-
PDF
- Split View
-
Views
-
Cite
Cite
Nenghui Ye, Guohui Zhu, Yinggao Liu, Aying Zhang, Yingxuan Li, Rui Liu, Lu Shi, Liguo Jia, Jianhua Zhang, Ascorbic acid and reactive oxygen species are involved in the inhibition of seed germination by abscisic acid in rice seeds, Journal of Experimental Botany, Volume 63, Issue 5, March 2012, Pages 1809–1822, https://doi.org/10.1093/jxb/err336
- Share Icon Share
Abstract
The antagonism between abscisic acid (ABA) and gibberellin (GA) plays a key role in controlling seed germination, but the mechanism of antagonism during this process is not known. The possible links among ABA, reactive oxygen species (ROS), ascorbic acid (ASC), and GA during rice seed germination were investigated. Unlike in non-seed tissues where ROS production is increased by ABA, ABA reduced ROS production in imbibed rice seeds, especially in the embryo region. Such reduced ROS also led to an inhibition of ASC production. GA accumulation was also suppressed by a reduced ROS and ASC level, which was indicated by the inhibited expression of GA biosynthesis genes, amylase genes, and enzyme activity. Application of exogenous ASC can partially rescue seed germination from ABA treatment. Production of ASC, which acts as a substrate in GA biosynthesis, was significantly inhibited by lycorine which thus suppressed the accumulation of GA. Consequently, expression of GA biosynthesis genes was suppressed by the low levels of ROS and ASC in ABA-treated seeds. It can be concluded that ABA regulates seed germination in multiple dimensions. ROS and ASC are involved in its inhibition of GA biosynthesis.
Introduction
Seed germination incorporates events that commence with the uptake of water (imbibition) by the quiescent dry seed and terminates with the emergence of the embryonic axis, usually the radicle (Bewley, 1997). It has been well documented that abscisic acid (ABA) and gibberellin (GA) have antagonistic roles in the regulation of seed germination (Koornneef et al., 2002; Finch-Savage et al., 2006). ABA auxotrophs of many plant species exhibit enhanced germination potential and sometimes produce viviparous seeds (McCarty, 1995; Salaita et al., 2005), whereas mutants and transgenic lines that overaccumulate ABA show enhanced dormancy or delayed germination (Thompson et al., 2000; Qin and Zeevaart, 2002; Okamoto et al., 2006, 2010). On the other hand, inhibitors of GA biosynthesis, such as paclobutrazol and diniconazole, reduce seed germination in Arabidopsis (Jacobsen and Olszewski, 1993; Leon-Kloosterziel et al., 1996; Toh et al., 2008). The inhibition of seed germination by a low concentration of ABA is reversed by GA (Liu et al., 2010). Using an ABA-deficient mutant aba2-2, Seo et al. (2006) showed that ABA was involved in the suppression of GA biosynthesis in both imbibed and developing seeds. ABA is also reported to inhibit seed germination by inhibiting GA biosynthesis directly under high temperature (Gonai et al., 2004; Toh et al., 2008). Such an antagonistic effect between ABA and GA plays a key role in controlling seed germination (Karssen and Lacka, 1986; Jia et al., 2001; Kucera et al., 2005; Seo et al., 2009).
Reactive oxygen species (ROS), including the superoxide anion radical (O2−), hydrogen peroxide (H2O2), the hydroxyl radical (·OH), and singlet oxygen (1O2), are metabolic by-products in both plants and animals. Although the toxicity of ROS is well documented, cellular antioxidant mechanisms seem to control ROS concentrations tightly, rather than to eliminate them completely, suggesting that some ROS play physiological roles and act as signalling molecules (Bailly, 2004). Indeed, since Prasad et al. (1994) first showed that H2O2 is involved in plant tolerance to various abiotic stresses, increasing evidence shows that ROS function as signalling molecules during plant growth and development, and plant defence against various abiotic and biotic stresses (Passardi et al., 2004; Rodriguez et al., 2004; Gechev et al., 2006; Jones et al., 2007; Zhang et al., 2010).
In seed biology, ROS also play a key role in seed dormancy alleviation, after-ripening, and germination (Oracz et al., 2007; El-Maarouf-Bouteau and Bailly, 2008). Seeds of the atrbohB mutant fail to after-ripen due to its blocked ROS production (Razem and Bernards, 2003; Müller et al., 2009). Inhibition of ROS production by diphenylene iodonium (DPI) leads to a delay in Arabidopsis and cress seed germination and cress endosperm weakening (Müller et al., 2009). H2O2 is reported to mediate the regulation of ABA catabolism and GA biosynthesis during dormancy alleviation and seed germination in Arabidopsis (Liu et al., 2010). These results indicate that ROS can be good candidates in mediating hormonal interactions.
Since its discovery in the late 1920s, probably no other chemical has ever been as celebrated as ascorbic acid (ASC) (Davies et al., 1991). Besides its most famous properties of an antioxidant, the question ‘what is the function of ASC?’ is still far from being answered (Arrigoni and De Tullio, 2002; Herschbach et al., 2010). In orthodox seeds, neither ASC nor ASC peroxidase activity exist at the quiescent stage, but they re-start after a few hours from the onset of imbibition (Tommasi et al., 1999; De Tullio and Arrigoni, 2003). Many investigations suggest that the capability of rapid re-starting of both ASC biosynthesis and ASC peroxidase activity is crucial to ensure seed germinability (De Gara et al., 1991, 2000; Innocenti et al., 1994). However, there is other research demonstrating that a high dose ASC treatment can induce cell death in mesothelioma cells and suppress germination in wheat seeds (Ishibashi and Iwaya-Inoue, 2006; Takemura et al., 2010). These opposite effects of ASC on seed germination suggest that the production of ASC in seed must be finely controlled or regulated.
Beside its antioxidant properties, recent research has revealed another role for ASC; that is, as a substrate for many 2-oxoacid-dependent dioxygenases (2-ODDs) (Prescott and John, 1996; Pastori et al., 2003). The 2-ODDs include a number of dioxygenases, which are involved in the synthesis of the plant hormones ethylene, GA, and ABA, indicating a possible link between ASC and plant hormones (De Tullio and Arrigoni, 2003).
Previous work mainly focused on the antagonistic relationship between ABA and GA during seed germination, but the mechanism of the hormone interaction is not yet apparent. Both ROS and ASC are suggested to be important in seed germination. However, their roles in hormone interactions, especially in the antagonism between ABA and GA, have not been investigated. Thus, the relationships among ABA, GA, ROS, and ASC during seed germination are still not clear. In this study, by manipulating the ROS and ASC contents in rice seed, it was found that ASC, together with ROS, mediates the inhibition of GA accumulation by ABA during seed germination in rice. The results have proved the hypothesis that ASC is not just an antioxidant and that both ROS and ASC play a pivotal role in mediating phytohormone interactions during seed germination. Suppression of their production can lead to inhibition of seed germination.
Materials and methods
Plant materials and germination
Rice seeds (Oryza sativa L. cv. Yangdao 6) were used. Dehulled rice seeds, the caryopses, were directly sown on sterile filter paper which contained different concentrations of ABA and ASC. In the pharmacological experiment, diniconazole at 10 μM, DPI at 10 μM, or Tiron at 10 mM was used. Seeds were placed in a growth chamber in continuous darkness at 28 °C to facilitate germination. Germination (based on radicles >2 mm) was recorded every 12 h or daily, depending on the experiment. Each plate contained 40 seeds. Every experiment was repeated three times. Seeds imbibed for 1, 3, 6, 12, 24, 36, and 48 h in the presence of water or treatments were collected and stored at –80 °C for ABA determination or RNA isolation. ROS detection was performed immediately after the collection of seed samples.
Determination of ABA content
For estimation of endogenous ABA levels of imbibed seeds, 0.2 g of rice seeds were homogenized in 1 ml of distilled water and then shaken at 4 °C overnight. The homogenates were centrifuged at 12 000 g for 10 min at 4 °C and the supernatant was directly used for ABA assay. ABA analysis was carried out using the radioimmunoassay (RIA) method as described by Quarrie et al. (1988). The 450 μl reaction mixture contained 200 μl of phosphate buffer (pH 6.0), 100 μl of diluted antibody (Mac 252) solution, 100 μl of [3H]ABA (∼8000 cpm) solution, and 50 μl of crude extract. The mixture was then incubated at 4 °C for 45 min and the bound radioactivity was measured in 50% saturated (NH4)2SO4-precipitated pellets with a liquid scintillation counter (Ren et al., 2007).
RNA isolation and quantitative real-time (qRT)-PCR
Total RNA was extracted from rice seeds with a Plant RNA Isolation Mini Kit (Agilent, Wilmington, DE, USA) and then digested with RNase-free DNase I (GE Healthcare, Uppsala, Sweden) to eliminate genomic DNA contamination. First-strand cDNA was synthesized with oligo(dT) primers using a SuperScript first-strand synthesis system according to the manufacturer’s instructions (Invitrogen, Carlsbad, CA, USA). Transcript levels of each gene were measured by qRT-PCR using a Mx3000p QPCR System (Agilent) with iQ SYBR Green Supermix (Bio-Rad, Hercules, CA, USA). The data were normalized to the amplification of a rice ACTIN gene. For each sample, the mean value from three qRT-PCRs was adopted to calculate the transcript abundance, and the mean values were then plotted with the SD. Primer sequences of the ACTIN gene and other genes used for qRT-PCR are listed in Table 1.
Gene | GenBank accession no. | Primers |
ACTIN | AY212324 | F: 5′ GGTATTGTTAGCAACTGGGATG 3′ |
R: 5′ GATGAAAGAGGGCTGGAAGA 3′ | ||
OsCATA | AK099923 | F: 5′ AGGAGGCAGAAGGCGACGATACA 3′ |
R: 5′ TCTTCACATGCTTGGCTTCACGTT 3′ | ||
OsCATB | AK069446 | F: 5′ GGCTGTCGGGAAAAGTGTGTCATTG 3′′ |
R: 5′ TTTCAGGTTGAGACGTGAAGCCAGC 3 | ||
OsAPx1 | GQ848050 | F: 5′ TTCCTAGTGACAAAGCCCTGCTGAG 3′ |
R: 5′ ACCACGGGCAATGTACTAGCAGTAG 3′ | ||
OsAPx2 | AK068430 | F: 5′ TGGCTGCATTTCGTCCCTGTT 3′ |
R: 5′ GGGGGAAGCGGGAAGGAGTAG 3′ | ||
OsAPx3 | AK287946 | F: 5′ TGAAACAGAAGCATCCCAAGA 3′ |
R: 5′ GTGACTTCAACGGCAACAACT 3′ | ||
OsAPx4 | AK070842 | F: 5′ TCAGGAGCCTCTGAAGTTTGA 3′ |
R: 5′ GGCATAAAGATCCACATAGCG 3′ | ||
OsGLDH1 | AK102697 | F: 5′ TGGCTGCATTTCGTCCCTGTT 3′ |
R: 5′ GGGGGAAGCGGGAAGGAGTAG 3′ | ||
OsGLDH2 | AK241565 | F: 5′ TGGCTGTGCTTCGTCCCGTC 3′ |
R: 5′ GGGGGAAGCGGGAAGGAGTAG 3′ | ||
OsGME1 | AK104822 | F: 5′ TCGGAGAAGAACGGAACTGC 3′ |
R: 5′ CGGAGGCGATGATGTAATGC 3′ | ||
OsGME2 | AK102348 | F: 5′ AATCTCGCTCCCACGCCTCT 3′ |
R: 5′ GATGTAGTGGCCCTCGCTCT 3′ | ||
OsGA3ox1 | AB056519 | F: 5′ CGGACTCGGGCTTCTTCACCT 3′ |
R: 5′ CGAGGAAGTAGCCGAGCGAGAC3′ | ||
OsGA3ox2 | AK120915 | F: 5′ CGACGACTACCACCGCTTCTGC 3′ |
R: 5′ GGATCTTCCGCTCGGTCTCG 3′ | ||
OsGA20ox1 | AK099111 | F: 5′ CCGTGGAAGGAGACGCTGTC 3′ |
R: 5′ GCGGCTCATCTCGTGGCAGT 3′ | ||
OsGA20ox2 | AB077025 | F: 5′ CGCCGACTACTTCTCCAGCACC 3′ |
R: 5′ GCTGTCCGCGAAGAACTCCCT 3′ | ||
OsGA20ox3 | NM_001065522 | F: 5′ GAGGCGTACCGCTGCTGTGA 3′ |
R: 5′ GGCGGAGCAGTTGAACGACAT 3′ | ||
OsGA20ox4 | NM_001187498 | F: 5′ GAGGAGGTGACGAAGGCGATAA 3′ |
R: 5′ CACGGCGGGTAGTAGTTGCA 3′ |
Gene | GenBank accession no. | Primers |
ACTIN | AY212324 | F: 5′ GGTATTGTTAGCAACTGGGATG 3′ |
R: 5′ GATGAAAGAGGGCTGGAAGA 3′ | ||
OsCATA | AK099923 | F: 5′ AGGAGGCAGAAGGCGACGATACA 3′ |
R: 5′ TCTTCACATGCTTGGCTTCACGTT 3′ | ||
OsCATB | AK069446 | F: 5′ GGCTGTCGGGAAAAGTGTGTCATTG 3′′ |
R: 5′ TTTCAGGTTGAGACGTGAAGCCAGC 3 | ||
OsAPx1 | GQ848050 | F: 5′ TTCCTAGTGACAAAGCCCTGCTGAG 3′ |
R: 5′ ACCACGGGCAATGTACTAGCAGTAG 3′ | ||
OsAPx2 | AK068430 | F: 5′ TGGCTGCATTTCGTCCCTGTT 3′ |
R: 5′ GGGGGAAGCGGGAAGGAGTAG 3′ | ||
OsAPx3 | AK287946 | F: 5′ TGAAACAGAAGCATCCCAAGA 3′ |
R: 5′ GTGACTTCAACGGCAACAACT 3′ | ||
OsAPx4 | AK070842 | F: 5′ TCAGGAGCCTCTGAAGTTTGA 3′ |
R: 5′ GGCATAAAGATCCACATAGCG 3′ | ||
OsGLDH1 | AK102697 | F: 5′ TGGCTGCATTTCGTCCCTGTT 3′ |
R: 5′ GGGGGAAGCGGGAAGGAGTAG 3′ | ||
OsGLDH2 | AK241565 | F: 5′ TGGCTGTGCTTCGTCCCGTC 3′ |
R: 5′ GGGGGAAGCGGGAAGGAGTAG 3′ | ||
OsGME1 | AK104822 | F: 5′ TCGGAGAAGAACGGAACTGC 3′ |
R: 5′ CGGAGGCGATGATGTAATGC 3′ | ||
OsGME2 | AK102348 | F: 5′ AATCTCGCTCCCACGCCTCT 3′ |
R: 5′ GATGTAGTGGCCCTCGCTCT 3′ | ||
OsGA3ox1 | AB056519 | F: 5′ CGGACTCGGGCTTCTTCACCT 3′ |
R: 5′ CGAGGAAGTAGCCGAGCGAGAC3′ | ||
OsGA3ox2 | AK120915 | F: 5′ CGACGACTACCACCGCTTCTGC 3′ |
R: 5′ GGATCTTCCGCTCGGTCTCG 3′ | ||
OsGA20ox1 | AK099111 | F: 5′ CCGTGGAAGGAGACGCTGTC 3′ |
R: 5′ GCGGCTCATCTCGTGGCAGT 3′ | ||
OsGA20ox2 | AB077025 | F: 5′ CGCCGACTACTTCTCCAGCACC 3′ |
R: 5′ GCTGTCCGCGAAGAACTCCCT 3′ | ||
OsGA20ox3 | NM_001065522 | F: 5′ GAGGCGTACCGCTGCTGTGA 3′ |
R: 5′ GGCGGAGCAGTTGAACGACAT 3′ | ||
OsGA20ox4 | NM_001187498 | F: 5′ GAGGAGGTGACGAAGGCGATAA 3′ |
R: 5′ CACGGCGGGTAGTAGTTGCA 3′ |
Gene | GenBank accession no. | Primers |
ACTIN | AY212324 | F: 5′ GGTATTGTTAGCAACTGGGATG 3′ |
R: 5′ GATGAAAGAGGGCTGGAAGA 3′ | ||
OsCATA | AK099923 | F: 5′ AGGAGGCAGAAGGCGACGATACA 3′ |
R: 5′ TCTTCACATGCTTGGCTTCACGTT 3′ | ||
OsCATB | AK069446 | F: 5′ GGCTGTCGGGAAAAGTGTGTCATTG 3′′ |
R: 5′ TTTCAGGTTGAGACGTGAAGCCAGC 3 | ||
OsAPx1 | GQ848050 | F: 5′ TTCCTAGTGACAAAGCCCTGCTGAG 3′ |
R: 5′ ACCACGGGCAATGTACTAGCAGTAG 3′ | ||
OsAPx2 | AK068430 | F: 5′ TGGCTGCATTTCGTCCCTGTT 3′ |
R: 5′ GGGGGAAGCGGGAAGGAGTAG 3′ | ||
OsAPx3 | AK287946 | F: 5′ TGAAACAGAAGCATCCCAAGA 3′ |
R: 5′ GTGACTTCAACGGCAACAACT 3′ | ||
OsAPx4 | AK070842 | F: 5′ TCAGGAGCCTCTGAAGTTTGA 3′ |
R: 5′ GGCATAAAGATCCACATAGCG 3′ | ||
OsGLDH1 | AK102697 | F: 5′ TGGCTGCATTTCGTCCCTGTT 3′ |
R: 5′ GGGGGAAGCGGGAAGGAGTAG 3′ | ||
OsGLDH2 | AK241565 | F: 5′ TGGCTGTGCTTCGTCCCGTC 3′ |
R: 5′ GGGGGAAGCGGGAAGGAGTAG 3′ | ||
OsGME1 | AK104822 | F: 5′ TCGGAGAAGAACGGAACTGC 3′ |
R: 5′ CGGAGGCGATGATGTAATGC 3′ | ||
OsGME2 | AK102348 | F: 5′ AATCTCGCTCCCACGCCTCT 3′ |
R: 5′ GATGTAGTGGCCCTCGCTCT 3′ | ||
OsGA3ox1 | AB056519 | F: 5′ CGGACTCGGGCTTCTTCACCT 3′ |
R: 5′ CGAGGAAGTAGCCGAGCGAGAC3′ | ||
OsGA3ox2 | AK120915 | F: 5′ CGACGACTACCACCGCTTCTGC 3′ |
R: 5′ GGATCTTCCGCTCGGTCTCG 3′ | ||
OsGA20ox1 | AK099111 | F: 5′ CCGTGGAAGGAGACGCTGTC 3′ |
R: 5′ GCGGCTCATCTCGTGGCAGT 3′ | ||
OsGA20ox2 | AB077025 | F: 5′ CGCCGACTACTTCTCCAGCACC 3′ |
R: 5′ GCTGTCCGCGAAGAACTCCCT 3′ | ||
OsGA20ox3 | NM_001065522 | F: 5′ GAGGCGTACCGCTGCTGTGA 3′ |
R: 5′ GGCGGAGCAGTTGAACGACAT 3′ | ||
OsGA20ox4 | NM_001187498 | F: 5′ GAGGAGGTGACGAAGGCGATAA 3′ |
R: 5′ CACGGCGGGTAGTAGTTGCA 3′ |
Gene | GenBank accession no. | Primers |
ACTIN | AY212324 | F: 5′ GGTATTGTTAGCAACTGGGATG 3′ |
R: 5′ GATGAAAGAGGGCTGGAAGA 3′ | ||
OsCATA | AK099923 | F: 5′ AGGAGGCAGAAGGCGACGATACA 3′ |
R: 5′ TCTTCACATGCTTGGCTTCACGTT 3′ | ||
OsCATB | AK069446 | F: 5′ GGCTGTCGGGAAAAGTGTGTCATTG 3′′ |
R: 5′ TTTCAGGTTGAGACGTGAAGCCAGC 3 | ||
OsAPx1 | GQ848050 | F: 5′ TTCCTAGTGACAAAGCCCTGCTGAG 3′ |
R: 5′ ACCACGGGCAATGTACTAGCAGTAG 3′ | ||
OsAPx2 | AK068430 | F: 5′ TGGCTGCATTTCGTCCCTGTT 3′ |
R: 5′ GGGGGAAGCGGGAAGGAGTAG 3′ | ||
OsAPx3 | AK287946 | F: 5′ TGAAACAGAAGCATCCCAAGA 3′ |
R: 5′ GTGACTTCAACGGCAACAACT 3′ | ||
OsAPx4 | AK070842 | F: 5′ TCAGGAGCCTCTGAAGTTTGA 3′ |
R: 5′ GGCATAAAGATCCACATAGCG 3′ | ||
OsGLDH1 | AK102697 | F: 5′ TGGCTGCATTTCGTCCCTGTT 3′ |
R: 5′ GGGGGAAGCGGGAAGGAGTAG 3′ | ||
OsGLDH2 | AK241565 | F: 5′ TGGCTGTGCTTCGTCCCGTC 3′ |
R: 5′ GGGGGAAGCGGGAAGGAGTAG 3′ | ||
OsGME1 | AK104822 | F: 5′ TCGGAGAAGAACGGAACTGC 3′ |
R: 5′ CGGAGGCGATGATGTAATGC 3′ | ||
OsGME2 | AK102348 | F: 5′ AATCTCGCTCCCACGCCTCT 3′ |
R: 5′ GATGTAGTGGCCCTCGCTCT 3′ | ||
OsGA3ox1 | AB056519 | F: 5′ CGGACTCGGGCTTCTTCACCT 3′ |
R: 5′ CGAGGAAGTAGCCGAGCGAGAC3′ | ||
OsGA3ox2 | AK120915 | F: 5′ CGACGACTACCACCGCTTCTGC 3′ |
R: 5′ GGATCTTCCGCTCGGTCTCG 3′ | ||
OsGA20ox1 | AK099111 | F: 5′ CCGTGGAAGGAGACGCTGTC 3′ |
R: 5′ GCGGCTCATCTCGTGGCAGT 3′ | ||
OsGA20ox2 | AB077025 | F: 5′ CGCCGACTACTTCTCCAGCACC 3′ |
R: 5′ GCTGTCCGCGAAGAACTCCCT 3′ | ||
OsGA20ox3 | NM_001065522 | F: 5′ GAGGCGTACCGCTGCTGTGA 3′ |
R: 5′ GGCGGAGCAGTTGAACGACAT 3′ | ||
OsGA20ox4 | NM_001187498 | F: 5′ GAGGAGGTGACGAAGGCGATAA 3′ |
R: 5′ CACGGCGGGTAGTAGTTGCA 3′ |
ROS measurement
An Amplex Red Hydrogen Peroxide/Peroxidase Assay Kit (Invitrogen) was used to measure H2O2 production in imbibed seeds. Ten seeds were homogenized on ice with 1 ml of phosphate buffer (20 mM K2HPO4, pH 6.5). After centrifugation at 4 °C, 50 μl of the supernatant was incubated with 0.2 U ml−1 horseradish peroxidase and 10 μM Amplex Red reagent (10-acetyl-3, 7-dihydrophenoxazine) at room temperature for 30 min under dark conditions. The fluorescence was quantified using an Infinite® 200 PRO microplate reader (Tecan, Männedorf, Switzerland) (excitation at 560 nm and emission at 590 nm) (Xing et al., 2008).
O2− production was measured as described by Able et al. (1998) by monitoring the reduction of 2,3-bis (2-methoxy-4-nitro-5-sulphophenyl)-5-[(phenylamino) carbonyl]-2Htetrazolium hydroxide (XTT) in the presence of O2−, with some modifications. Ten seeds were homogenized with 5 ml of 50 mM TRIS-HCl buffer (pH 7.5) and centrifuged at 5000 g for 10 min at 4 °C. The reaction mixture (1 ml) contained 50 mM TRIS-HCl buffer (pH 7.5), 50 mg of 5000 g supernatant proteins, and 0.5 mM XTT. The reduction of XTT was determined at 470 nm for 5 min. Corrections were made for the background absorbance in the presence of 50 U of superoxidase dismutase (SOD). The O2− production rate was calculated using an extinction coefficient of 2.163104 M−1cm−1.
Malondialdehyde measurements
Lipid peroxidation was evaluated by measuring the malondialdehyde (MDA) content from 0.5 g fresh weight (FW) of imbibed seeds, according to the method of Heath and Packer (1968) with slight modification. The results are expressed as μmol g−1 FW of seeds and correspond to means of measurements carried out with five extracts ±SD.
In situ localization of superoxide anion
Whole seeds were hand-cut into two embryo parts containing half-seeds using a very fine knife and were then incubated in 6 mM nitroblue tetrazolium (NBT) in 10 mM TRIS-HCl buffer (pH 7.4) at room temperature for 5 min. Superoxide anion was visualized as deposits of dark-blue insoluble formazan compounds (Beyer and Fridovich, 1987).
Dye loading and laser scanning confocal microscopy
The imbibed seeds were hand-cut into thin sections using a very sharp knife. Embryo-containing sections were washed gently in warm Hanks’ balanced salt solution (HBSS) buffer with Ca2+ and Mg2+ immediately, then a sufficient amount of 25 μM 2′,7′-dichlorofluorescin diacetate (H2DCF-DA) (Invitrogen) working solution was added for 30 min at 37 °C in the dark, and then washed briefly three times before further experiments. The fluorescence in seed sections was measured using a laser scanning confocal microscope (LSCM; Zeiss 510) with the following settings: excitation=488 nm, emission=535 nm, frame 512×512. To enable a comparison of changes in the signal intensity, confocal images were taken under identical exposure conditions for all samples. The experiments were repeated at least three times with 10–15 seed sections in each treatment, and the selected confocal image represented the same results from ∼15 time experiments.
Enzyme assays
Frozen seeds (0.3 g) were homogenized on ice with 1 ml of 50 mM potassium phosphate buffer (pH 7.0) containing 1 mM EDTA and 1% polyvinylpyrrolidone (PVP). The homogenate was centrifuged at 15 000 g for 20 min at 4 °C and the supernatant was used for the following enzyme assays. Protein content was determined according to the method of Bradford (1976) with bovine serum albumin (BSA) as standard.
Total SOD (EC 1.15.1.1) activity was assayed by monitoring the inhibition of photochemical reduction of NBT according to the method of Giannopolitis and Ries (1977). The 2.5 ml reaction mixture contained 50 mM potassium phosphate buffer (pH 7.8), 13 mM methionine, 75 mM NBT, 2 mM riboflavin, 0.1 mM EDTA, and 20 μl of enzyme extract. The reaction mixtures were illuminated for 15 min at a light intensity of 5000 lux. One unit of SOD activity was defined as the amount of enzyme required to cause 50% inhibition of the reduction of NBT as monitored at 560 nm.
Catalase (CAT; EC 1.11.1.6) activity was determined by following the consumption of H2O2 (extinction coefficient 39.4 mM−1 cm−1) at 240 nm for 1 min (Aebi, 1984). The reaction mixture contained 50 mM potassium phosphate buffer (pH 7.0), 10 mM H2O2, and 20 μl of enzyme extract in a 3 ml volume.
Glutathione reductase (GR; EC 1.6.4.2) activity was determined by following the oxidation of NADPH at 340 nm (extinction coefficient 6.2 mM−1 cm−1) for 1 min in 1 ml of an assay mixture containing 50 mM potassium phosphate buffer (pH 7.8), 2 mM Na2EDTA, 0.15 mM NADPH, 0.5 mM oxidized glutathione, and 100 μl of enzyme extract. The reaction was initiated by adding NADPH. Corrections were made for the background absorbance at 340 nm, without NADPH (Schaedle and Bassham, 1977).
Ascorbate peroxidase (APx; EC 1.11.1.11) activity was determined by following the decrease in A290 (extinction coefficient 2.8 mM−1 cm−1) for 30 s in 1 ml of a reaction mixture containing 50 mM potassium phosphate buffer (pH 7.0), 0.5 mM ASC, 0.1 mM H2O2, and 20 μl of enzyme extract. The reaction was started by enzyme extract. Correction was done for the low, non-enzymatic oxidation of ASC by H2O2 (Nakano and Asada, 1981).
Ascorbic acid measurements
ASC content was measured from 0.5 g FW of imbibed seeds, according to the method of Kampfenkel et al. (1995) with slight modification. The results are expressed as μmol g−1 FW of seeds and correspond to means of measurements carried out with five extracts ±SD.
Amylase activity assay
Frozen seeds (0.5 g) were homogenized on ice with 1.5 ml of 100 mM potassium phosphate buffer with protease inhibitor [1 mM EDTA, 10% (v/v) glycerol, 1% (v/v) Triton X-100, 7 mM β-mercaptoethanol, 100 mM NaF, 1 mM NaVO3, 1 mM Na3VO4, 10 mM Na4P2O7, 10 mM N-ethylmaleimide]. A 100 μg aliquot of total soluble proteins was assayed in the assay buffer (50 mM Na-acetate, pH 5.2, 10 mM CaCl2, 2% boiled soluble potato starch) and incubated at 37 °C for 1 h. Then 100 μl was taken from the assay mixture and treated with 100 μl of reaction termination buffer (0.1 N NaOH) and 100 μl of 3, 5-dinitrosalycilic acid (DNS) solution (40 mM DNS, 400 mM NaOH, 1 M K-Na tartrate) for 5 min at 100 °C. After dilution with 700 μl of distilled water, the absorbance at 540 nm (A540) was measured, and the reducing power was evaluated with a standard curve obtained with glucose (0–20 μmol). One enzymatic unit is defined as the amount of enzyme releasing 1 mmol of glucose in 1 min.
Results
ABA inhibits germination in rice seed
The process of water uptake by a mature dry seed is triphasic. The initial influx (Phase I, imbibition) is a result of the very low water potential of the dry matrices of the seed (cell walls and storage components). After the rapid hydration, water uptake reaches a plateau (Phase II). A further increase in water uptake occurs only after germination is completed, as the embryo grows into a seedling (Phase III) (Bewley, 1997). In this study, the dehulled rice grain was used. Figure 1 shows the kinetic water uptake of the caryopses. Though germination of caryopses is faster than that of normal seeds, they still follow three phases of water uptake. Phase I covered 6 h from the onset of imbibition, followed by a plateau phase (Phase II) to 24 h, and then the start of germination (Phase III). The time point of 24 h is critical because it links Phase II and Phase III and determines a successful germination.
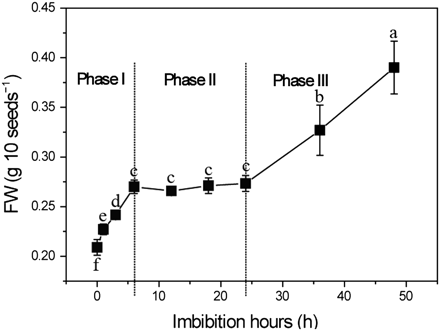
Time course of germination of dehulled rice seeds. Seeds were directly sown on sterile filter paper in 5 cm Petri dishes and placed in a growth chamber in continuous darkness at 28 °C to facilitate germination. Seeds were weighed at various time points. Values are means ±SD (n=5). Means denoted by the same letter did not differ significantly at P < 0.05 according to Duncan’s multiple range test.
During the rice seed germination, ABA catabolism plays a key role by decreasing the ABA level in imbibed seeds (Zhu et al., 2009). Here an ABA catabolic inhibitor, diniconazole, which acts as a potent competitive inhibitor of ABA 8'-hydroxylase and is an effective ABA catabolic inhibitor (Kitahata et al., 2005), was used to demonstrate the suppressive effect of ABA on germination of rice caryopsis. As shown in Fig. 2a, seed germination is effectively suppressed by a low concentration of ABA (0.5 μM) and diniconazole. A high concentration (5 μM) of ABA totally arrested the seed germination. To reveal further ABA actions on seed germination, the ABA levels of imbibed seeds were determined using the RIA method. ABA contents decrease significantly within 6 h after the onset of imbibition. However, the ABA level in diniconazole-treated seeds is higher than that in control seeds, which results in inhibition of germination (Fig. 2b). These results indicate that a relatively high ABA level in Phase II is essential to suppress the germination of rice caryopsis.
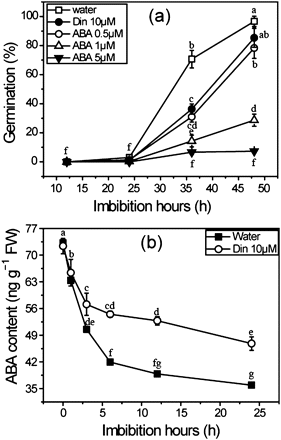
Effects of ABA and diniconazole on seed germination (a) and ABA contents (b). Rice seeds were imbibed at 28 °C in the presence of water, diniconazole, and ABA solutions. Seed samples were collected at different time intervals and stored at –80 °C for ABA content determination. ABA was detected by RIA as described in the Materials and methods. Values are means ±SD (n=5). Means denoted by the same letter did not differ significantly at P < 0.05 according to Duncan’s multiple range test.
ABA suppresses rather than enhances the production of ROS during imbibition
Since ABA has been reported to induce ROS production and lead to oxidative stress in leaves (Jiang and Zhang, 2001, 2002; Hu et al., 2006), it is interesting to know whether ABA can also induce an oxidative stress that may result in any inhibition of germination. To answer this question, the MDA content, which is a well known index for lipid oxidation and the oxidative state in plant, was detected in imbibing seeds. The results show that the MDA content increased during seed imbibition. However, ABA treatment (5 μM) did not perturb the MDA level when compared with control (Fig. 3a), whereas treatment with copper significantly increased the MDA content in germinating seeds (Fig. 3b). These results indicate that ROS production is not increased by ABA treatment. Hence the contents of superoxide anion radical and hydrogen peroxide (H2O2) were measured directly. As shown in Fig. 3c and d, the ROS contents were increased during imbibition, and the variation trends were consistent with the MDA content (Fig. 3a). However, if compared with seeds imbibed in water, ABA treatment markedly suppressed the production of O2− and H2O2 after the emergence of the radicle. Furthermore, the inhibitory effects of ABA on the two ROS were concentration dependent (Fig. 3e, f). Results from these experiments suggest that ABA can suppress rather than enhance ROS production during seed imbibition.
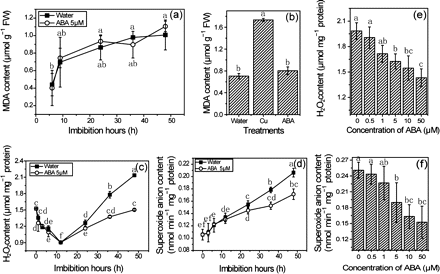
Effects of ABA treatment on MDA content (a, b), H2O2 content (c, e), and superoxide anion (d, f). Seeds were sown on filter paper with ABA and Cu solutions for imbibition. Seed samples were collected at various times and used for measurements of MDA, H2O2, and superoxide anion as rapidly as possible. For concentration experiments, seeds imbibed for 36 h were used for the measurements. Error bars show ±SD (n=5). Means denoted by the same letter did not differ significantly at P < 0.05 according to Duncan’s multiple range test.
A reduced ROS content is involved in the inhibition of seed germination by ABA
In recent years many researches have proved that ROS play a key role during seed germination (Oracz et al., 2007; Müller et al., 2009; Liu et al., 2010). In order to further explain the role of ROS in germination of rice seeds, two ROS scavengers, DPI and Tiron, were used. DPI can effectively inhibit seed germination at low concentrations, while Tiron can only partly delay germination in rice seeds (Fig. 4a). A further result shows that the inhibitory effects on seed germination of the scavengers are well related to seed ROS levels. For example, Tiron only decreased H2O2 and O2− by 15% and 10%, respectively. However, the contents of H2O2 and O2− were significantly suppressed by DPI, by 30% and 24%, respectively (Fig. 4b). In situ fluorescent images for H2O2 with H2DCF-DA and localization of O2− with NBT are shown in Fig. 4c and d. These results show that H2O2 and O2− are both located mainly in the embryo and partly in the aleurone layer. The fluorescent intensity and NBT staining were non-significantly reduced by Tiron when compared with control (water). However, they were obviously impaired by the treatments with DPI and ABA (Fig. 4c, d), suggesting that the reduction of ROS production may be the crucial factor that mediates the inhibition of seed germination by ABA.
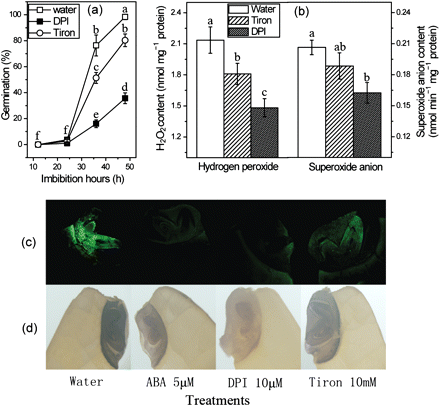
Changes in germination rate and ROS content in imbibing seeds under different treatments. (a) Effects of the ROS scavengers, DPI and Tiron, on seed germination. (b) Changes of H2O2 and O2− contents in imbibing seeds treated with DPI and Tiron. (c) H2O2 fluorescent images of seeds imbibed for 24 h under different treatments. The seeds which were cut straight across were loaded with H2DCF-DA for 30 min and then cut into very thin sections. The embryo-containing section was detected by confocal laser scanning microscopy. (d) Localization of O2− by NBT staining. The seeds which were cut straight across were stained by NBT solution for 5 min and viewed by light microscopy. Error bars show ±SD (n=5). Means denoted by the same letter did not differ significantly at P < 0.05 according to Duncan’s multiple range test.
The reduced ROS content results in decreases APx activity and ASC content
Because ROS production is suppressed by ABA, it is interesting to know whether the antioxidant system is also affected by ABA. As shown in Fig. 5a–d, the activities of CAT, APx, and GR are increased during seed imbibition, and have a similar trend to ROS production. In contrast, the activity of SOD is the highest in dry seed and shows a decreasing trend during imbibition. The activities of SOD and GR were not affected when seed were treated with ABA (Fig. 5a, b); CAT activity was reduced by 17%, whereas APx activity was more significantly suppressed, up to 45%, by exogenous ABA (Fig. 5c, d). In order to investigate how CAT and APx were affected by ABA treatment, their gene expression during imbibition was analysed by qRT-PCR. The results show that OsCATA and OsAPx1 are the most abundant genes in seeds germinated for 36 h and have a similar pattern of change to their enzyme activities, indicating that the two genes are the key genes responsible for their enzyme activities, respectively (Fig. 6a, b). As expected, when ROS production was reduced by ABA or DPI, the expression of OsCATA and OsAPx1 genes was suppressed in imbibing seeds (Fig. 6c, d). As a fine control of H2O2, APx with a high affinity for H2O2 was significantly reduced by reduced ROS production in seeds treated with ABA and DPI, indicating that the APx activity is dependent on ROS production during seed germination.
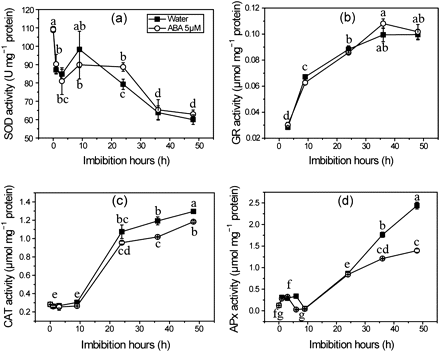
Effects of ABA on SOD activity (a), GR activity (b), CAT activity (c), and APx activity (d) during seed imbibition. Seed samples were collected at different times and stored at –80 °C for these antioxidant enzymes assays. Values are means ±SD (n=5). Means denoted by the same letter did not differ significantly at P < 0.05 according to Duncan’s multiple range test.
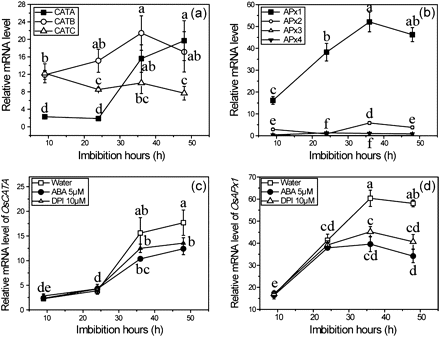
Expression analyses of CAT and APx gene families. (a, b) Expression of the CAT gene family and the APx gene family during seed germination. (c, d) Changes in expression of OsCATA and OsAPx1 genes during seed germination under treatment with ABA and DPI. Seeds samples were collected at different times and stored at –80 °C for expression analysis by qRT-PCR. Error bars show ±SD (n=3). Means denoted by the same letter did not differ significantly at P < 0.05 according to Duncan’s multiple range test.
Being a powerful antioxidant, ASC is expected to play a role in seed germination (De Tullio and Arrigoni, 2003). In this study, the ASC content did not increase until 24 h after the onset of imbibition (Fig. 7a). Exogenous application of ABA decreased ASC contents up to 40% in imbibing seeds (Fig. 7a) and, moreover, the inhibition of ASC accumulation by ABA was concentration dependent, similar to APx activity (Fig. 7b). OsGLDH and OsGME are key genes in the ASC biosynthesis pathway and were also inhibited by ABA and DPI (Fig. 7c, d). The effects of ROS on ASC production were clearly shown by manipulating the ROS content using ABA, diniconazole, DPI, and Tiron, indicating the relationship between ASC production and ROS (Fig. 7e). These results suggest that ABA suppresses the ASC content through inhibiting its biosynthesis, which is mediated by a reduced ROS production.
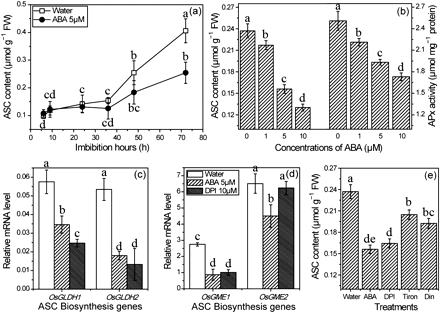
Changes of expression of ASC biosynthesis genes and ASC contents in seeds under treatment with ABA, DPI, Tiron, and diniconazole. (a) ASC content during imbibition under ABA treatment. (b) Effect of ABA at different concentrations on ASC content and APx activity. (c, d) Changes in xpression of ASC biosynthesis gene in seeds treated with ABA and DPI for 36 h. (e) Change of ASC content in seeds treated with ABA, DPI, Tiron, and diniconazole for 36 h. Means are values ±SD (n=5). Means denoted by the same letter did not differ significantly at P < 0.05 according to Duncan’s multiple range test.
Application of ASC releases the inhibitory effect of ABA on seed germination
Many investigations have shown that ASC is induced during seed germination (De Gara et al., 1991, 2000; De Tullio and Arrigoni, 2003). However, its functions in this process have not been investigated. In the present study, it was found that an ASC concentration higher than 4 mM would inhibit seed germination (Fig. 8a). Consistent with this, the ASC content in seeds treated with >4 mM ASC showed a significant increase, which was not seen with those treated with concentrations <4 mM when compared with the control (Fig. 8b). A similar result has also been reported in wheat (Takemura et al., 2010). These results indicate that the ASC level in imbibing seeds in normal conditions is sufficient and under fine control. To find out whether exogenous ASC can compensate for the inhibitory effect of ABA on seed germination, seeds were treated with ABA and various concentrations of ASC, together. As shown in Fig. 8c, application of ASC at 1 mM and 2 mM can only rescue a small part of the seed, whereas ASC concentrations >4 mM have aggravated the suppression of seed germination. This result indicates that ABA-inhibited seed germination is partially mediated by ASC.
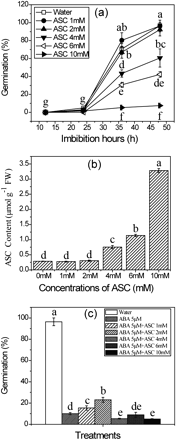
Effects of ASC on germination of rice seeds in water and ABA. (a) Inhibition of seed germination by different concentrations of ASC. (b) Endogenous ASC level in seeds treated with different concentrations of ASC. (c) Effect of ASC on ABA during seed germination. Seeds were sown on filter paper with solutions of ABA at 5 μM plus different concentrations of ASC for imbibition. Seeds imbibed for 36 h were used for the ASC measurement. Error bars here show ±SD (n=5). Means denoted by the same letter did not differ significantly at P < 0.05 according to Duncan’s multiple range test.
ASC is involved in the ABA-induced inhibition of seed germination by suppressing GA production
The antagonism between ABA and GA plays a key role in the release of seed dormancy. In the present study, the expression of key GA biosynthesis genes, OsGA3ox and OsGA20ox, was inhibited by ABA and DPI treatments (Fig. 9a). Amylase, which is indicator of GA accumulation during seed germination, is significantly induced by GA. In this study, both gene expression and enzyme activity of amylase were effectively inhibited by ABA and DPI (Fig. 9b, c), indicating a reduced GA accumulation in seeds treated with ABA and DPI. Similar results found with ABA and DPI treatments also indicate that reducing ROS production can lead to a suppression of GA biosynthesis.
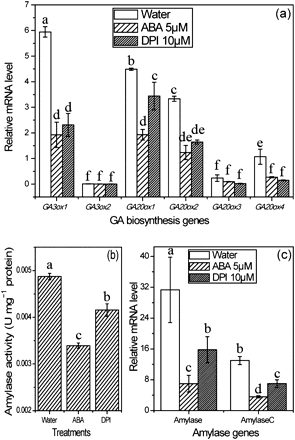
Effects of ABA and DPI treatments on expression of GA biosynthesis genes (a), amylase gene expression (c), and amylase activity (b). Seeds were sampled after imbibition for 36 h in water, 5 μM ABA, and 10 μM DPI solutions. Samples were stored at –80 °C for further experiments. Error bars here show ±SD (n=5). Means denoted by the same letter did not differ significantly at P < 0.05 according to Duncan’s multiple range test.
Since ASC and GA biosynthesis are inhibited by ABA, is there any interaction between ASC and GA production during seed germination? To answer this question, an inhibitor, lycorine, was used since this alkaloid has been proved to be a strong inhibitor of (l-galactono-γ-lactone) GaL dehydrogenase (EC 1.3.2.3), the last enzyme of the ASC biosynthetic pathway, both in vivo (Arrigoni, 1975; De Tullio et al., 1998; Onofri et al., 2003) and in vitro (Arrigoni et al., 1997). As shown in Fig. 10a, seed germination was significantly inhibited by concentrations ≥50 μM of lycorine, but not by lower concentrations. After analysing the ASC content in imbibing seeds it was found that lycorine only at concentration of >50 μM could reduce the ASC content in seeds (Fig. 10a, b). Exogenous application of lycorine reduced the expression of key genes in the GA biosynthesis pathway in seeds treated with lycorine, which is similar to treatment with ABA (Fig. 10c). Meanwhile, both of the treatments had the same effect on ASC biosynthesis in seed. In addition, the amylase mRNA level and its activity were inhibited by lycorine in a concentration-dependent manner in rice seeds (Figs. 10d, e). These results indicate that the ABA-reduced ROS production leads to the suppression of ASC biosynthesis, which subsequently inhibits the GA production.
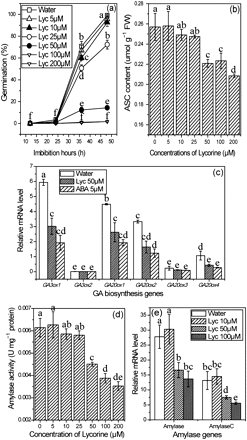
Effect of lycorine at different concentrations on seed germination (a), ASC content (b), amylase activity (d), and amylase gene expression (e). Inhibition of expression of GA biosynthesis genes by ABA and lycorine (b). Seeds for ASC content, RNA extraction, and amylase activity were sampled after imbibition in lycorine solution for 36 h and stored at –80 °C for various experiments. Values are means ± SD (n=5). Means denoted by the same letter did not differ significantly at P < 0.05 according to Duncan’s multiple range test.
Discussion
Seed germination is a complex process and is controlled by interactions of many internal factors (Kucera et al., 2005). Studies of ABA catabolism and GA biosynthesis in seed biology have proved that both of them play a prominent role in seed germination (Ogawa et al., 2003; Saito et al., 2004; Okamato et al., 2006; Seo et al., 2006). Although much progress has been made, the counteractive relationship between ABA and GA is still not clear. In this study, a possible signalling pathway for an ABA-suppressed GA accumulation during seed germination is provided. This result also suggests that ABA inhibits seed germination in a multipathway manner.
ABA stays at a relatively high level in dry seeds and decreases rapidly after the onset of imbibition (Zhu et al., 2009). As shown in Fig. 2, ABA can sensitively inhibit seed germination. Suppression of ABA catabolism by diniconazole had a similar effect to that of a low concentration of ABA (0.5 μM). By analysing the ABA content in the imbibing seeds, it was found that the ABA level in seeds treated with diniconazole was much higher than in those treated with water (Fig. 2b). This higher ABA content thus resulted in the failure of germination.
ROS production is increased by ABA in plants under stress conditions, which plays a pivotal role in the ABA signalling pathway (Jiang and Zhang, 2001; Zhang et al., 2001; Gechev et al., 2006). Studying the function of ROS and their relevance to ABA in seed biology is therefore an interesting subject. Some results indicate that ROS is essential for seed germination (Schopfer et al., 2001; Bailly, 2004). However, it was found that the regulatory role of ABA on ROS production is different from that in non-seed tissues. NADPH-oxidases are key enzymes for ROS production and their activities are suppressed by ABA in seed germination. Inhibition of these enzymes by mutation or a pharmacological approach will reduce the ROS accumulation, which leads to inhibition of seed dormancy alleviation and germination (Müller et al., 2009; Ishibashi et al., 2010). In the present study, the contents of H2O2 and O2− are reduced by ABA (Fig. 3c, d) and the negative regulation is concentration dependent (Fig. 3e, f). A pharmacological approach using DPI and Tiron further reveals that the germination rate is highly positively related to ROS production during imbibition in rice seeds (Figs. 4a, b). Fluorescence imaging and localization by NBT staining experiments show that the ROS concentrate in the embryo region as well as the aleurone layer during imbibition in rice seeds. Both the fluorescent and staining intensities are impaired by treatments with ABA, diniconazole, DPI, and Tiron (Fig. 4c, d). Previous research has proved that ROS can act as a key component in cell wall loosening, testa and endosperm weakening, as well as protein oxidation in the aleurone layer, which is essential for radicle protrusion in seed germination (Schopfer et al., 2001; Schweikert et al., 2000, 2002; Oracz et al., 2007). In addition, ROS are also capable of inducing gene expression for GA biosynthesis in Arabidopsis (Liu et al., 2010). Together with previous studies, the present results indicate that ROS may have multiple functions and their enhanced levels are essential for dormancy breaking and seed germination in rice.
Due to the toxic properties of ROS, plants have developed a very complicated antioxidant system to control their production. Furthermore, the regulation of antioxidant activity is not only a strategy in plant adaptation to environmental stresses but also a mechanism in modulating cellular signal transduction (Veal et al., 2007). According to the present results, enzyme activities of GR, CAT, and APx, but not SOD, are not detected in dry seeds, but they all are increased in a few hours after imbibition (Fig. 5a–d). The activities of CAT and APx are suppressed differently by application of ABA (Fig. 5c, d). Because the affinity of APx for H2O2 (substrate) is much higher than that of CAT, APx activity is more easily affected by ROS production than CAT activity and acts as a fine control for ROS (Van Breusegem et al., 2001). In agreement with this theory, the effects of reducing ROS production by ABA on both enzyme activity and gene expression of APx were found to be far more significant than that of CAT in this study (Figs 5, 6).
Since its discovery in the late 1920s (Davies et al., 1991), perhaps no other chemical has ever been as celebrated as ASC. ASC can react directly with ROS or act as a substrate for APx to scavenge ROS. Together with its regenerative property, ASC is perhaps the most powerful antioxidant (Noctor and Foyer, 1998). In the present study, ASC was not detected in dry seed but increased rapidly by imbibition. Similar to APx activity, ASC production is also suppressed by ABA application in a concentration-dependent manner, revealing the function of ASC and the inhibitory effect of ABA on ASC production during seed germination (Fig. 7a, b). The expression of ASC biosynthesis genes, OsGLDH and OsGME, was inhibited by ABA and DPI treatments (Fig. 7c, d), suggesting that suppression of ASC content results from the inhibition of ASC biosynthesis. Furthermore, treatments that can reduce ROS production also repressed ASC content (Fig. 7e). These results suggest that ASC production is inhibited by ABA, as a consequence of a reduced ROS content during seed germination.
It is possible that suppressing the ASC content during seed germination will lead to other consequences, because ASC is much more than just an antioxidant (Arrigoni and De Tullio, 2002). As a proton donor, ASC also acts as substrate for many 2-ODDs, which include dioxygenases involved in plant hormone biosynthesis such as GA. The function of ASC indicates its close relationship with plant hormone biosynthesis during seed germination. A high dose of ASC can suppress seed germination (Fig. 8a). As a powerful antioxidant, too much ASC would lead to the elimination of ROS in seed, which results in failure of germination (Takemura et al., 2010). ASC is absorbed in seed by both active transport and simple diffusion (Rumsey et al., 1997; Savini et al., 2007), which explains why ASC concentrations of 1 mM and 2 mM do not affect the endogenous ASC level in seeds (Fig. 8b). However, these low doses of ASC slightly increased the germination rate in seed treated with ABA (Fig. 8c), suggesting the involvement of ASC in the ABA signalling pathway. As it is already known that the ASC content did not increase until 24 h after the onset of imbibition (Fig. 7a), which occurs in phase III during germination (Fig. 1), this indicates the involvement of ASC in the later stage of germination and the reason why ASC can only rescue a small part of seeds treated with ABA. By treating seeds with ABA and DPI, it was found that expression of key genes in GA biosynthesis was suppressed. The activity of amylase was also inhibited as a result of a lower GA level (Fig. 9). It has been reported that expression of GA biosynthesis genes is inhibited by DPI but increased by H2O2 in seeds of Arabidopsis (Liu et al., 2010). The present results prove further that ROS play a key role in mediating GA biosynthesis. The results of the pharmacological experiment also show that when the ASC content was effectively suppressed by lycorine, an inhibitor of the ASC biosynthesis pathway, seed germination was reduced (Fig. 10a, b), together with a reduced expression of GA biosynthesis genes (Fig. 10c). This is very similar to the effects of ABA. In addition, lycorine can also concentration-dependently suppress the expression of amylase genes and amylase activity (Fig. 10d, e). These results suggest that ASC plays a key role in mediating the accumulation of GA and amylase activities by suppressing their gene expression. Thus, it is concluded that ABA inhibits GA accumulation through reducing the ASC level, the substrate of GA biosynthesis enzymes.
In summary, it is concluded that ABA and ROS regulate seed germination in multiple dimensions. ABA reduces the ROS levels in key tissues of seeds such as embryos and aleurone layers. Reduced ROS will also inhibit ASC production. Both ROS and ASC are required at enhanced levels to promote many biochemical and physiological processes of seed germination. GA biosynthesis is also suppressed by ABA, possibly through a reduced level of ASC, which acts as a substrate in the biosynthesis. GA is known to regulate directly the expression of many genes during seed germination. Whether ABA can directly influence such regulation requires further study.
Abbreviations
- ABA
abscisic acid
- APx
ascorbate peroxidase
- ASC
ascorbic acid
- CAT
catalase
- GA
gibberellin
- MDA
malondialdehyde
- ROS
reactive oxygen species
This work was supported by Hong Kong Research Grants Council (HKBU 262809) and Hong Kong Baptist University Strategic Development Fund (SDF 090910P03).
Comments