-
PDF
- Split View
-
Views
-
Cite
Cite
Christine H. Foyer, Jenny Neukermans, Guillaume Queval, Graham Noctor, Jeremy Harbinson, Photosynthetic control of electron transport and the regulation of gene expression, Journal of Experimental Botany, Volume 63, Issue 4, February 2012, Pages 1637–1661, https://doi.org/10.1093/jxb/ers013
- Share Icon Share
Abstract
The term ‘photosynthetic control’ describes the short- and long-term mechanisms that regulate reactions in the photosynthetic electron transport (PET) chain so that the rate of production of ATP and NADPH is coordinated with the rate of their utilization in metabolism. At low irradiances these mechanisms serve to optimize light use efficiency, while at high irradiances they operate to dissipate excess excitation energy as heat. Similarly, the production of ATP and NADPH in ratios tailored to meet demand is finely tuned by a sophisticated series of controls that prevents the accumulation of high NAD(P)H/NAD(P) ratios and ATP/ADP ratios that would lead to potentially harmful over-reduction and inactivation of PET chain components. In recent years, photosynthetic control has also been extrapolated to the regulation of gene expression because mechanisms that are identical or similar to those that serve to regulate electron flow through the PET chain also coordinate the regulated expression of genes encoding photosynthetic proteins. This requires coordinated gene expression in the chloroplasts, mitochondria, and nuclei, involving complex networks of forward and retrograde signalling pathways. Photosynthetic control operates to control photosynthetic gene expression in response to environmental and metabolic changes. Mining literature data on transcriptome profiles of C3 and C4 leaves from plants grown under high atmospheric carbon dioxide (CO2) levels compared with those grown with ambient CO2 reveals that the transition to higher photorespiratory conditions in C3 plants enhances the expression of genes associated with cyclic electron flow pathways in Arabidopsis thaliana, consistent with the higher ATP requirement (relative to NADPH) of photorespiration.
Introduction
Photosynthetic control of electron transport is a fundamental concept in the regulation of photosynthesis (Foyer et al., 1990). It stems from the observation that the proton motive force (pmf) exerts a feedback control over electron flux, forming a regulatory circuit between ATP synthesis and electron transport in energy-transducing membranes. In this way, electron transport rates can be restrained when ATP is abundant and the pmf is high. The pmf is comprised of the transthylakoid voltage and the pH gradient (ΔpH). As electrons pass through the photosynthetic electron transport (PET) chain the thylakoid lumen becomes positively charged and acidic relative to the stroma. Lumen acidification controls plastoquinol (PQH2) oxidation by the cytochrome b6/f (cytb6f) complex. The plastoquinone (PQ)/PQH2 pool is an integrating regulatory redox hub that controls both the efficiency of electron transport and the activation of gene expression. PQH2 oxidation also controls post-translational regulation of photosynthetic proteins by phosphorylation and also the rate of transcription of genes encoding the reaction centre apoproteins of photosystem I (PSI) and PSII. In this way, the stoichiometries of the photosystems are balanced to optimize the relative efficiency of operation of each photosystem. Gene expression within the chloroplasts and nucleus is controlled through the activation of a network of kinases that are involved in chloroplast to nucleus retrograde signalling pathways. The fundamental concepts and hypotheses concerning the photosynthetic control hub are considered here in detail, with particular emphasis on the central role of reduction–oxidation (redox) metabolism, homeostasis, and signalling in the regulation of gene expression in response to changes in atmospheric CO2 levels.
Concepts of energy production and utilization
The basic function of the light-harvesting and electron transport systems is to convert the free energy of absorbed light as efficiently as possible into forms [ATP, reduced ferredoxin (Fd), and NADPH] that can be used to drive metabolism in the chloroplast and cytosol. When the supply of free energy from light is in excess of the needs of metabolism, a secondary function of light harvesting and electron transport emerges, namely the safe dissipation of the excess absorbed free energy. A third function arises from the fluctuating ratio of demand for reductant and ATP. The photosynthetic apparatus must be able to adjust the relative rates of synthesis of ATP and reductant in order to prevent limitations on the operation of photosynthetic energy transduction arising from an imbalance in the availability of the two forms of assimilatory power. The integrated operation of light-limited photosynthesis, energy dissipation, and the balance between the synthesis of ATP and reductant lies at the heart of photosynthetic regulation and its responses to the environment.
Light absorption and charge separation by PSI and PSII
An essential property of any light-driven system is its ability to absorb light. In the case of photosynthesis in higher plants, light absorption results from the presence in leaves of significant quantities of chlorophylls (chls) a and b and carotenoids, of which β-carotene, lutein, violaxanthin, and neoxanthin are the most common (Kusaba et al., 2009). The photosynthetically active wavelengths within the spectrum are normally in the range 400–700 nm, though wavelengths shorter or longer than this can still have some photosynthetic activity. For a typical leaf, the absorption of photosynthetically active radiation (PAR) is ∼85% (Baker et al., 2007), implying that leaves are good light traps. At the chl and carotenoid concentrations normally found in leaves, the absorptivity for PAR is relatively insensitive to changes in pigment level (Evans, 1993; de Groot et al., 2003), though at wavelengths of ∼560 nm and >700 nm, where absorption is low, the effect of increased pigment levels is more noticeable (Björkman and Demmig, 1987). It is important to note that not only photosynthetic pigments absorb PAR; flavonoids and other phenolic substances are present in most leaves to varying degrees and absorb wavelengths <560 nm. This partly accounts for the lower quantum efficiencies for CO2 fixation measured under light-limiting conditions at wavelengths <560 nm (Inada, 1976). The relatively inefficient energy transfer from carotenoids to chls (only 70–90%) also contributes to the lowered light use efficiency of photosynthesis at wavelengths <560 nm (Croce et al., 2001; de Weerd et al., 2003; van Oort et al., 2008).
When a photosynthetic pigment (chl or carotenoid) absorbs a quantum of PAR, an excited state is formed. These excited states are ultimately transferred to chl a to form the first singlet (S1) excited state (1chl*) and it is in this form that the excitation energy migrates, by resonance transfer from pigment to pigment, to the reaction centres. 1Chl* is inherently unstable and, in PSII, for example, has a maximum lifetime of only 3 ns so excited states cannot be accumulated by the photosystems. An important property of the reaction centres of both photosystems is their ability to use the fugitive, high-energy 1chl* to drive an otherwise endothermic charge separation reaction, a stable chemical transformation, with a high efficiency, implying very rapid chemistry (Lavergne and Joliot, 2000). The efficiency by which this charge separation reaction is driven by PAR can be assessed in different ways. Firstly, it can be determined as a quantum efficiency, which is a measure of what proportion of quanta, on either an incident or an absorbed light basis, produce a specific change. The quantum efficiencies of photosynthesis are frequently assessed on a relative basis (Baker et al., 2007). They can also be measured in terms of the proportion of the incident or absorbed light energy that is conserved in free energy of the products of photosynthesis, but this parameter is less easy to measure (Hill and Rich, 1983; Nitsch et al., 1988).
The maximum (i.e. dark-adapted) quantum yield for charge separation in PSII (ΦPSII) obtained from chl fluorescence measurements is ∼0.83 (Björkman and Demmig, 1987). However, ΦPSII measurements derived from fluorescence analysis can be subject to error (Lavergne and Trissl, 1995; Baker et al., 2007). Lavergne and Trissl (1995) estimate the maximum ΦPSII to be 0.88, while Vasili’ev and Bruce estimated the maximum quantum yield for charge separation in PSI (ΦPSI) and ΦPSII to be ∼0.95 (Vasil’ev and Bruce, 2004). Other estimates of maximum ΦPSI place values close to 1.0 (Nelson and Yocum, 2006). However, both photosystems can undergo rapidly reversible losses of efficiency as part of the regulation of light use by photosynthesis (Genty and Harbinson, 1996). Imperfect excitation transfer from the carotenoids to chl (Croce et al., 2001; de Weerd et al., 2003; van Oort et al., 2008) will decrease efficiency, as will light-induced damage (Aro et al., 1993; Sonoike, 1996). Slowly reversible down-regulation of efficiencies has also been reported (Demmig-Adams and Adams, 2006).
Electron and proton transport
The PET system incorporates linear and cyclic pathways (Fig. 1). Linear (or non-cyclic) electron transport follows the classic Z-scheme model from water to the terminal electron acceptor of PSI, Fd. Cyclic electron transport operates around PSI and is much less well defined than the linear pathway, and various components may be involved (Bendall and Manasse, 1995; Bukhov and Carpentier, 2004; Rumeau et al., 2007). Proton transport coupled to electron transport results in the formation of a transthylakoid proton potential (ΔμH+), or pmf (Kramer et al., 2003; Avenson et al., 2005a). This pmf is important for two reasons. First it drives the formation of ATP. The gating of protons, driven by the ΔμH+, through the ATPase results in the otherwise energetically unfavourable synthesis of ATP from ADP and Pi in the chloroplast stroma, thus linking electron transport to ATP formation. Secondly, the ΔμH+ is comprised of two components: a pH difference between the chloroplast stroma and the thylakoid lumen and a voltage difference across the thylakoid membrane, with the thylakoid lumen becoming more acid and electrically positively charged when electron transport occurs (Witt, 1979; Cruz et al., 2001). Acidification of the thylakoid lumen is important in regulating electron transport and modulating the non-photochemical quenching (NPQ) mechanism of PSII. An important consequence of these regulatory effects is that one of the results of electron transport, lumen acidification, is a down-regulation of electron transport and an up-regulation of photoprotection in PSII. Linear electron transport results in the formation of reductant and ATP, while cyclic electron transport results only in the formation of ATP with no net formation of reductant. The flux of electrons through these pathways is regulated at the level of the cytb6f complex. While some cyclic fluxes operating around PSII have been reported, these are not considered to be significant in vivo so will not be discussed further here.

Depiction of the thylakoid electron transport chain showing ferredoxin (Fd)-dependent electron transport-associated proton transport pathways. The linear electron transport pathway runs from water to Fd via PSII, the plastoquinol/plastoquinone (PQ) pool, the cytb6f complex and PSI. Protons released into the thylakoid lumen by water splitting and PQH2 oxidation by the cytb6f complex exit via the lumen the ATPase. This process is driven by the transthylakoid proton motive force and results in the formation of ATP from ADP and inorganic phosphate (Pi). Electrons can be transferred Fd to NADP (forming NADPH), or transferred to O2 to form superoxide, or they can enter a cyclic electron transport pathway around PSI. Several pathways of cyclic electron transport around PSI have been described, including the NAD(P)H dehydrogenase (NDH) pathway in which PQ is reduced by NADPH. Other possible cyclic routes include the Fd-quinone reductase pathway in which electrons are transferred back into the cytb6f complex.
Cyclic electron flow around PSI in higher plants consists of at least two partially redundant pathways known as the ferredoxin quinone oxidoreductase (FQR)- and NAD(P)H dehydrogenase (NDH)-dependent pathways (Miyake, 2010). Despite intensive efforts, FQR has not been found associated with any known chloroplast protein or protein complex. In contrast, it is now established that the NDH complex associates with PSI, a process that is considered to be important in facilitating NDH functions such as cyclic electron flow and chlororespiration (Peng et al., 2009, 2011). The NDH complex can interact with multiple PSI units to form NDH–PSI supercomplexes in the thylakoid membranes, an association that requires two minor LHCI proteins, Lhca5 and Lhca6 (Peng et al., 2009). The thylakoid PROTON GRADIENT REGULATION 5 (PGR5) and PGR 5-like 1 (PGRL1) proteins (Avenson et al., 2005b; Munekage et al., 2008) also associate with PSI in a manner that is considered to facilitate the operation of PSI cyclic electron transport (DalCorso et al., 2008). The NDH–PSI association may re-direct electron flow and so prevent over-reduction of the stroma in mutants such as pgr5, which are defective in PSI cyclic electron transport (Peng et al., 2009). In the NDH-dependent cyclic electron flow pathway, electrons are transported from PSI to the PQ pool via NADPH or reduced ferredoxin and the NDH complex.
Cyclic electron transport around PSI only predominates in some special cases, such as in the bundle sheath cells of some C4 leaves (Shikanai, 2007), though some mutants show clear up-regulation of cyclic electron transport (e.g. Livingston et al., 2010). The majority of PET follows the linear (or non-cyclic) pathway passing from water through PSII, the cytb6f complex, and PSI to Fd, and thereafter, for most electrons, to NADP. However, it is generally accepted that cyclic electron flow makes an important contribution to the overall regulation of electron transport in response to environmental variation and metabolic cues, even though the nature of these pathways and their precise functions remain controversial. Cyclic electron transport serves only to support the production of the pmf and thus allows ATP generation without the net formation of reductant, such as reduced Fd or NADPH. It is therefore believed that switching between cyclic and non-cyclic pathways provides a degree of flexibility in the ratio of ATP and NAPDH production and plants can hence adjust the ratio of ATP to NAPDH to meet the needs of metabolism (Hosler and Yocum, 1987; Munekage et al., 2008; Livingston et al., 2010).
Flexibility in the formation of pmf and reductant is not only important in meeting the needs of the Benson–Calvin cycle and other metabolic pathways, but it is also vital to the activation and regulation of photosynthesis in the transition from darkness to light, in response to rapid (i.e. on the time scale of seconds) fluctuations in the light environment and in atmospheric CO2 availability, and the acclimation to environmental stress. The operation of cyclic electron flow around PSI is considered to protect PSII by enhancing protonation of the lumen, thus limiting electron transport and triggering NPQ (Rumeau et al., 2007). By regulating electron transport into PSI via the cytb6f complex, cyclic electron flow also minimizes the probability of the formation of reactive oxygen species (ROS) such as superoxide (O2·–) on the acceptor side of PSI. The mid-point potential for the O2/O2·– couple is –160 mV while the redox potential of the stroma is close to –330 mV. Many of the stromal electron acceptors such as Fd, thioredoxin (TRX), and ferredoxin-NADP+ oxidoreductase (FNR) are thus able reduce O2 to O2–. This process, which is called the Mehler reaction or pseudocyclic electron flow, is important in photosynthetic control because it contributes to the prevention of over-reduction of the PET carriers and it produces oxidative signals that regulate gene expression (Foyer and Noctor, 2009). The addition of H2O2 to plant cells leads to substantial transcriptome re-programming (Desikan et al., 2001). Similarly, the expression of numerous genes was changed in mutants lacking a chloroplastic superoxide dismutase (SOD; Rizhsky et al., 2003).
The assimilation (A)/intercellular CO2 (Ci) curves of photosynthesis
The variable relationship between the PET chain and carbon metabolism can be illustrated by the A/Ci curves of photosynthesis, which are considered to show two main zones or phases of limitation (von Caemmerer, 2000). The first phase is observed at low Ci values and corresponds to a limitation by ribulose-1, 5-bisphosphate carboxylase/oxygenase (Rubisco)-catalysed carboxylation (Fig. 2). Under these conditions, which would be experienced by a leaf with closed or partially closed stomata, the capacity of electron transport could exceed that of metabolism. The second phase is observed at higher Ci values and corresponds to a limitation of photosynthesis by regeneration of ribulose 1, 5-bisphosphate (RuBP), which is believed to be substantially due to a limitation of electron transport capacity. Under these conditions, down-regulation of electron transport would be minimal.
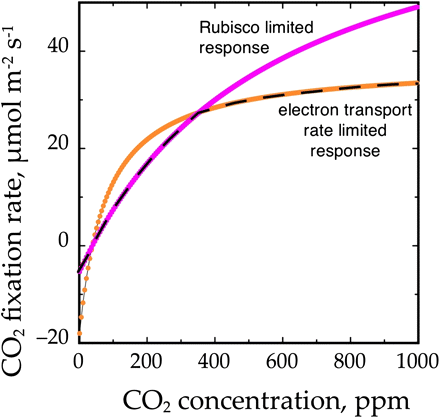
The relationship between CO2 concentration at the site of carboxylation (i.e. in the chloroplast stroma) and the rate of CO2 fixation, commonly referred to as an A/Ci curve, for a leaf in an atmosphere containing 21% (21 kPa) oxygen. The rate of CO2 fixation is the minimum of the either the Rubisco-limited rate (the Ac line) or the ribulose-1,5-phosphate-limited rate (the Aj line). The supply of ribulose-1,5-bisphosphate is strongly influenced by the rate of electron transport and thus irradiance, so for any leaf there are an infinite number of possible Aj lines, corresponding to different irradiances (only one is illustrated). The Ac line is determined by the amount of Rubisco, its activation state and temperature, so if the Rubisco is fully activated and temperature is constant there is only one Ac line.
The capacities for electron transport and metabolism are closely balanced by regulation of chloroplast structure and composition (Evans and Terashima, 1988) and by physiological regulation that controls the rate constants for electron and proton transport (Genty and Harbinson, 1996; Takizawa et al., 2008). For example, the Ci value of the transition point between the Rubisco- and electron transport-limited zones is offset relative to the growth Ci values (Ainsworth and Rogers, 2007). Similarly, the light-saturated, maximum rate of CO2 fixation is proportional to the leaf content of the Rubisco and cytb6f proteins (Onoda et al., 2005; Yamori et al., 2005). Although the rate constants for electron or transport processes such as P700+ reduction by electrons from the PQH2 pool (Fig. 1) can vary in response to increasing irradiance, the rate constants do not change significantly (i.e. there is little need for regulation) under steady-state conditions in the absence of stress, implying that the capacity of electron transport is well balanced with the capacity (or demand) of metabolism (Harbinson and Hedley, 1989; Laisk and Oja, 1994; Takizawa et al., 2008).
Regulation of electron and proton transport
The supply of ATP and reductant NADPH by the thylakoid membranes must be precisely matched to their use in metabolism (Noctor and Foyer, 2000). This coordination minimizes singlet oxygen production in PSII and superoxide production in PSI. Considered in isolation from metabolism, electron transport is generally limited by the availability of light (i.e. the rate of charge separation in PSI and PSII) or by the intrinsic capacity of the electron transport chain (particularly the intersystem electron transport chain) to transport electrons, while metabolism, which generates demand for ATP and reductant, is limited by the extent of enzyme activation.
The key to the regulation of electron transport is the pH sensitivity of the reaction between PQH2 and the Rieske FeS of the cytb6f complex. PQH2 is able to form an H-bond with a specific histidine residue adjacent to the binding pocket of the Rieske FeS protein. The formation of an H-bond between a hydroxyl group proton on the quinol head group of PQH2 and the lone pair of a nitrogen in the imidazole ring of the histidine residue is the first step in oxidation of the bound PQH2 (Crofts et al., 1999). Protonation of the lone electron pair on the histidine residue blocks H-bond formation. The pH optima for cytf reduction lie in the range 6.5–7.25 (Tikhonov et al., 1984; Nishio and Whitmarsh, 1993; Hope et al., 1994; Finazzi, 2002), with the highest value being found in the alga Chlamydomonas. The pH sensitivity of the PQH2–cytb6f reaction combined with decreases in lumenal pH offers a model for the regulation of linear and cyclic electron transport that explains the observed changes in the oxidation and reduction of components of the electron transport chain during regulation. This model, however, does not explain the mechanism that brings about the changes in lumen pH required for adjustment of the kinetics of the reaction between PQH2 and cytb6f. This mechanism will be discussed once the problem of regulating the ratio of ATP and reductant formation has been introduced because the mechanisms that regulate electron transport and the ratio of ATP to NADPH have much in common.
The ATP produced/reductant produced ratio is largely determined by two parameters: the number of protons translocated to the thylakoid lumen per unit electron transport (H+/e–), and the number of protons required to synthesize ATP (H+/ATP). These parameters, acting together, will determine the yield of ATP per unit linear electron transport. Studies on the structure and operation of the thylakoid ATPase (Seelert et al., 2000; Müller et al., 2001), in particular the ratio of the F0 and F1 subunits, gave an estimate of ∼4.7 H+ per ATP. Although the structure-based estimate for the H+/ATP ratio is currently widely accepted, some recent measurements of the H+/ATP stoichiometry made on the ATPases from Escherichia coli and spinach have cast some doubt on the plausible 4.7 H+ per ATP derived from structural studies (Steigmiller et al., 2008). The ATPases from E. coli and spinach have different stoichiometries for the F0 subunits (10 for E. coli and 14 for spinach), but in both cases the H+/ATP ratio is 4 (Steigmiller et al., 2008), a value which is consistent with earlier experimental determinations of the H+/ATP ratio. Whatever the H+/ATP of the ATPase, the effective H+/ATP ratio of the thylakoid will be increased by processes that allow the uncoupled dissipation of the ΔμH+. Several such processes can operate. They include slippage (the passage of protons through the ATPase without ATP formation; Nelson et al., 2002), uncoupling of the ATPase (Evron and Avron, 1990), and H+ leakage through the thylakoid membrane (Fuks and Homblé, 1996). All have been demonstrated in vitro and would decrease the H+/ATP stoichiometry. Slippage and leakage are expected to increase as the pmf increases, and uncoupling of the ATPase occurs when the stromal pH falls below ∼7.5 (the nominal value of the stromal pH is ∼7.5). These ΔμH+ dissipation mechanisms, which do not lead to ATP synthesis, are expected to occur when the electron transport chain is subject to down-regulation and the intrathylakoid pH decreases, though the impact of such processes on overall regulation remains to be quantified. In particular, the occurrence of slippage reactions under physiological conditions has been disputed (Junge et al., 2009). The minimum ΔμH+ and, by implication, the transthylakoid pH difference required to drive ATP synthesis will depend on the H+/ATP. Based on an H+/ATP of 4.7, Kramer et al. (2003) estimated that if the stromal pH was 7.5 and the ΔμH+ was divided equally between voltage and pH differences, then a lumen pH of no less than 6.5–7.0 would be enough to support ATP synthesis.
Uncertainty in the stoichiometry of proton transport into the thylakoid lumen coupled to electron transport hinges on the presence or absence of the Q-cycle in the cytb6f complex. Current concepts incorporate a Q-cycle around the cytb6f complex (Cape et al., 2006) that is always in operation (Rich, 1988; Sacksteder et al., 2000). If this is the case, the number of protons translocated into the thylakoid lumen per electron (H+/e–) is three for linear electron transport. The scale of any uncoupled dissipation accompanying this ΔμH+ formation is unknown and, although some leakage seems inevitable, there is no evidence that in vivo uncoupled dissipation of the ΔμH+ is controlled so as to regulate the ΔμH+. Given an H+/e– of 3 and an H+/ATP of 4.7, the ATP/e– is 0.64 at most (equal to an ATP/NADPH ratio of ∼1.3), and this value will decrease as leakage increases.
Balancing the varying energy requirements of CO2 fixation and photorespiration
The basic ATP/NADPH ratio required by CO2 fixation is 1.5 in the steady state (i.e. no photorespiration with stable Calvin cycle metabolite concentrations so that the rate of CO2 assimilated equals the rate of product formed). However, CO2 fixation is only one of many reductant- and ATP-requiring metabolic reactions occurring in the stroma. Many of these reactions such as nitrite and ammonia assimilation occur at much lower rates than CO2 fixation. They will therefore have only modest effects on the ATP/reductant demand of the stroma. Moreover, considerable energetic as well as metabolic cross-talk occurs between the energy-producing reactions of the chloroplasts and mitochondria, which are highly interdependent. These organelles cooperate in the coordinated regulation of photosynthesis, sucrose synthesis, and primary nitrogen assimilation (Foyer et al., 2011). Mitochondria also dissipate excess reducing equivalents produced by the chloroplasts and thus mutations in mitochondrial proteins can adversely affect chloroplast processes (Dutilleul et al., 2003; Noguchi and Yoshida, 2008). The metabolic and energy dialogue that occurs between the energy-producing organelles is also important in the regulation of gene expression. As discussed later, organelle energy metabolism impacts on the elaborate network of anterograde and retrograde signalling pathways between the chloroplasts, mitochondria, and nucleus that regulates organelle biogenesis and composition.
The oxygenation of RuBP, which occurs alongside CO2 fixation, drives photorespiration. Use of RuBP for oxygenation in parallel with CO2 fixation changes the ATP/reductant requirements associated with the Calvin cycle. First, unlike carboxylation, metabolism driven by oxygenation does not require reductant (in the chloroplast), but it does require ATP for the final reaction, which produces 3-phosphoglyceric acid (PGA) from glycerate. In addition, the oxygenation of RuBP will change the relative rates of the reductive phase of the Calvin cycle [PGA kinase/glyceraldehyde-3-phosphate dehydrogenase (GAPDH), ATP/NADPH=1] to the regeneration phase (ATP required by the phosphoribulokinase reaction). When both carboxylation and oxygenation operate in parallel, photorespiratory carbon metabolism in the chloroplast will increase the ATP/NADPH requirement from 1.5 to 1.62 (Table 1). However, if photorespiratory ammonia re-assimilation is taken into account, the effect of photorespiration on the required ATP/reductant ratio will be less marked (Table 1). The effects of photorespiration on ATP/reductant ratios may seem minor, and they are certainly less dramatic than the impact of oxygenation on net carbon fixation (Table 1). However, the key point is that even relatively small changes in the required ATP/NADPH ratio, such as from 1.5 to 1.6, are predicted to cause rapid de-regulation of chloroplast metabolite pools, particularly if the new demands are not met by appropriate adjustments in production by PET and the ATP synthase. This prediction is a consequence of the high turnover rates of adenylate and reductant pools during photosynthesis (Noctor and Foyer, 2000).
Theoretical ATP/NADPH requirements of photosynthesis, with or without photorespiration
ATP | NADPH (or 2e–) | ||
1. No photorespiration | |||
7 RuBP+7 CO2=14 PGA | – | – | |
14 PGA=14 G3P | 14 | 14 | |
11.7 trioses=7 Ru5P | – | – | |
7 Ru5P=7 RuBP | 7 | – | |
Net product=7/3=2.33 trioses | ATP/NADPH=21/14=1.50 | ||
2a. With photorespiration (carbon metabolism only) | |||
5 RuBP+5 CO2=10 PGA | – | – | |
2 RuBP+2 O2=2 PGA+2 2-PG | – | – | |
2 2-PG=1 glycerate+1 CO2 | – | – | |
1 glycerate=1 PGA | 1 | – | |
10+2+1 PGA=13 G3P | 13 | 13 | |
11.7 trioses=7 Ru5P | – | – | |
7 Ru5P=7 RuBP | 7 | – | |
Net product=4/3=1.33 trioses) | ATP:NADPH=21/13=1.62 | ||
2b. With photorespiration (including ammonia recycling) | |||
2 glycine=1 serine+1 CO2+1 NH3 | – | – | |
1 glutamate+1 NH3=1 glutamine | 1 | – | |
1 glutamine+1 2-OG=2 glutamate | – | 1 | |
ATP:NADPH=22/14=1.57 |
ATP | NADPH (or 2e–) | ||
1. No photorespiration | |||
7 RuBP+7 CO2=14 PGA | – | – | |
14 PGA=14 G3P | 14 | 14 | |
11.7 trioses=7 Ru5P | – | – | |
7 Ru5P=7 RuBP | 7 | – | |
Net product=7/3=2.33 trioses | ATP/NADPH=21/14=1.50 | ||
2a. With photorespiration (carbon metabolism only) | |||
5 RuBP+5 CO2=10 PGA | – | – | |
2 RuBP+2 O2=2 PGA+2 2-PG | – | – | |
2 2-PG=1 glycerate+1 CO2 | – | – | |
1 glycerate=1 PGA | 1 | – | |
10+2+1 PGA=13 G3P | 13 | 13 | |
11.7 trioses=7 Ru5P | – | – | |
7 Ru5P=7 RuBP | 7 | – | |
Net product=4/3=1.33 trioses) | ATP:NADPH=21/13=1.62 | ||
2b. With photorespiration (including ammonia recycling) | |||
2 glycine=1 serine+1 CO2+1 NH3 | – | – | |
1 glutamate+1 NH3=1 glutamine | 1 | – | |
1 glutamine+1 2-OG=2 glutamate | – | 1 | |
ATP:NADPH=22/14=1.57 |
Steady-state conditions are assumed. For the photorespiratory condition, the carboxylation:oxygenation (C:O) ratio is taken as 2.5. This is a fairly low value, though not excessively so (see Keys, 1999 for a discussion of likely C:O values under typical conditions). The rate of RuBP utilization is taken to be the same in the two conditions, and is set arbitrarily at 7 (see fig. 4 in Foyer et al., 2009 for an overview of CO2 and O2 exchange and photon requirements under similar assumptions).
G3P, glyceraldehyde 3-phosphate; 2-OG, 2-oxoglutarate; 2-PG, 2-phosphoglycollate; PGA, 3-phosphoglycerate; RuBP, ribulose 1,5-bisphosphate; Ru5P, ribulose 5-phosphate.
Theoretical ATP/NADPH requirements of photosynthesis, with or without photorespiration
ATP | NADPH (or 2e–) | ||
1. No photorespiration | |||
7 RuBP+7 CO2=14 PGA | – | – | |
14 PGA=14 G3P | 14 | 14 | |
11.7 trioses=7 Ru5P | – | – | |
7 Ru5P=7 RuBP | 7 | – | |
Net product=7/3=2.33 trioses | ATP/NADPH=21/14=1.50 | ||
2a. With photorespiration (carbon metabolism only) | |||
5 RuBP+5 CO2=10 PGA | – | – | |
2 RuBP+2 O2=2 PGA+2 2-PG | – | – | |
2 2-PG=1 glycerate+1 CO2 | – | – | |
1 glycerate=1 PGA | 1 | – | |
10+2+1 PGA=13 G3P | 13 | 13 | |
11.7 trioses=7 Ru5P | – | – | |
7 Ru5P=7 RuBP | 7 | – | |
Net product=4/3=1.33 trioses) | ATP:NADPH=21/13=1.62 | ||
2b. With photorespiration (including ammonia recycling) | |||
2 glycine=1 serine+1 CO2+1 NH3 | – | – | |
1 glutamate+1 NH3=1 glutamine | 1 | – | |
1 glutamine+1 2-OG=2 glutamate | – | 1 | |
ATP:NADPH=22/14=1.57 |
ATP | NADPH (or 2e–) | ||
1. No photorespiration | |||
7 RuBP+7 CO2=14 PGA | – | – | |
14 PGA=14 G3P | 14 | 14 | |
11.7 trioses=7 Ru5P | – | – | |
7 Ru5P=7 RuBP | 7 | – | |
Net product=7/3=2.33 trioses | ATP/NADPH=21/14=1.50 | ||
2a. With photorespiration (carbon metabolism only) | |||
5 RuBP+5 CO2=10 PGA | – | – | |
2 RuBP+2 O2=2 PGA+2 2-PG | – | – | |
2 2-PG=1 glycerate+1 CO2 | – | – | |
1 glycerate=1 PGA | 1 | – | |
10+2+1 PGA=13 G3P | 13 | 13 | |
11.7 trioses=7 Ru5P | – | – | |
7 Ru5P=7 RuBP | 7 | – | |
Net product=4/3=1.33 trioses) | ATP:NADPH=21/13=1.62 | ||
2b. With photorespiration (including ammonia recycling) | |||
2 glycine=1 serine+1 CO2+1 NH3 | – | – | |
1 glutamate+1 NH3=1 glutamine | 1 | – | |
1 glutamine+1 2-OG=2 glutamate | – | 1 | |
ATP:NADPH=22/14=1.57 |
Steady-state conditions are assumed. For the photorespiratory condition, the carboxylation:oxygenation (C:O) ratio is taken as 2.5. This is a fairly low value, though not excessively so (see Keys, 1999 for a discussion of likely C:O values under typical conditions). The rate of RuBP utilization is taken to be the same in the two conditions, and is set arbitrarily at 7 (see fig. 4 in Foyer et al., 2009 for an overview of CO2 and O2 exchange and photon requirements under similar assumptions).
G3P, glyceraldehyde 3-phosphate; 2-OG, 2-oxoglutarate; 2-PG, 2-phosphoglycollate; PGA, 3-phosphoglycerate; RuBP, ribulose 1,5-bisphosphate; Ru5P, ribulose 5-phosphate.
The balance between CO2 fixation and photorespiration and hence the ATP/reductant ratios that are required in C3 leaves depends on atmospheric CO2 availability (Farquhar et al., 1980; Lawlor, 2001). If CO2 fixation and photorespiration alone are considered, then the relative requirement for ATP and reductant (NADPH or two electrons) at 25 °C decreases from a ratio of 1.75 in the absence of carboxylation but with continued oxygenation (e.g. Ci=0 Pa, O2=210 kPa) to ∼1.5 in the absence of oxygenation, but with continued carboxylation (Fig. 3). When photorespiration rates are high, as occurs when the stomata are closed, the ATP/reductant (NADPH) requirement will be close to 1.75. Conversely, when the atmospheric CO2 content is enriched to values of ≥1000 μl l−1, the required ATP and reductant ratios are close to 1.5 (Fig. 3). Changes in the ratio of carboxylation and oxygenation of RuBP also occur in response to temperature changes (Long and Drake, 1992). In the absence of oxygenation, the ATP/NADPH ratio is at the minimum requirement level of 1.5 (Table 1).

Relationships between CO2 concentration at the site of carboxylation and the ratio of demand for ATP and NADPH for the combined activities of the Calvin–Benson and the photosynthetic carbon oxidation cycles in C3 leaves. Values were calculated using equations and parameters from von Caemmerer (2000) in an atmosphere containing 21% (21 kPa) oxygen. For a leaf in air and with open stomata the CO2 concentration in the stroma is ∼200 ppm (20 Pa). With partial or complete stomatal closure, values can fall to the CO2 compensation point, ∼50 ppm (5 Pa), depending on the temperature and the respiration rate. Increases in atmospheric CO2 concentrations will result in higher stromal CO2 concentrations and a lower ATP/NADPH demand.
As linear electron transport translocates three H+ into the lumen per electron, the formation of two NADPH would be coupled to the synthesis of only 2.6 ATP (12/4.7, assuming the 4.7 H+ per ATP is correct); that is, a shortfall of 0.4 ATP. Increased rates of oxygenation of the substrate, RuBP, will increase the ATP/NADPH ratio because of the additional requirement of the photorespiratory pathway (Table 1). Starch synthesis in the chloroplasts and sucrose synthesis in the cytosol also require ATP, and thus are another factor in the chloroplast’s budget for ATP and reductant, but one which is more difficult to account for in a quantitative manner. Starch synthesis and degradation occur simultaneously in chloroplasts, the flux in each direction being dependent on the supply of triose phosphate and the regulation of the component enzymes.
Pathways to boost ATP production
Two well known pathways are potentially able to boost ATP production while not themselves supporting easily measurable oxygen evolution. These are (i) cyclic electron transport and (ii) pseudocyclic electron transport, with the latter having other commonly used names (e.g. Mehler reaction, water–water cycle). As the operation of both these pathways is ‘hidden’ from standard measurements of O2 exchange, their extent remains subject to debate. Increases in the rate of cyclic electron transport offer a mechanism for increasing the rate of ATP formation relative to the formation of reductant.
In addition to this thylakoid level flexibility, there is also flexibility arising from processes in the stroma. Not all the reductant formed by linear electron transport is associated with processing the products of carboxylation and oxygenation of RuBP, and these other sinks for reductant change the ratio of ATP/reductant demand in the stroma. Under all conditions some reductant is used to reduce O2 to (O2–) in the Mehler reaction. This is the first step of the water–water cycle. This traditionally incorporates pseudocyclic electron transport, the Mehler–peroxidase reaction, and the ascorbate/glutathione cycle (Asada, 2000), but it now most probably also includes peroxiredoxin-dependent pathways. Two electrons are required to produce two superoxide molecules from two molecules of O2, supporting the evolution of 0.5 O2 at PSII. Assuming that hydrogen peroxide (H2O2) production occurs only through superoxide dismutation (rather than superoxide reduction), two superoxide (O2·–) radicals undergo a dismutation reaction to produce one molecule each of O2 and H2O2. Reduction of the H2O2 molecule requires two electrons to regenerate reductants oxidized via ascorbate peroxidase activity, and this also supports the evolution of 0.5 O2 at PSII. The end result is that one O2 is evolved at PSII, two are consumed by the electron transport chain, and one is evolved by SOD. Overall, this process necessarily involves four electrons (i.e. four per O2 evolved from or reduced to water), and neither easily measurable O2 exchange nor net production of reducing power for biosynthetic reactions.
Pseudocyclic electron transport occurs alongside processes that support net O2 evolution, but its hidden nature means that it is usually detected by indirect methods. In consequence, the literature contains very variable estimates of the proportion of electrons that flow to O2 in the Mehler reaction. One consequence of O2 reduction is some contribution to the transthylakoid proton gradient. As well as the protons released into the thylakoid from H2O oxidation or during PQH2 oxidation by the cytb6f complex, this mechanism also involves proton consumption in the stroma as O2 is reduced to water. It will therefore build up ΔμH+ and allow the formation of more ATP without net reductant formation. It is also expected to increase in activity as the stroma becomes more reduced because the regeneration of electron acceptors (NADP+, oxidized Fd) becomes limiting. Because this is an expected first consequence of a shortfall of ATP relative to reductant, O2 reduction may be an important self-correcting mechanism to balance ATP production relative to NADPH. This aspect of O2·– production and metabolism has become increasingly overlooked in recent years, as the research focus has shifted to the roles of ROS in oxidative stress and/or signalling.
Another mechanism that could operate when the stroma becomes over-reduced is the reduction of oxaloacetate by NADP-malate dehydrogenase (NADP-MDH), which oxidizes NADPH, with the malate formed then being available for export from the chloroplast via the malate shuttle (Scheibe, 2004). The export of malate removes reductant from the ATP/reductant balance of the stroma; the malate is re-oxidized in the cytosol to oxaloacetate, which can then exchange for malate via the shuttle. The activity of NADP-MDH is subject to regulation via reduction of regulatory thiol groups by TRX, with increasing reduction of the TRX pool increasing the activation state of the enzyme. Changes in the activity of NADP-MDH have been observed under a range of conditions, such as increasing irradiance and during photosynthetic induction (Harbinson et al., 1990; Foyer et al., 1992). The malate valve is therefore considered to be a central regulator of the export of reductant from the stroma. Finally, although most of stromal demand for reductant (as NADPH or reduced Fd) and ATP (estimated to be ∼90%) is associated with photosynthetic carbon metabolism, other chloroplast processes also require reductant and/or ATP. Of these perhaps the most important are associated with primary nitrate assimilation and sulphur assimilation, as enzymes such as nitrite reductase and sulphite reductase are localized in the chloroplasts. These reductive processes would result in the net build up of the ΔμH+, though nitrite reduction is likely to be more significant than the slower reduction of sulphite.
The total net formation of ATP and reductant needs therefore to be viewed at a whole system level; flexibility at the thylakoid level needs to be integrated with flexibility at the stromal and cytosolic levels (any reductant exported from the chloroplast needs, after all, to be used somewhere). Of the various processes that influence the ratio of ATP and (net) reductant formation, the rates of pseudocyclic and cyclic electron transport have been proposed to increase in response to the accumulation of reductant in the stroma (Hosler and Yocum, 1987; Breyton et al., 2006). In addition, activation of NADP-MDH in response to increasing stromal reduction would be expected to increase the export of reductant via the malate valve. So any increase in the degree of reduction of the stroma will increase the rate of reactions that tend to increase the ΔμH+ with no net increase in stromal reduction. This can compensate for the relatively low ATP/reductant ratio of linear electron transport. Finally, the presence of other metabolic pathways in the stroma other than the predominant pathways associated with CO2 and photorespiration play a role in determining the total demand for ATP and reductant.
Cyclic electron transport is not normally considered as a major flux in C3 leaves. At most, it is probably <10% of the linear flux (Baker and Ort, 1992; Avenson et al., 2005a). In some circumstances, however, such as the induction phase of photosynthesis (Joliot and Joliot, 2006) or in the absence of CO2 (Harbinson and Foyer, 1991), cyclic electron transport rates can be much higher. Cyclic electron transport is more important in the bundle sheath cells in C4 leaves, where it may achieve much high rates.
Pseudocyclic electron flow to O2 generally accounts for only ∼10% of the linear flux (Genty and Harbinson, 1996; Badger et al., 2000; Ort and Baker, 2002), but higher rates have been reported in plants exposed to stress (Cheeseman et al., 1997; Fryer et al., 1998; Farage et al., 2006). It is also assumed that up to ∼10% of linear electron transport could be used for processes other than carbon metabolism such as nitrite reduction (De la Torre et al., 1991; Lewis et al., 2000). Using a model-based approach, it has been estimated that the export of reductant from the chloroplast as malate accounts for ∼5% of linear electron transport (Fridlyand et al., 1998).
The mechanisms that regulate chloroplast ATP/reductant ratios could also act to down-regulate electron transport processes by decreasing intrathylakoid pH. If metabolic demand for reductant were to be diminished, then the increase in the reduction state of the stromally located electron transport system (Fd, etc.) that would then develop would increase the rates of cyclic electron transport, the Mehler reaction, and export via the malate shuttle. The result of this would be a decrease in the intrathylakoid pH, slowing electron transport and resulting in an increase in ATP/ADP.
An elegant, alternative mechanism developed by Kramer and co-workers suggests that the decrease in intrathylakoid pH can arise as the result of an increased kinetic limitation of the ATPase (Kramer et al., 2003, 2004; Takizawa et al., 2008). This would require a greater pH gradient, and thus a lower intrathylakoid pH, for the same rate of ATP synthesis. Any decrease in intrathylakoid pH would result in down-regulation of the reaction between PQH2 and the cytb6f complex, slowing electron transport of all kinds. The hierarchy of mechanisms acting in vivo to restrict linear electron transport in response to decreased metabolic demand remains to be demonstrated. Arabidopsis mutants that lack both the PGR5 and chlororespiratory reduction (CRR) proteins have a greatly restricted capacity for cyclic electron transport. PGR5 is believed to lack FQR activity, while the CRR mutants lack NDH activity. These mutants have restricted growth at low irradiances and have much decreased levels of chl in the leaves (Munekage et al., 2004). The simplest interpretation of these results is that other mechanisms that build up the ΔμH+ cannot compensate for a shortfall in cyclic electron transport capacity in Arabidopsis.
Regulation of light use efficiency and NPQ in PSII
A characteristic of PSII is its sensitivity to light-induced damage (Aro et al., 1993; Yokthongwattana and Melis, 2006) because of singlet oxygen (1O2) production in the reaction centre of PSII. 1O2 directly damages the PSII D1 reaction centre protein (Telfer et al., 1999; Krieger-Liszkay et al., 2008). This vulnerability means that each PSII reaction centre is rebuilt about once every 20–30 min, even under optimal irradiances. The photodamage to PSII requires light and increases when the reaction centre quinone in the PSII reaction centre (QA) is reduced. Limitation of electron transport after PSII will increase the extent of QA reduction. This phenomenon arises with increasing irradiance because of the increasingly limited capacity for electron transport through the cytb6f complex. This effect will be exacerbated if electron transport through cytb6f is restricted by down-regulation (Genty and Harbinson, 1996).
Singlet oxygen is a major oxidative signal in chloroplasts, 1O2 accumulation leading to substantial re-programming of gene expression (Gadjev et al., 2006). Evidence in support of this role was provided by analysis of the Arabidopsis fluorescent (flu) mutant that accumulates the sensitizing compound, protochlorophyllide, an intermediate in chl biosynthesis in the dark (op den Camp et al., 2003). When transferred into the light after a period of darkness, the flu mutant produces large amounts of 1O2, leading to major changes in nuclear gene expression (op den Camp et al., 2003). Annulling expression of the EXECUTOR1 and 2 genes, which encode thylakoid proteins, in the flu background suppresses the flu gene response, suggesting a role for these proteins in the retrograde 1O2 signalling (Lee et al., 2007a). Singlet oxygen selectively activates nuclear genes that are either not responsive or are less responsive to superoxide or H2O2, suggesting that activation by 1O2 might require promoter elements that are different from those used by H2O2.
The production of singlet oxygen and the protection of PSII from damage is minimized under conditions of limiting electron transport capacity through the cytb6f complex by protective mechanisms, the most important of which is called non-photochemical quenching (of chl fluorescence), or NPQ. Sometimes the name is qualified to imply that it is inducible or regulated (e.g. Hendrickson et al., 2004) to distinguish it from the basal or constitutive mechanisms that would operate to dissipate excitation energy in PSII even in the absence of NPQ.
In the absence of stress, energy-dependent quenching (qE) is the dominant component of NPQ that develops at irradiances above light-limiting levels and it increases with irradiance. The formation of qE depends on lumen acidification resulting from electron transport and upon the presence of the PsbS protein of PSII. Lumen acidification results in protonation of the PsbS protein, triggering qE formation (Li et al., 2004; Horton et al., 2005). The formation of the xanthophyll pigment, zeaxanthin, is also induced by lumen acidification (Kramer et al., 2003). The binding of a fraction of the zeaxanthin pool to an as yet unknown protein (not PsbS; Johnson and Ruban, 2010) also facilitates the quenching process induced by lumen acidification (Ruban et al., 2012). Zeaxanthin accumulation results in enhanced quenching at higher (although still acidic) lumen pH values, consistent with earlier observations (Noctor et al., 1991).
Much uncertainty remains regarding the precise mechanism of the qE mechanism, and several models have been proposed (Jahns and Holzwarth, 2012; Ruban et al., 2012). While it is clear that pH changes, facilitated by the presence of zeaxanthin and PsbS, result in structural changes in PSII, it is still not certain how these changes result in the formation of quenching centres. The consequence of qE is, however, certain: 1chl* formed in PSII is quenched and thus PSII is protected from damage. NPQ and qE work alongside QA reduction to diminish the quantum yield for PSII electron transport. In this way, the rate of PSII electron transport, i. e. the product of the quantum yield and light absorbed by PSII, is kept in balance with the capacity for electron transport through the rest of the thylakoid electron transport chain. Although qE is the most commonly encountered form of PSII regulation, other mechanisms exist and are important under some circumstances (Demmig-Adams and Adams, 2006).
Acclimation of photosynthesis in C3 plants to high CO2 and its absence in C4 species
The responses of different plant species, such as Arabidopsis, soybean, maize, rice, and poplar, to growth with CO2 enrichment has been extensively characterized over the last 30 years (Miyazaki et al., 2004; Gupta et al., 2005; Taylor et al., 2005; Ainsworth et al., 2006; Li et al., 2008; Fukayama et al., 2009, 2011; Leakey et al., 2009b; Tallis et al., 2010; Prins et al., 2011; Queval et al., 2012). Growth with CO2 enrichment has a positive effect on the plant growth, and yield of C3 species by the stimulation of yield is often much smaller than expected in many crop species (Long et al., 2004; Ainsworth and Long, 2005; Leakey et al., 2009a). When C3 leaves are exposed to elevated CO2 there is an immediate increase in photosynthesis (Kramer, 1981; Cure and Acock, 1986) because of decreased photorespiration, which is negatively affected by an increase in atmospheric CO2/O2 ratios (Bowes, 1991). A stimulation of net photosynthesis can be observed even when plants are grown for long periods at high CO2 (Idso and Kimball, 1992; Gunderson et al., 1993; Davey et al., 1999; Ainsworth et al., 2003; Ainsworth and Long, 2005). However, photosynthetic capacity is often decreased in plants grown for long periods at elevated CO2, a process that is known as ‘acclimation’ (Paul and Foyer, 2001). The acclimation of photosynthesis observed in C3 plants grown for long periods at elevated CO2 is related to a substantial re-programming of gene expression (Fukayama et al., 2009, 2011; Leakey et al., 2009b).
When C3 plants are grown for long periods under high CO2, they tend to become carbohydrate rich (Delucia et al., 1985; Sasek et al., 1985; Peet et al., 1986; Sage et al., 1988; Yelle et al., 1989a, b; Stitt, 1991) and CO2 fixation becomes progressively attenuated by decreases in Rubisco protein and/or activity (Sage et al., 1988; Rowland-Bamford et al., 1991; Chen et al., 2005). Alterations of gene expression patterns in response to elevated CO2 are at least in part related to altered sugar signalling because the expression of photosynthetic genes is rapidly inhibited when plants are supplied with exogenous sugars (Krapp et al., 1991). Field studies have consistently confirmed that reduced or insufficient sink capacity leads to an increase in leaf carbohydrates and down-regulation of photosynthetic capacity. While genetic factors play an important role in photosynthetic response to high CO2, the down-regulation of transcripts encoding Rubisco and other proteins associated with CO2 fixation and an up-regulation of those encoding proteins involved in RuBP regeneration and starch synthesis is a common response to long-term growth with CO2 enrichment (Leakey et al., 2009a).
The acclimation of photosynthesis to long-term growth with CO2 enrichment is also related to enhanced nutrient demands, particularly for nitrogen and phosphorus. Nitrogen uptake and assimilation can fail to keep pace with the higher rates of photosynthesis and assimilate production. There is therefore a tendency for the carbon/nitrogen ratios of plant tissues to increase under high CO2 and the leaves suffer a progressive nitrogen deficit (Bloom et al., 2010). Furthermore, growth at elevated CO2 can inhibit shoot nitrate assimilation in C3 plants if primary nitrate assimilation in the cytosol requires photorespiration for the provision of NADH (Rachmilevitch et al., 2004; Bloom et al., 2010). Long-term growth with CO2 enrichment improves nitrogen use efficiency and favours higher rates of dark respiration (Leakey et al., 2009a).
The acclimation of photosynthesis to elevated CO2 observed in C3 plants is largely absent from C4 plants (Prins et al., 2011). Experiments conducted in free-air concentration enrichment (FACE) experiments have consistently shown that photosynthesis in C4 plants such as maize is not changed when plants are grown at elevated CO2 (Leakey et al. 2006, 2009a). Moreover, leaf transcriptome patterns are similar in leaves from plants at high CO2 and those grown in air (Kim et al. 2006; Leakey et al. 2009a, b; Prins et al., 2011).
Control of photosynthetic gene expression
The expression of nuclear genes encoding photosynthetic proteins is influenced by a plethora of metabolic, developmental, and environmental triggers. However, the unifying central feature of this control is that expression of photosynthetic proteins is highly responsive to chloroplast (retrograde) signals that reflect the metabolic state of the organelle (Fey et al., 2005). The components of the photosynthetic electron transport chain are important sensors of light quantity and quality, over-reduction, overoxidation, or imbalances between components that trigger long- and short-term light acclimation processes that serve to optimize light harvesting and CO2 fixation for growth and development (Havaux and Niyogi, 1999; Karpinski et al., 1999, 2003; Niyogi, 1999, 2000; Pfannschmidt et al., 1999; Muller et al., 2001; Mateo et al., 2004, 2006). Changes in photosynthetic energy metabolism are sensed by oxidative and reductive signalling pathways that regulate gene transcription and post-transcriptional processing (Pfannschmidt and Liere, 2005). Metabolites and proteins that are exchanged between the chloroplasts, cytosol, and nucleus also make a key contribution to the coordination of gene expression in the nucleus and chloroplast (Baier and Dietz, 2005; Weber and Fischer, 2007). Mitochondria are also important in cellular energy and redox metabolism, and it is therefore not surprising that they influence the transmission of retrograde chloroplast signals to the nucleus (Pesaresi et al., 2006; Pfannschmidt, 2010).
Light drives photosynthetic electron transport and is the most important factor in the control of photosynthetic gene expression (Ma et al., 2001; 2002; Kurth et al., 2002; Im et al., 2006). The abundance of transcripts encoding photosynthetic proteins is generally highest at the end of the night period and at the beginning of the light period (Bläsing et al., 2005). The diurnal patterns of photosynthetic gene expression are fundamentally controlled by circadian clock regulators with input from photoreceptors such as phytochromes, crytochromes, phototropins, and Zeitlupe-like proteins (Ma et al., 2001; Jiao et al., 2005; Ghassemian et al., 2006). Phytochromes mediate the control of plant development by light and influence plant architecture and flowering dormancy (Schäfer and Bowler, 2002). Phototropins control light-induced movement responses that are considered to optimize photosynthesis. Crytochromes are important in regulating plant responses to excess excitation energy responses (Kleine et al., 2007). Excess excitation energy describes light energy that cannot be used to drive photosynthetic metabolism (Karpinski et al., 1997, 1999; Niyogi, 1999, 2000; Fryer et al., 2003).
Exposure to high light causes rapid re-programming of gene transcription (Pogson et al., 2008). This can involve the rapid repression of genes encoding light-harvesting complex (LHC) components as well as PSII and PSI reaction centre subunits (Kimura et al., 2003a, b; Atienza et al., 2004; Murchie et al., 2005; Fischer et al., 2006). The repression of LHC genes by high light is found in organisms as diverse as barley and Dunaliella (Masuda et al., 2003; Atienza et al., 2004). Transcripts encoding proteins involved in photoprotection such as the PSII-S and EARLY LIGHT-INDUCIBLE PROTEIN 2 (ELIP2) are enhanced in high light (Kimura et al., 2003a; Murchie et al., 2005). PSII-S is involved in NPQ and energy dissipation in PSII (Li et al., 2000). ELIP2 is considered to be a sensor and negative regulator of chl biosynthesis (Tzvetkova-Chevolleau et al., 2007).
Responses to changing light intensity involve changes not only in photosynthesis but also in leaf water status and temperature that activate multiple signalling pathways including abscisic acid (ABA), salicylic acid (SA), and other hormone-mediated signal transduction systems (Fryer et al., 2003; Mateo et al., 2006). Light-induced changes in stomatal conductance result in a photorespiratory H2O2 burst (Mateo et al., 2004, 2006). H2O2 signalling from the peroxisome contributes to the information on the redox changes in the PET chain, particularly the PQ pool, that influence photosynthetic gene expression. Limitations in CO2 availability that stimulate photorespiratory flux, which occur concomitantly with over-reduction of the PQ pool, trigger programmed cell death responses (Mühlenbock et al., 2007, 2008; Szechyńska-Hebda et al., 2010). Transcriptome responses to excess excitation energy can thus share common features with responses to infection by biotrophic pathogens with induction of local and systemic defence responses and defence gene expression (Mühlenbock et al., 2007a, b; 2008). High CO2 is able to prevent many of these negative effects of exposure to high light by preventing over-reduction of the PET chain. The level of CO2 can also influence photosystem composition. For example, high light stress under high CO2 led to the specific induction of genes encoding LHC and PSI subunits in Chlamydomonas (Im et al., 2003).
Exposure to UV-B often decreases photosynthetic gene expression (Mackerness et al., 1997; Casati and Walbot, 2003; Izaguirre et al., 2003; Jenkins, 2009). However, in Arabidopsis seedlings, transcripts encoding PSII, PSI, and LHC were increased following exposure to UV-B (Peschke and Kretsch, 2011). The UVB-RESISTANCE 8 (UVR8) protein is considered to be an important UV-B photoreceptor. It functions upstream of the ELONGATED HYPOCOTYL 5 (HY5) and CONSTITUTIVELY PHOTOMORPHOGENIC 1 (COP1) transcription factors (Rizzini et al., 2011). COP1 is inactivated by photoreceptor signalling in the light and participates in the inactivation of light-responsive genes in the dark (Ma et al., 2002). HY5 is also important in photoreceptor signalling pathways (Lee et al., 2007b).
Chl biosynthesis precursors (porphyrins) have long been known to play an important role in chloroplast to nucleus retrograde signalling pathways (Johanningmeier and Howell, 1984; Nott et al., 2006; Beale, 2011). The genomes uncoupled (gun) mutants, gun2, 3, 4, and 5, are affected in enzymes of porphyrin metabolism in Arabidopsis (Mochizuki et al., 2001; Larkin et al., 2003). The concept that Mg-protoporphyrin IX and its monomethylester are signals in retrograde signalling (Oster et al., 1996; Kropat et al., 1997; La Rocca et al., 2001) was challenged by findings showing no correlation between the quantity of Mg-protoporphyrin IX and the expression of chloroplast-associated nuclear genes (Mochizuki et al., 2008; Moulin et al., 2008). The recent characterization of a new Arabidopsis gun mutant (gun6-1D) suggests a role for haem synthesized by the plastid ferrochelatase I as a regulator of photosynthesis-associated nuclear genes (Woodson et al., 2011). The ABA-insensitive (ABI) 4 transcription factor has been implicated in the GUN1 (a chloroplast-localized pentatricopeptide repeat protein) pathway (Koussevitzky et al., 2007). Arabidopsis mutants lacking a ζ-carotene desaturase have impaired biosynthesis of carotenoids, which are required for both photoprotection of photosystem function and ABA synthesis. These mutants also show a repression of CHLOROPHYLL A OXYGENASE (CH1 or CAO), PROTOCHLOROPHYLLIDE OXIDOREDUCTASE B (PORB), Lhcb1.1, Lhcb1.4, and RbcS expression (Dong et al., 2007).
The central role of the plastoquinone pool
The photosystems have different ranges of light absorption, with maxima at 680 nm for PSII and 700 nm for PSI, respectively. Changes in light quality can therefore lead to imbalances in light capture by the photosystems. It is not surprising, therefore, that the relative abundance of PSII and PSI is regulated by light quality to ensure optimal photosynthesis efficiency. The specific excitation of PSII (PSII-light) leads to the induction of genes encoding PSI proteins and the repression of genes encoding PSII proteins (Pfannschmidt et al., 1999). Similarly, excitation of PSI (PSI-light) induces an inverse pattern of expression (Pfannschmidt et al., 1999). In early studies, the electron transport inhibitors 3-(3,4-dichlorophenyl)-1,1-dimethylurea (DCMU) and 2,5-dibromo-3- methyl-6-isopropyl-p-benzoquinone (DBMIB), which induce either oxidation or reduction of the PQ pool, respectively, were used extensively to study the effects of the reduction state of the PQ pool on chloroplast to nucleus retrograde signalling pathways (Escoubas et al., 1995; Karpinski et al., 1999). Accumulating evidence demonstrates that the redox state of the PQ pool modulates several independent redox signalling pathways that serve to regulate photosynthetic efficiency by the post-translational modification of existing light-harvesting and reaction centre proteins and by the activation of reaction centre gene transcription (Allen et al., 2011).
Enhanced reduction of the PQ pool, which can result, for example, from an imbalance in the rate of excitation of PSII relative to PSI, activates the redox-regulated STN7 protein kinase in the thylakoid membranes (Lemeille and Rochaix, 2010; Pesaresi et al., 2010). The STN7 kinase phosphorylates the LHCIIs associated with PSII (Pesaresi et al., 2009). Phosphorylation of LHCII causes a subpopulation of LHCII to move from PSII to PSI. This process re-distributes absorbed excitation energy so that the relative rates of photochemical conversion and turnover in each reaction centre are balanced. Moreover, STN7 activation provides an input signal for a further kinase signalling network that involves STN8, an orthologue of STN7 (Pesaresi et al., 2009). The chloroplast sensor kinase (CSK) also plays an important role in the adjustment of PSII and PSI stoichiometries (Puthiyaveetil et al., 2008). Unlike STN7, CSK does not regulate LHCII phosphorylation but rather it controls photosystem stoichiometry by the regulation of the transcription of photosynthetic genes encoding photosystem proteins (Allen et al., 2011).
A second major target for redox-regulated protein phosphorylation is the chloroplast RNA polymerase complex called the plastid-encoded polymerase (PEP). A Ser/Thr protein kinase, which was originally called the plastid transcription kinase and is now better known as cpCK2 because it is a nuclear-coded plastid-targeted casein kinase 2, is associated with the phosphorylation of PEP (Baginsky et al., 1997; Baena-Gonzalez et al., 2001) and plastid sigma factors (Shimizu et al., 2010). cpCK2 plays a key role in the regulation of the chloroplast phosphoproteome, and is considered to be particularly important in the cross-talk between chloroplast metabolism and gene expression (Reiland et al., 2009). The plastid sigma factors, which are encoded by six genes (AtSig1–AtSig6) in Arabidopsis thaliana, regulate the transcription of the photosynthetic reaction centre genes such as PsbA, which encodes the PSII D1 reaction centre protein, and PsaA/PsaB, which encode the PSI reaction centre apoproteins (Schweer et al., 2010; Shimizu et al., 2010).
Glutathione, TRX, and other reductants such as NADPH and Fd are also involved in the regulated expression of PET chain components (Baena-Gonzalez et al., 2001; Baier and Dietz, 2005; Brautigam et al., 2010; Dietz and Pfannschmidt, 2011). Exposure to PSII-light favours increased reduction of the PQ pool and an oxidation of chloroplast TRX, whereas PSI-light promotes the inverse effects of PQ oxidation and TRX reduction (Bräutigam et al., 2010). The signalling cascades triggered by reduction of the PQ pool interface with the other signalling pathways triggered by the PET chain and metabolism, particularly the singlet oxygen, H2O2, and glutathione pathways. Redox signalling pathways involving some of these components have been considered at length in other reviews and will therefore not be considered here in detail (see, for example, Foyer and Noctor, 2009; Foyer and Shigeoka, 2011; Noctor et al., 2012, and references therein).
Evidence for photosynthetic control of gene expression associated with photorespiration
Substantial re-programming of photosynthetic gene expression occurs when C3 plants are grown under conditions where photorespiration is diminished or inhibited (Miyazaki et al., 2004; Gupta et al., 2005; Taylor et al., 2005; Ainsworth et al., 2006; Li et al., 2008; Fukayama et al., 2009, 2011; Leakey et al., 2009b; Tallis et al., 2010; Prins et al., 2011; Queval et al., 2012). In contrast, in comparisons of leaf transcriptomes of C4 plants grown with CO2 enrichment or with air, such changes in gene expression patterns are much less apparent (Leakey et al. 2006, 2009a, b; Prins et al., 2011). Such observations are consistent with the absence of a high CO2-dependent stimulation of photosynthesis or growth in C4 plants such as maize (Leakey et al. 2006, 2009a, b). However, growth with CO2 enrichment alters stomatal opening and conductance in a similar manner in C3 and C4 plants. For example, stomatal conductance values were significantly higher in leaves of maize plants grown under ambient CO2 conditions compared with those from plants grown at high CO2 (Prins et al., 2011). Regardless of the CO2-dependent effects on whole plant water status, C4 leaf transcriptome patterns are similar in plants unchanged by atmospheric CO2 availability (Kim et al., 2006; Leakey et al. 2009a, b; Prins et al., 2011). Patterns of transcriptional changes occurring following the transition from growth at high CO2 to air in C3 leaves might therefore be used as an indicator of the influence of photorespiration on the photosynthetic control of gene expression (Queval et al., 2012). The published data sets for A. thaliana leaves of plants grown in high CO2 (3000 μl l−1) and then transferred to ambient conditions (390 μl l−1 CO2) for 2 d and 4 d in air (Queval et al., 2012) were used to determine the differential expression of photosynthesis genes (Supplementary Table S1 available at JXB online). Of the probe sets (22 810) present on the A. thaliana microarray chips, ∼10% displayed modified expression under photorespiratory conditions compared with high CO2, using a t-test calculation with selection of genes with P-values <0.05 and fold changes (in log2) of >0.5 and less than –0.5 for each comparison (either high CO2/air 2 d or high CO2/air 4 d). The significantly induced or repressed genes were classified into functional categories (Table 2) by using MAPMAN (Thimm et al., 2004). A large number of genes involved in signalling, protein synthesis, and degradation or encoding transcription factors were modified following the transition from high CO2 to photorespiratory conditions (Table 2). Similar observations were made in rice (Fukayama et al., 2009) and soybean (Ainsworth et al., 2006) leaves, where a large number of transcripts encoding transcription factors were responsive to atmospheric CO2 availability (Fukayama et al., 2009). Taken together, available data show that CO2 availability exerts a much greater major influence on the genes involved in signal transduction and protein metabolism in C3 species than on those encoding proteins with functions in primary processes such as carbon, nitrogen, or lipid metabolism (Ainsworth et al., 2006; Fukayama et al., 2009; Queval et al., 2012). Of the 104 differentially expressed genes involved in photosynthesis, photorespiration, respiration, and carbon metabolism in A. thaliana (Table 2), only 31 transcripts encode proteins annotated to be involved in photosynthesis and carbohydrate metabolism (Figs 4, 5).
Arabidopsis thaliana genes that respond to atmospheric CO2 availability (Queval et al., 2010)
Functional categories | Number of genes | |
Induced | Repressed | |
Protein metabolism | 95 | 200 |
Transcription factors | 94 | 151 |
Signalling | 26 | 135 |
Stress response | 38 | 68 |
Photosynthesis, (photo)respiration and carbon metabolism | 34 | 70 |
Transport | 30 | 68 |
DNA synthesis and repair | 5 | 74 |
Development | 26 | 49 |
Hormone metabolism | 30 | 33 |
Secondary metabolism | 28 | 20 |
Nitrogen and sulphur metabolism | 9 | 26 |
Lipid metabolism | 16 | 17 |
Cell wall metabolism | 12 | 21 |
Cell cycle and division | 0 | 32 |
Redox processes | 17 | 10 |
Unknown function | 315 | 381 |
Functional categories | Number of genes | |
Induced | Repressed | |
Protein metabolism | 95 | 200 |
Transcription factors | 94 | 151 |
Signalling | 26 | 135 |
Stress response | 38 | 68 |
Photosynthesis, (photo)respiration and carbon metabolism | 34 | 70 |
Transport | 30 | 68 |
DNA synthesis and repair | 5 | 74 |
Development | 26 | 49 |
Hormone metabolism | 30 | 33 |
Secondary metabolism | 28 | 20 |
Nitrogen and sulphur metabolism | 9 | 26 |
Lipid metabolism | 16 | 17 |
Cell wall metabolism | 12 | 21 |
Cell cycle and division | 0 | 32 |
Redox processes | 17 | 10 |
Unknown function | 315 | 381 |
The number of transcripts that were significantly induced or repressed in leaves 2 d and 4 d after transfer from high CO2 growth conditions to air are listed according to Functional categories.
Arabidopsis thaliana genes that respond to atmospheric CO2 availability (Queval et al., 2010)
Functional categories | Number of genes | |
Induced | Repressed | |
Protein metabolism | 95 | 200 |
Transcription factors | 94 | 151 |
Signalling | 26 | 135 |
Stress response | 38 | 68 |
Photosynthesis, (photo)respiration and carbon metabolism | 34 | 70 |
Transport | 30 | 68 |
DNA synthesis and repair | 5 | 74 |
Development | 26 | 49 |
Hormone metabolism | 30 | 33 |
Secondary metabolism | 28 | 20 |
Nitrogen and sulphur metabolism | 9 | 26 |
Lipid metabolism | 16 | 17 |
Cell wall metabolism | 12 | 21 |
Cell cycle and division | 0 | 32 |
Redox processes | 17 | 10 |
Unknown function | 315 | 381 |
Functional categories | Number of genes | |
Induced | Repressed | |
Protein metabolism | 95 | 200 |
Transcription factors | 94 | 151 |
Signalling | 26 | 135 |
Stress response | 38 | 68 |
Photosynthesis, (photo)respiration and carbon metabolism | 34 | 70 |
Transport | 30 | 68 |
DNA synthesis and repair | 5 | 74 |
Development | 26 | 49 |
Hormone metabolism | 30 | 33 |
Secondary metabolism | 28 | 20 |
Nitrogen and sulphur metabolism | 9 | 26 |
Lipid metabolism | 16 | 17 |
Cell wall metabolism | 12 | 21 |
Cell cycle and division | 0 | 32 |
Redox processes | 17 | 10 |
Unknown function | 315 | 381 |
The number of transcripts that were significantly induced or repressed in leaves 2 d and 4 d after transfer from high CO2 growth conditions to air are listed according to Functional categories.

Transcripts encoding thylakoid electron transport chain components that were differentially expressed 2 d and 4 d after transfer from growth at high CO2 to air. Transcripts with P-values <0.05 and fold changes (log2) of >0.5 and less than –0.5 are shown. Grey bars, 2 d after transfer; white bars, 4 d after transfer.
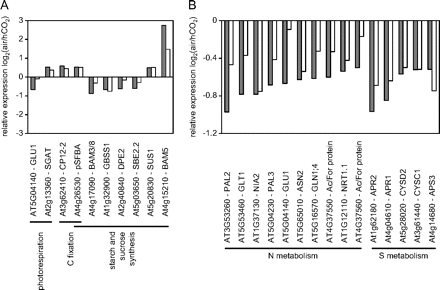
Transcripts encoding proteins associated with photorespiration, carbon fixation, and sucrose and starch synthesis that were differentially expressed 2 d and 4 d after transfer from growth at high CO2 to air. Transcripts with P-values <0.05 and fold changes (log2) of >0.5 and less than –0.5 are shown. Grey bars, 2 d after transfer; white bars, 4 d after transfer. A, carbon metabolism; B, nitrogen and sulphur metabolism.
Photorespiration increases transcripts encoding cyclic electron flow components
The transcriptome comparisons of A. thaliana leaves 2 d and 4 d after the transfer from high CO2 growth conditions showed that transcripts encoding the major PSII or PSI components were unaffected by photorespiration (Fig. 4). However, transcripts encoding the PSII reaction centre proteins PsbN and PsbI and LHCB4.3, were increased (Fig. 4). In contrast, LHCB2.4, encoding another LHCII protein, was repressed on transfer to air (Fig. 4). The location of the proteins encoded by PsbN, PsbI, LHCB2.4, and LHCB4.3 within the PET chain is indicated in Fig. 6. Other transcripts that were decreased 2 d and 4 d after the transfer to air were CH1, a chlorophyllide a oxygenase that converts chlorophyllide a to chlorophyllide b, and HCF107, which is required for the translation of three PSII subunit genes PsbB, PsbT, and PsbH (Fig. 4). Two cytb6f complex genes (PETB and PETD) and transcripts encoding violaxanthin de-epoxidase (VDE1), which is required for zeaxanthin synthesis and therefore involved in NPQ regulation, were also decreased 2 d and 4 d after the transfer to air (Fig. 6).
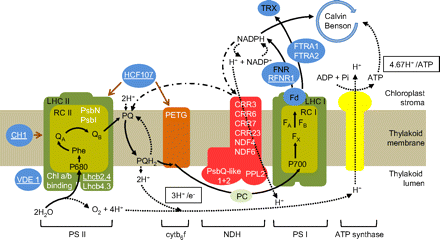
Simplified scheme for the thylakoid electron transport chain showing the location of proteins encoded by genes whose expression is altered following the transfer from high CO2 to air. White lettering denotes transcripts with P-values <0.05 and fold changes (log2) of >0.5 and less than –0.5. Letters that are underlined indicate that the abundance of transcripts was lower in air relative to high CO2, while the absence of underlining indicates transcripts whose abundance was higher in air than in high CO2. Gene lists for photosynthesis light reactions were assembled from metabolic and signalling pathways illustrated by the KEGG PATHWAY Database (http://www.genome.jp/kegg/pathway.html), the Arabidopsis Information Resource (TAIR; http://www.arabidopsis.org/), and the study by Bilgin et al. (2010). (This figure is available in colour at JXB online.)
As discussed above, a decrease in atmospheric CO2 availability requires a change in the generation of ATP relative to NADPH by the PET chain in order to accommodate the alterations in chloroplast metabolism associated with enhanced flux through the photorespiration pathways. The higher demand for ATP relative to NADPH in photorespiratory conditions (Table 1) can be met by several mechanisms, such as cyclic or pseudocyclic electron transport. One of the most striking features of the transcriptome signature of Arabidopsis leaves photosynthesizing in air compared with high CO2 (Queval et al., 2012) is the enhanced abundance of transcripts encoding components involved in cyclic electron flow around PSI (Figs 4, 6). Of the genes encoding photosynthetic components that were induced after transfer to photorespiratory conditions (Fig. 4), four are involved in chlororespiration (CRR3, CRR6, CRR7, and CRR23), two are NDH-dependent cyclic electron flow genes (NDF4 and NDF6), two are PsbQ-like proteins, while PPL2 encodes a PsbP-like protein. The location of proteins encoded by these genes within the PET chain is indicated in Fig. 6. The enhanced abundance of transcripts encoding components of the NDH complex and associated cyclic electron flow pathways (Suorsa et al., 2009) suggests that photosynthetic control of gene expression operates to enhance the expression of cyclic electron flow to meet the increased ATP/NADPH demand of photorespiration. Moreover, the repression of VDE1 might suggest that photosynthetic control of gene expression operates to decrease NPQ in the face of the enhanced demand for protons to make ATP (as illustrated in Fig. 6).
Photorespiration decreases transcripts associated with starch synthesis and nitrogen and sulphur metabolism
The second striking feature of the transcriptome signatures of Arabidopsis leaves in air compared with high CO2 is the absence of large changes in transcripts encoding enzymes of carbon fixation or photorespiration (Queval et al., 2012). However, transcripts encoding two proteins involved in photosynthetic carbon metabolism were increased in expression in air relative to high CO2 (Fig. 5). These are pSFBA, which encodes a putative aldolase superfamily protein, and CP12-2, which encodes a redox-regulated scaffold protein that forms a complex with GAPDH and phosphoribulokinase (Marri et al., 2005). Only six genes encoding proteins involved in starch and sucrose synthesis (Fig. 5) were changed in expression 2 d and 4 d after the transfer from high CO2 to air, and these are mapped onto the metabolic scheme shown in Fig. 7. Transcripts encoding two enzymes of starch synthesis, GBSS1, which encodes a granule-bound starch synthase, and SBE2.2, encoding a starch branching enzyme, were decreased in abundance 2 d and 4 d after transfer to air (Fig. 5). In contrast, a β-amylase gene (BAM5), which is involved in starch degradation, and SUS1 (sucrose synthase 1) were higher under photorespiratory conditions (Fig. 5). These results are consistent with decreased starch production when CO2 availability is low and photorespiration is increased (Fig. 8).
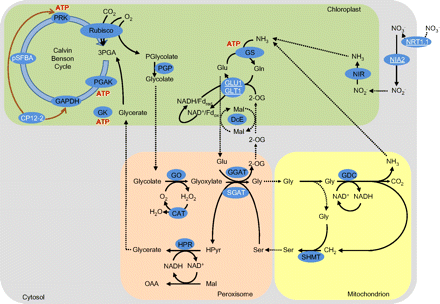
Simplified scheme showing the photosynthetic carbon reduction (Calvin–Benson) cycle and the photosynthetic carbon oxidation cycle and associated ammonia re-assimilation pathways. The figure indicates the location of proteins encoded by genes whose expression is altered following the transfer from high CO2 to air. White lettering denotes transcripts with P-values <0.05 and fold changes (log2) of >0.5 and less than –0.5. Letters that are underlined indicate that the abundance of transcripts was lower in air relative to high CO2, while the absence of underlining indicates transcripts whose abundance was higher in air than in high CO2. Chloroplast reactions that use ATP are indicated, as are reactions using NADPH or other reductants such as ferredoxin. Gene lists for carbon fixation, nitrogen assimilation and photorespiration, were assembled as described in Fig. 6. (This figure is available in colour at JXB online.)

Depiction of the pathways of sucrose synthesis in the cytosol and starch synthesis in the chloroplasts showing the location of proteins encoded by genes whose expression is altered following the transfer from high CO2 to air. White lettering denotes transcripts with P-values <0.05 and fold changes (log2) of >0.5 and less than –0.5. Letters that are underlined indicate that the abundance of transcripts was lower in air relative to high CO2, while the absence of underlining indicates transcripts whose abundance was higher in air than in high CO2. Gene lists for starch and sucrose metabolism were assembled as described in Fig. 6. (This figure is available in colour at JXB online.)
Transcripts encoding proteins involved in nitrogen assimilation that were repressed under photorespiratory conditions in Arabidopsis leaves include NRT1.1, NIA2, GLN1:4, GLT1, and GLU1 (Fig. 5, mapped onto Fig. 7). Transcripts (ASN2, PAL2, and PAL3) encoding proteins involved in amino acid metabolism were also decreased under photorespiratory conditions (Fig. 5). Similarly, APS3, APR1, APR2, CYSC1, and CYSD2 transcripts, which encode proteins associated with sulphur assimilation, were decreased in expression under conditions of enhanced photorespiration (Fig. 5). These data are consistent with the findings of other studies regarding the higher requirement for nitrogen and sulphur metabolites in plants grown with CO2 enrichment (Fig. 7).
Photosynthetic control of transcription factors
A surprisingly large number (245) of transcription factors were differentially expressed in response to photorespiration (Table 2). Transcripts encoding the most photorespiration-repressed and photorespiration-induced transcription factors are shown in Fig. 9. The MAR binding filament-like protein 1 (MFP1), which is associated with the formation of thylakoids and nucleoids (Jeong et al., 2003), was decreased in expression under photorespiratory conditions (Fig. 9). Interestingly, of the photorespiration-induced transcription factors, eight belong to the dehydration-responsive element-binding (DREB) subfamily. This finding is consistent with the higher level of stomatal opening, transpiration, and water transport that accompanies the transfer of C3 leaves from high CO2 growth conditions to air. None of the eight photorespiration-induced DREB transcription factors has yet been ascribed roles in the regulation of photosynthetic gene expression.
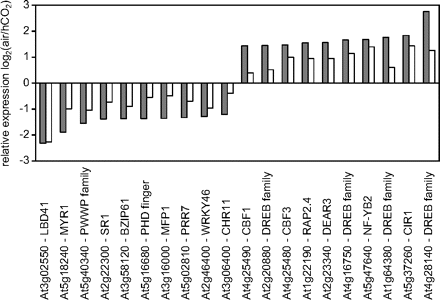
Transcripts encoding the 10 most repressed or induced transcription factors 2 d and 4 d after transfer from growth at high CO2 to air. Grey bars, 2 d after transfer; white bars, 4 d after transfer.
The apetala2.4 (RAP2.4) transcription factor has been shown to be induced by cold, drought, and osmotic stress and may have a role in water homeostasis by controlling the expression of aquaporins (Rae et al., 2011). C-repeat binding factors 1 and 3 (CBF1 and CBF3) are involved in cold acclimation and they are regulated by a core component of the circadian clock (Dong et al., 2011). Other transcription factors that were altered in expression following the transfer from high CO2 growth conditions to air (Fig. 9) are also involved in circadian regulation. These changes are consistent with recently described interactions between CO2 and daylength in the control of gene expression (Queval et al., 2012). Transcripts encoding the pseudo-response regulator 7 (PRR7) that is an element involved in the feedback loop controlling circadian rhythms (Nakamichi et al., 2010) are decreased in abundance following the transfer to air (Fig. 9). In contrast, circadian 1 (CIR1) is up-regulated by photorespiratory conditions. The expression of CIR1 follows a circadian rhythm, and perturbations in its expression affect flowering time and the circadian regulation of several genes, including Lhcb (Zhang et al., 2007). The MYB-related protein 1 (MYR1) gene is a repressor of flowering (Zhao et al., 2011) and was repressed after transfer to air (Fig. 9). Conversely, transcripts encoding the nuclear factor YB2 (NF-YB2), which promotes flowering (Kumimoto et al., 2008), were increased in abundance following the transfer to photorespiratory conditions. Transcripts encoding the LOB domain 41 (LBD41) transcription factor, which is responsive to the oxygen level, were greatly decreased in abundance following the transfer to photorespiratory conditions (Fig. 9). Hypoxia induces the expression of LBD41; this transcription factor activates the promoter of an anaerobic gene [non-symbiotic haemoglobin 1 (HB1); Licausi et al., 2011]. Since the ambient oxygen concentration was maintained constant at air levels in all conditions, it is of note that the expression of LBD41 is highly sensitive to the transfer from non-photorespiratory to photorespiratory conditions. Similarly, the absence of detectable changes in the abundance of transcription factor-encoding genes such as ZAT 10, ANAC036, ANAC090, ORA47, and ERF104 that are associated with changes in redox buffering capacity and H2O2 accumulation (Kerchev et al., 2011) suggests that the photosynthetic control of gene expression that accompanies the transfer from non-photorespiratory to photorespiratory conditions does not involve large changes in ROS or associated signalling pathways.
Conclusions and perspectives
Photosynthetic control operates at multiple levels in order to balance energy supply and energy demand and optimize photosynthetic efficiency, while minimizing potentially harmful back reactions. This balancing act not only involves matching output (assimilated carbon) to input (light and CO2) within a fluctuating environment (temperature, water status, and other potential stress factors) but it also requires the production of ATP and reductant at appropriate ratios. These ratios are responsive to metabolic factors, requiring flexibility in the relative rates of production. This flexibility is achieved through rapid, short-term modifications in post-translational controls and longer term regulation through effects on gene expression. The classical example of photosynthetic control of gene expression is the altered expression of genes that encode the core apoproteins of the photosystems that accompanies state transitions. It is rarely applied to transcriptome changes associated with varying metabolic demands for ATP and reductant, such as occur when the rate of photorespiration is varied relative to photosynthesis. The evidence presented here demonstrates that appropriate transcriptional responses are triggered in A. thaliana leaves alongside the rapid short-term adjustments in the photosynthetic control of electron transport flux in the days immediately following the transfer to air from high CO2 growth conditions. Moreover, transcripts associated with cyclic electron transport and NPQ respond in a way that is consistent with the expected alterations in supply and demand of ATP relative to reductant.
The changes in transcriptome signatures that accompany alterations in atmospheric CO2 availability are similar in different C3 species, with the most impact on genes involved in signalling and protein turnover (Ainsworth et al., 2006; Fukayama et al., 2009; Leakey et al., 2009b; Queval et al., 2012). CO2 enrichment also has an impact on the expression of genes encoding proteins involved in photosynthesis, such as light reaction components that tend to be decreased under CO2 enrichment (Li et al., 2008) and enzymes of carbondrate metabolism such as starch synthesis that are increased under these conditions (Ainsworth et al., 2006; Fukayama et al., 2009; Leakey et al., 2009b). The change in the transcription patterns of cyclic electron transport genes in A. thaliana points to an important role for cyclic pathways in adjusting ATP/reductant requirements. Thus transient changes in photorespiration that may occur, for example, in response to changes in stomatal conductance could influence the expression of genes encoding cyclic pathway proteins.
C4 grasses consistently exhibit lower maximum stomatal conductance to water than C3 grasses, together with shifts towards smaller stomata at a given density (Taylor et al., 2012). Carbonic anhydrases have been suggested to function early in the CO2 signalling pathway that controls stomatal movement and hence gas exchange between plants and the atmosphere (Hu et al., 2010). The response of specific photosynthesis genes to changes in CO2 concentration is accompanied by modified expression of specific transcription factors. As previously discussed (Queval et al., 2012), the finding that many of the most highly expressed transcription factors that were differentially expressed 2 d and 4 d after the transfer from high CO2 to air (Fig. 9) encode DREB family members is consistent with altered water relations resulting from increased stomatal opening in air relative to high CO2. Because patterns of CO2-regulated stomatal closure (and hence associated signalling) are similar in C3 and C4 plants, key questions remain concerning why C3 and C4 leaves do not show similar gene expression changes related to altered leaf water status as atmospheric CO2 availability is changed (Leakey et al., 2006, 2009a, b; Prins et al., 2011; Queval et al., 2012). A major difference between the two photosynthetic groups is the low level of photorespiration in C4 plants and the absence of any effects of high CO2 on photosynthesis in these species. One possibility is that the altered expression of transcription factors such as the DREB family members observed in the C3 species, A. thaliana, following the transfer to air from high CO2, are related directly to the onset of photorespiration and related photosynthetic control of electron transport rather than plant water status per se. Thus, changes associated with photorespiration and PET could be a proxy sensor for water status in C3 plants, or at least act as a reinforcing signal that tones the response to changes in this status. This hypothesis requires further study to elucidate relationships of cause and effect.
Considerable literature evidence supports the view that photosynthetic control is embedded not only within the whole cell physiology of the leaf but also in genome biology and control. This is therefore an important point to consider when identifying potential targets for improving photosynthesis through selection or genetic modification. Models describing how metabolism and electron (and proton) transport activities interact are also an important tool in better understanding the integration of metabolism with electron transport and ATP synthesis (e.g. Yin et al., 2006). They can be used to estimate, based on parameters such as the H+/e– and H+/ATP ratios, the extent to which linear electron transport alone could meet the demands of metabolism, and what activities of other electron transport processes could compensate for a shortfall in ATP (Noctor and Foyer, 1998). They can thus establish some boundary conditions for chloroplast function. These models cannot, however, replace a direct experimental analysis of the regulation of electron transport in relation to metabolism.
Abbreviations
- A
assimilation
- ABA
abscisic acid
- 1chl*
singlet excited state of chlorophyll a
- Ci
CO2 concentration in the substomatal cavity
- CRR
chlororespiratory reduction
- CSK
chloroplast sensor kinase
- cytb6f
cytochrome b6/f complex
- FACE
free-air concentration enrichment
- Fd
ferredoxin
- FNR
ferredoxin-NADP+ oxidoreductase
- FQR
ferredoxin quinone oxidoreductase
- GAPDH
glyceraldehyde-3-phosphate dehydrogenase
- H2O2
hydrogen peroxide
- LHC
light-harvesting complex
- MDH
malate dehydrogenase
- NDH
NAD(P)H dehydrogenase
- NPQ
non-photochemical quenching of chlorophyll fluorescence
- 1O2
singlet oxygen
- O2·–
superoxide
- PAR
photosynthetically active radiation
- PEP
plastid-encoded RNA polymerase
- PET
photosynthetic electron transport
- PGA
3-phosphoglyceric acid
- pmf
proton motive force
- PQ
plastoquinone
- PQH2
plastoquinol
- PS
photosystem
- QA
primary stable electron acceptor of photosystem II
- ROS
reactive oxygen species
- RuBP
ribulose-1,5-bisphosphate
- Rubisco
ribulose-1,5-bisphosphate carboxylase/oxygenase
- TRX
thioredoxin
- ΔμH+
transthylakoid proton potential
- ΔpH
transthylakoid pH gradient
- ΦPSI
quantum yield for electron transport by photosystem I
- ΦPSII
quantum yield for electron transport by photosystem II
Comments