V. rupestris, the peaks were much more pronounced compared with V. riparia (Fig. 2B).
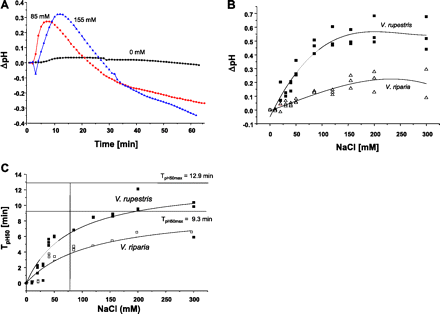
Response of apoplastic pH to NaCl in grapevine cells. (A) Representative time course for V. rupestris. (B) Dose–response curves for the maximal pH change with salt concentration. (C) Kinetic analysis of pH responses in V. rupestris (filled squares, solid lines) and V. riparia (open squares, dotted lines). The half-maxima of pH to increasing concentrations of NaCl were fitted by a Michaelis–Menten function. TpH50max is defined as time to reach 50% of the maximal response. (This figure is available in colour at JXB online.)
To characterize the difference between the two cell lines on a quantitative level, time courses were recorded by varying the concentration of salt. The results were fitted using a Michaelis–Menten equation with pHmax50 (the time when the pH response reached the half-maximum) as an indicator of velocity. When TpH50 was plotted over the concentration of NaCl (Fig. 2C), saturable curves were found that could be well fitted by a Michaelis–Menten function (R2=0.840 for V. rupestris and R2=0.882 for V. riparia). From these functions, effective concentrations (EC25, inducing 25% of the maximal response) could be calculated to be 80 mM NaCl for V. rupestris and 122 mM NaCl for V. riparia, respectively. This means that the responsiveness of V. rupestris is ∼1.5 times higher compared with V. riparia, consistent with the shift of the dose–response curves for cell growth (Fig. 1A). Since the apoplastic alkalinization triggered by biotic stress can be blocked by gadolinium ions (Qiao et al., 2010), the salt-induced pH response was assessed after pre-treatment with GdCl3. As shown in Supplementary Fig. S1 at JXB online, 500 μM could already inhibit salt-induced alkalinization in V. riparia. For V. rupestris, inhibition was observed at a somewhat higher (750 μM) concentration of GdCl3.
Isolation and Identification of JAZ genes
Based on the available sequences of A. thaliana JAZ/TIFY proteins, putative homologues in V. vinifera cv. ‘Pinot Noir’ were identified by a BLAST search. The phylogenetic tree based on the 12 available JAZ/TIFY protein sequences of A. thaliana and their nine putative JAZ/TIFY-LIKE (JAZL/TIFYL) homologues in V. vinifera cv. ‘Pinot Noir’ (Fig. 3A) showed five branches (I–V). Four of those contained members from both plant species; branch III did not contain a member from Vitis. All Vitis JAZL/TIFYL proteins contain a putative N-terminal ZIM/tify domain, and a putative C-terminal Jas domain as conserved domains. The consensus sequence for the ZIM/tify and Jas domains (Fig. 3B) was created using http://weblogo.berkeley.edu/logo.cgi. Three of these putative JAZ/TIFYL genes were successfully cloned from the V. rupestris cell line using RT-PCR with degenerated primers (Supplementary Table S1 at JXB online). Alignment of the three proteins (Fig. 3C) showed that two of those, VrJAZ1/TIFY10a (GenBank accession no. JF900329) and VrJAZ2/TIFY10b (GenBank accession no. JF900330) were fairly similar and belonged to the same branch of the tree (I, Fig. 3A), whereas the third, VrJAZ3/TIFY6b (GenBank accession no. JF900331) was longer and belonged to a different branch (IV, Fig. 3A). The sequences from V. rupestris were almost identical to those from V. vinifera cv. ‘Pinot Noir’ (only 2–3 amino acid exchanges in the variable regions of the JAZ/TIFYL proteins).
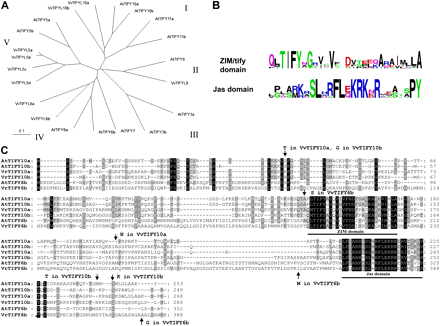
In silico analysis of Vitis JAZ/TIFY homologues. (A) Phylogenetic tree based on Neighbor-Joining (NJ) of available JAZ/TIFY sequences from Arabidopsis thaliana and their JAZ-LIKE/TIFY-LIKE (JAZL/TIFYL) homologues in Vitis vinifera cv. ‘Pinot Noir’. NJ, bootstrap values 0.1. (B) Consensus motifs. (C) Alignment with AtJAZ/TIFY-homologues (the sequences of Vr and Vv are almost identical); arrows indicate amino acid differences between V. rupestris and V. vinifera cv. ‘Pinot Noir’. (This figure is available in colour at JXB online.)
Impact of NaCl, JA, and aspirin on the expression of JAZ/TIFY genes
Since the three proteins (termed as JAZ1–3/TIFY10a, b, 6b based on their homology to AtJAZ/TIFY proteins) seemed to be the dominant members in V. rupestris cells, their expression was investigated under salt-stress by RT-PCR using elongation factor 1α as the internal reference for quantification. The dose–response dependency of transcript abundance was determined 30 min and 1 h after the addition of NaCl. The preliminary work showed that the expression of JAZ1/TIFY10a after 30 min was very weak in both cell lines (Supplementary Fig. S2 at JXB online). The results were in agreement with the expression of defence genes under Harpin treatment (Qiao et al., 2010). In contrast, all three genes were induced after addition of salt for 1 h (Fig. 4A shows a representative concentration series; Supplementary Table S2A shows the quantification) with a peak at concentrations around 80–120 mM and a decrease for higher salt concentrations. JAZ1/TIFY10a showed significant (Fig. 4A) induction in V. rupestris under salt stress as compared with V. riparia. The same was observed for JAZ3/TIFY6b. JAZ2/TIFY10b was slightly induced by salt by a similar factor in both cultivars (Fig. 4A; Supplementary Table S2A). These results show a significant (by a factor of ∼2) salt induction of JAZ1/TIFY10a and JAZ3/TIFY6b in V. rupestris, which parallels the salt tolerance of growth and the induction of extracellular alkalinization.
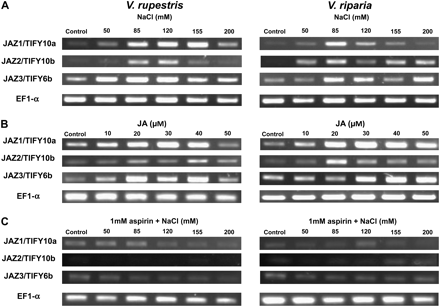
Response of JAZ/TIFY genes in V. rupestris and V. riparia to different concentrations of NaCl (A) and JA (B) for 1 h, or to NaCl for 1 h after pre-treatment with aspirin (1 mM) (C) for 6 h. Elongation factor 1α (EF1-α) was used as the internal standard. One representative example of three independent experiments is shown.
To test whether these JAZ/TIFY genes respond to JA, cells were treated for 1 h with different concentrations (0–50 μM) of JA. As a solvent control, cells were treated with a corresponding concentration of EtOH. Figure 4B shows a representative dose series, and Supplementary Table S2B at JXB online shows the quantification. JAZ1/TIFY10a as well as JAZ3/TIFY6b was induced with the increase in the JA concentration to 30 μM, with a tendency to decrease at 40 μM and 50 μM in both cell lines (Fig. 4B; Supplementary Table S2B). JAZ2/TIFY10b was only very weakly expressed. Both cell lines responded in a similar way; the difference in induction observed for NaCl (Fig. 4A; Supplementary Table S2A) was not manifest in their response to JA.
To test whether the induction of JAZ/TIFY transcripts by salt can by suppressed by JA antagonists, cells were treated with the same concentrations of NaCl for 1 h after pre-treatment with 1 mM aspirin or a corresponding amount of its solvent solution (DMSO) as a control for 6 h. The induction of these JAZ/TIFY genes by salt treatments was completely suppressed by pre-treatment with aspirin (Fig. 4C shows a representative dose series; Supplementary Table S2C at JXB online shows the quantification).
Thus, JAZ1/TIFY10a and JAZ3/TIFY6b are significantly induced by NaCl in a concentration-dependent manner. This induction is more pronounced in V. rupestris as compared with V. riparia, especially in the physiologically relevant range around 100 mM NaCl. Both JAZ/TIFY gene transcripts are induced by JA, with the highest expression at 30 μM and without any significant difference between the two cell lines. Aspirin can suppress the induction of JAZ/TIFY transcripts by salt, indicating that the response of transcription requires JA signalling.
Time courses of gene induction by salt
To gain insight into the sequence of cellular events, the expression of three marker genes for JA signalling (JAZ1/TIFY10a, MYC2, and COI1), a marker for salt adaptation (NHX1), and two markers of biotic defence (phytoalexin synthesis genes StSy and RS) was measured at a concentration of 155 mM NaCl, at which concentration JAZ1/TIFY10a was significantly expressed (Fig. 5 shows a representative time series; Supplementary Table S3 at JXB online shows the quantifications). In both cell lines, induction of JAZ1/TIFY10a was clearly manifest 1 h after addition of NaCl. However, it was significant in V. rupestris, and even doubled during the following 2 h, declining subsequently, while in V. riparia the induction was much weaker and did not increase after 1 h (Fig. 5; Supplementary Table S3). In contrast, MYC2 slightly increased after 1 h and then declined over time in both cell lines. COI1, encoding the JA receptor, seemed to be increased between 1 h and 3 h after addition of salt (Fig. 5), but this response was not significant (Supplementary Table S3). In contrast, NHX1, as a marker for salt adaptation, was clearly induced in V. rupestris from 3 h (∼3-fold at 6 h) which was not observed in V. riparia. The stilbene-related genes StSy and RS, widely used as markers for biotic defence in Vitis, did not respond to salt in V. riparia. However, they were clearly induced in V. rupestris from 3 h after addition of salt, especially RS. These results show a stronger induction of JAZ1/TIFY10a correlated with elevated induction of NHX1 (salt adaptation) and RS/StSy (phytoalexin, biotic defence) in V. rupestris as compared with V. riparia.
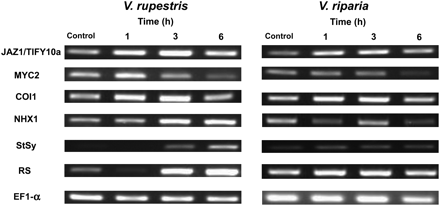
Time course of induction for transcripts of JAZ1/TIFY10a, MYC2, COI1, NHX1, StSy, and RS by 155 mM NaCl in V. rupestris and V. riparia. Elongation factor 1α (EF1-α) was used as internal standard. One representative example of three independent experiments is shown.
Phenidone can inhibit the induction of JAZ1/TIFY10a genes by salt
To investigate further whether the induction of JAZ1/TIFY10a by salt requires JA, the synthesis of JA was blocked by phenidone, an inhibitor of LOXs that trigger early steps in the octadecanoid pathway. Vitis cells were pre-treated with 2 mM phenidone for 30 min before adding 155 mM NaCl for 1, 3, and 6 h. As a control, cells were pre-treated with 2 mM phenidone or 0.1% Tween-20 for 30 min before 0 mM NaCl was added for 1 h (Fig. 6). Phenidone inhibited the induction of JAZ1/TIFY10a by salt stress very efficiently, especially in V. rupestris. Similar to the results obtained for aspirin (Fig. 4C), this result suggests that JA signalling (more specifically JA synthesis) is necessary to mediate the induction of JAZ1/TIFY10a expression in response to NaCl.
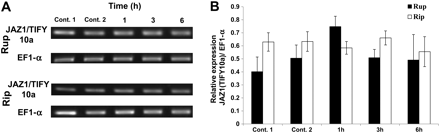
Effect of phenidone on the induction of JAZ1/TIFY10a transcripts under salt stress at different time points in V. rupestris (Rup) and V. riparia (Rip). (A) Representative time course of transcript abundance of cells pre-treated with phenidone for 30 min in response to 155 mM NaCl for 1, 3, and 6 h. Cells were pre-treated with 0.1% Tween-20 as control 1 or phenidone as control 2 for 30 min before 0 mM NaCl was added for 1 h. (B) Time course of transcript abundance of JAZ1/TIFY10a in both cultivars relative to elongation factor 1α (EF1-α). The data represent averages from three independent experimental series; error bars represent standard errors.
NaCl induces ROS
To test for the negative impact of NaCl on Vitis cells, ROS as an indicator of cellular stress were visualized by DHE. DHE is a cell-permeable compound that upon entering the cells interacts with O2•−, considered to be the earliest ROS to be generated, to form oxyethidium, which in turn interacts with nucleic acids to emit a bright red colour detectable as red fluorescence (Tarpey et al., 2004). The induction of ROS species was monitored over 6 h in both cell lines. Elevated levels of ROS were induced by salt stress in both cultivars (Fig. 7). However, ROS induction commenced earlier (from 1 h) and reached much higher levels in V. riparia as compared with V. rupestris, where the earliest indications of elevated ROS levels were not visible before 3 h after addition of salt. Thus, the amplitude and onset of the oxidative burst clearly behave antagonistically to the other tested events (extracellular alkalinization, induction of JAZ/TIFY, induction of NHX1, induction of RS, and growth), because here the response is most pronounced in V. riparia, but not in V. rupestris.
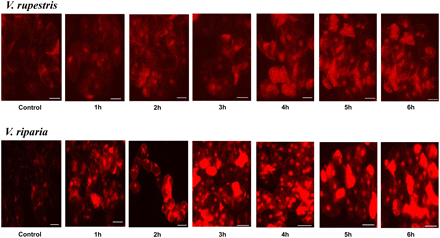
Effect of 155 mM NaCl on ROS induction in 5-day-old suspension cells of V. rupestris an V. riparia. Cells were stained with dihydroethidium (DHE), which records superoxide radicals (O2•−). NaCl (155 mM) was added to cells pre-loaded with the dye immediately before microscopic observation, and the signal was followed over 6 h. Size bar 20 μm. (This figure is available in colour at JXB online.)
JAZ1/TIFY10a is induced during biotic stress
Since JA has been shown to trigger phytoalexin synthesis in Vitis (Tassoni et al., 2005), a typical biotic stress response, and since salt stress was found to induce RS and StSy in V. rupestris, tests were carried out to determine whether JAZ1/TIFY10a can be induced in the absence of salt by triggering biotic defence. For this purpose the elicitor Harpin that, in V. rupestris, can induce the entire programme of defence (Qiao et al., 2010), was used. In fact, a clear induction of JAZ1/TIFY10a (Fig. 8) could be observed to a level similar to that observed after treatment with NaCl (Fig. 5; Supplementary Table S3 at JXB online). Also MYC2 showed a slight induction, similar to that observed after salt treatment (Fig. 5; Supplementary Table S3). Thus, the primary events of biotic and salt signalling including extracellular alkalinization, induction of JAZ1/TIFY10a, and (slight) induction of MYC2, seem to be very similar.
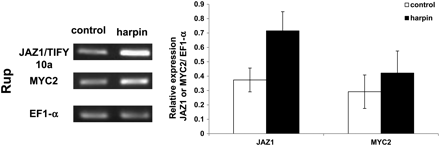
Effect of Harpin elicitor on the induction of JAZ1/TIFY10a and MYC2 transcripts in V. rupestris (Rup). Cells were treated with 9 μg ml−1 active elicitor for 1 h. The transcript abundance of JAZ1/TIFY10a and MYC2 is given relative to elongation factor 1α (EF1-α). The data represent averages of three independent experimental series; error bars represent standard errors.
Discussion
Adaptive response versus cellular damage
The following cellular events were observed in response to salt stress (Fig. 9): extracellular alkalinization, which can be inhibited by gadolinium ions, induction of JAZ1/TIFY10a and JAZ3/TIFY6b, (weak) induction of MYC2 and COI1, induction of NHX1, induction of RS, formation of ROS, and salt tolerance monitored as an increase in PCV despite challenge by NaCl. These responses could either be adaptive or they could merely report cellular damage caused by NaCl.
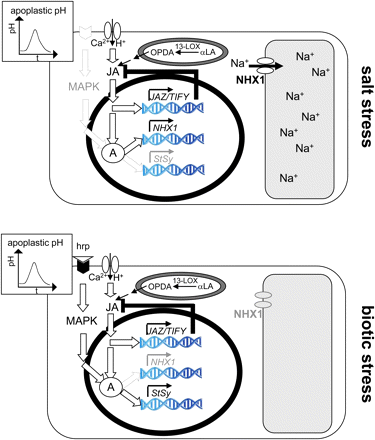
Model for the signalling induced by salt stress and biotic stress (as induced by the Harpin elicitor) in grapevine. Csk, cytoskeleton; hrp, Harpin elicitor; hrpR, putative Harpin receptor; msc, putative mechanosensitive channel; ΔΨ, gradient in water potential between the apoplast and cytoplasm triggering salt signalling; 13-LOX, 13-lipoxygenase species triggering jasmonate synthesis by converting α-linoleic acid (αLA); JA, jasmonate-dependent signaling; A, a hypothetical factor required for the activation of defence and salt adaptive genes. For details refer to the Discussion. (This figure is available in colour at JXB online.)
For instance, both biotic and abiotic stress factors induce an oxidative burst. The resulting ROS are highly destructive and can damage DNA, proteins, lipids, and carbohydrates (for a review, see Gill and Tuteja, 2010). On the other hand, ROS can be important signal transduction molecules acting in stress adaptation (for a review, see Miller et al., 2010).
To discriminate between adaptive and damage events, two grapevine cell lines were used that were expected to differ in their salt tolerance, because they originated from two species with different humidity requirements. In fact, the growth of V. rupestris cells was found to be more tolerant to NaCl by a factor of 1.5 compared with V. riparia. Based on this reference, adaptive responses should be more pronounced in V. rupestris, whereas events found to be stronger in V. riparia are likely to report damage rather than adaptation. In fact, extracellular alkalinization was stronger in V. rupestris. The same was true for the induction of JAZ1/TIFY10a, JAZ3/TIFY6b, NHX1, and RS. In contrast, generation of ROS was stronger in V. riparia, suggesting that this oxidative burst represents a manifestation of cellular damage rather than an adaptive response.
JA signalling is necessary and sufficient for JAZ/TIFY induction and salt adaptation
JA signalling plays a crucial role in plant responses to biotic and abiotic stress factors, such as wounding, drought, ozone exposure, pathogen infection, and insect attack (for reviews, see Wasternack, 2007; Avanci et al., 2010). The biosynthetic and signalling pathways underlying these responses have been intensively studied during the last decades. Recently, JAZ/TIFY repressor proteins have been identified as key regulators of JA signalling (Chini et al., 2007; Thines et al., 2007). Three representatives of the Vitis JAZ/TIFY family (Fig. 3) were therefore cloned and their expression was followed under NaCl stress (Fig. 4A). A transient induction of two JAZ/TIFY members (JAZ1/TIFY10a and JAZ3/TIFY6b) was observed that was more pronounced in V. rupestris (Fig. 4). In parallel, MYC2, a transcription factor whose activity is blocked in the absence of JA-Ile by dimerization with JAZ/TIFY proteins, is slightly elevated, as is the JA receptor gene COI1, whose transcription is induced in response to JAs. Since the activity of JA signalling is primarily regulated at the post-translational level (e.g. by proteolytic degradation of the inhibitory JAZ/TIFY proteins), these transcript responses of JAZ1/TIFY10a and JAZ3/TIFY6b as well as of MYC2 and COI1 are probably not directly involved in the signalling per se, but must be interpreted as elements in the complex feedback regulation that tunes the competence for JA signalling with the activity of the signalling pathway (Fig. 9). Nevertheless, these responses can be used as a readout to monitor the activity of JA signalling.
The differential responsiveness of JAZ/TIFY in the two cell lines could be caused either by differential activation of JA synthesis or, alternatively, by differential JA sensitivity of signalling. To differentiate between these two possibilities, the cell lines were treated with exogenous JA. The application of JA could mimick the effect of salt stress on the induction of JAZ/TIFY transcripts (Fig. 4B), with virtually the same response observed for both cell lines. This suggests that the signalling evoked by JA proceeds in the same manner and with the same intensity. Thus, the differences in salt-induced JAZ/TIFY induction must reside upstream of JA signalling, for instance in different levels of JA synthesis. The salt tolerance of growth in the sensitive V. riparia line can be rescued by exogenous JA (Fig. 1C), supporting a model where the activation of JA synthesis probably induces the adaptation to salt stress.
Pre-treatment with aspirin (Fig. 4C) or phenidone (Fig. 6A, B) was able to suppress the induction of JAZ/TIFY genes in response to salt stress, suggesting that salt-induced JA signalling is necessary for the JAZ/TIFY response. Both agents have been used to silence JA-dependent signalling, but have a different mode of action (Fig. 9): SA and its acetylated derivative, aspirin, seem to act as antagonists of JA signalling (reviewed in Lorenzo and Solano, 2005; Balbi and Devoto, 2008), whereas phenidone is thought to block JA synthesis through inhibition of the LOX that converts α-linolenic acid (Bruinsma et al., 2010a, b).
Since aspirin and phenidone inhibit JA signalling by different modes of action, the observed suppression of NaCl-induced induction of JAZ/TIFY transcripts is unlikely to be caused by unspecific side effects of the two compounds but rather by their inhibition of the JA signalling pathway. Thus, JA signalling is necessary and sufficient for JAZ/TIFY induction and cellular adaptation in the context of salt stress.
Salt and defence signalling share several upstream events
Salt signalling shares several events with biotic defence, including apoplastic alkalinization (Fig. 2) that is sensitive to gadolinium (Supplementary Fig. S1 at JXB online), and transient induction of JAZ/TIFY transcripts (Figs 4, 8). Apoplastic pH has been used to monitor rapid defence responses upstream of gene expression (Felix et al., 1993; Felle and Hanstein, 2002). A widely accepted model (for a review, see Scheel, 1998) proposes that pathogen-derived elicitors bind to a host receptor activating calcium influx. Together with calcium, protons can permeate from the acidic apoplast into the neutral cytoplasm (Jabs et al., 1997). The resulting apoplastic alkalinization is therefore a readout for the activity of the calcium channel, but does not convey defence signalling itself (Fig. 9). The time course of apoplastic alkalinization under salt stress differed from that observed after Harpin elicitation. After Harpin treatment, the pH increased directly, whereas, in the case of salt stress, it first showed a transient reduction of pH before returning to the initial level and subsequently increasing. This transient drop in pH reduction was more pronounced with increasing salt concentration, such that a longer time was required to reach the initial level and to launch the pH increase. Since the proton influx underlying apoplastic alkalinization is linked to the activity of a mechanosensitive calcium channel (Gus-Mayer et al., 1998), it is expected to close due to relaxation of membrane strains as a result of plasmolytic shrinkage in the hyperosmotic solution. This would account for the initial decrease. After activation of the channel, a certain time is required until the initial pH is established, such that alkalinization can become manifest. In the V. rupestris cell line, the activation of apoplastic alkalinization by the Harpin elicitor could be blocked by gadolinium, a classical inhibitor of mechanosensitive calcium channels (Qiao et al., 2010). Gadolinium sensitivity could be confirmed also for salt-induced alkalinization (Supplementaty Fig. S1 at JXB online), suggesting that the same mechanosensitive calcium channel is at work. Consistent with this conclusion, in self-reporting A. thaliana cells expressing green fluorescent protein (GFP)-based pH and Ca2+ indicators, salt and drought stress were shown to trigger apoplastic alkalinization as well as calcium influx (Gao et al., 2004). Activation of calcium influx is followed by activation of JA synthesis (blocked by phenidone) and JA signalling (blocked by aspirin), culminating in the induction of JAZ/TIFY transcripts. All of these early events are more pronounced in V. rupestris as compared with V. riparia on the background of a comparable JA responsiveness of JA signalling (Fig. 4B). This indicates that the difference between the two cell lines resides in the sensitivity of osmotic sensing. Whether V. rupestris is responding more efficiently because it harbours a higher density of mechanosensitive calcium channels or whether the activity of the individual channels is elevated remains to be elucidated.
Salt and defence signalling diverge in their downstream events
Whereas early responses of transcription to salt and Harpin (JAZ1/TIFY10a and MYC2) showed a similar pattern (compare Figs 5 and 8), there were clear differences in the expression of downstream genes. For instance, transcripts for the phytoalexin synthesis genes StSy and RS were induced much later after salt stress (from 3 h) as compared with a much earlier induction (∼0.5 h) after Harpin treatment (Qiao et al., 2010). Moreover, the pattern of transcript abundance is inverted; whereas in the case of Harpin StSy is most responsive, for salt stress it was RS that showed the strongest induction. These differences suggest that both kinds of stresses use separate pathways. In addition to phytoalexin synthesis, salt stress initiates adaptive processes, such as the synthesis of NHX1 that is observed from 3 h in V. rupestris. NHX1 sequestrates Na+ into the vacuole and thus contributes to osmotic adjustment by maintaining water uptake under saline conditions (for a review, see Zhu, 2003). As a consequence of this adaptation, cellular damage monitored by fluorescent detection of superoxide radicals was clearly ameliorated in V. rupestris as compared with V. riparia, where superoxide radicals were dramatically increased from 2 h after the onset of salt stress (Fig. 7). Again, this response clearly diverges from the situation observed for biotic stress: in the V. rupestris cell line, Harpin treatment was found to induce a massive oxidative burst. This seems to be connected to the oxidation of resveratrol into its highly toxic derivative δ-viniferin (Chang et al., 2011), probably involved in the execution of hypersensitive cell death. Programmed cell death is an efficient strategy to block a biotic intruder, but would be completely inappropriate for salt adaptation.
When salt and biotic signalling overlap in their upstream events (gadolinium-sensitive calcium influx accompanied by apoplastic alkalinization and induction of JA signalling), but differ in their downstream output (salt stress producing induction of salt sequestration, late induction of stilbene metabolism, predominance of RS, and suppression of the oxidative burst; and Harpin-triggered biotic signalling producing early induction of stilbene metabolism, predominance of StSy, and induction of the oxidative burst), there must be a parallel pathway activated during biotic signalling that modulates the transcriptional responses to JA signalling (Fig. 9). A good candidate is the mitogen-activated proten (MAP) kinase pathway, which is triggered by Harpin independently of calcium influx (Lee et al., 2001). A simple mechanism to switch between salt adaptation and biotic defence would be the JA-dependent formation or activation of a transcriptional regulator (depicted as A in Fig. 9) that is recruited to JA-responsive genes acting in salt adaptation, but can form a complex with the output from the biotically triggered MAP kinase pathway and will then be recruited to JA-responsive genes acting in biotic defence. Thus, salt signalling would act as a default pathway that can be modulated by biotic signalling towards activation of defence genes.
AI was supported by the German Egyptian Research Long-term Scholarship ‘GERLS’ program. Dr Hanns-Heinz Kassemeyer (State Institute of Viticulture, Freiburg) is acknowledged for the kind gift of the cell lines, and Sabine Purper for competent support in the maintenance of the cell lines.
Comments