-
PDF
- Split View
-
Views
-
Cite
Cite
Agnieszka Katarzyna Banaś, Chhavi Aggarwal, Justyna Łabuz, Olga Sztatelman, Halina Gabryś, Blue light signalling in chloroplast movements, Journal of Experimental Botany, Volume 63, Issue 4, February 2012, Pages 1559–1574, https://doi.org/10.1093/jxb/err429
- Share Icon Share
Abstract
Chloroplast movements are among the mechanisms allowing plants to cope with changes in their environment. Chloroplasts accumulate at illuminated cell areas under weak light while they avoid areas exposed to strong light. These directional responses may be controlled by blue and/or red light, depending on the plant group. In terrestrial angiosperms only the blue light perceived by phototropins is active. The last decade has seen a rapid development of studies on the mechanism of directional chloroplast movements, which started with an identification of the photoreceptors. A forward genetic approach has been used to identify the components which control chloroplast movements. This review summarizes the current state of research into the signalling pathways which lead to chloroplast responses. First, the molecular properties of phototropins are presented, followed by a characterization both of proteins which are active downstream of phototropins and of secondary messengers. Finally, cross-talk between light signalling involved in chloroplast movements and other signalling pathways is discussed.
Introduction
A general feature of light-controlled chloroplast relocation is their accumulation in weakly illuminated regions of the cell and avoidance of regions exposed to strong light. This distribution pattern helps to maximize light harvesting under energy-limiting conditions (Zurzycki, 1955; Takemiya et al., 2005), while it acts as a form of protection from excess energy in strong light (Park et al., 1996; Kasahara et al., 2002a; Sztatelman et al., 2010). The positional movements of chloroplasts happen only in an illuminated cell. The positioning is brought about in various ways depending on the plant group.
Only blue light (BL) induces the directional movement of chloroplasts in the mesophyll of terrestrial angiosperms. Arabidopsis thaliana is an undisputable leader among model plants belonging to this group. Other model species investigated include Nicotiana sp., Spinacia oleracea, and Tradescantia albiflora. In the dark, chloroplasts have stationary positions and their distribution pattern depends on the light intensity during growth, as shown in Arabidopsis (Trojan and Gabryś, 1996). In weak BL, chloroplasts redistribute to the periclinal walls, whereas in strong BL they move towards the anticlinal walls of the illuminated cell. The relationship between chloroplast distribution and the level of light transmittance through the tissue is often used to quantify chloroplast movements using photometry (Walczak and Gabryś, 1980; DeBlasio et al., 2003; Berg et al., 2006). Both types of chloroplast response measured as transmission changes attain stationary levels after ∼1.5 h, with the avoidance response starting and proceeding faster than the accumulation response. The light signal is perceived by phototropins, BL receptors associated with the plasma membrane (Jarillo et al., 2001; Kagawa et al., 2001; Sakai et al., 2001). Like other plant organelles, chloroplasts move along actin tracks in terrestrial angiosperms. The nature of the involvement of the actin cytoskeleton has not been resolved and the available data are rather controversial.
In model organisms among water angiosperms, the directional redistribution of chloroplasts depends mutually on cytoplasmic streaming. The representative chloroplast behaviour has been studied in detail in Vallisneria sp. (Izutani et al., 1990; Dong et al., 1996, 1998; Sakurai et al., 2005). The organelles, stationary in the dark, are set in motion together with the cytoplasmic matrix by continuous weak light, and they migrate to the periclinal side of the cell. The migration proceeds slowly to reach the full accumulation response after several hours. Red and blue spectral regions stimulate the accumulation response, with an approximately triple quantum efficiency of red light (RL) as compared with BL. It has been suggested that the PFR form of phytochrome and photosynthesis cooperatively regulate the accumulation response (Dong et al., 1996, 1998). A very special rearrangement of the actin cytoskeleton accompanies the accumulation response. A characteristic honeycomb formation becomes visible upon irradiation with RL, with a concomitant decline in the motility of chloroplasts, which appear inside the cavities of the honeycomb structure. Accordingly, the accumulation of chloroplasts at the periclinal wall of Vallisneria has been interpreted as the trapping of the flowing chloroplasts in a unique structure built of light-reorganized actin (Dong et al., 1996, 1998). Strong light induces a much faster avoidance response which directs the chloroplasts to the anticlinal sides within tens of minutes. This response is activated almost exclusively by BL (Izutani et al., 1990). Strong BL, presumably absorbed by a blue-specific photoreceptor, causes a fast reconstruction of F-actin into an ordinary network of linearly arranged bundles. Chloroplasts regain mobility, and, after a short phase of random short-range activity, they start to move unidirectionally along the actin tracks (Sakai and Takagi, 2005). Various elements of this mechanism can be detected in the light-mediated chloroplast positioning of cryptogam plants (for a review, see Wada et al., 2003).
In all genera tested, phototropins have turned out to be responsible for controlling the BL-mediated chloroplast responses. Another class of BL photoreceptors, cryptochromes, is not involved in chloroplast relocation (Ohgishi et al., 2004).
Besides phototropins, other photoreceptors (RL sensors) also control chloroplast relocation in several cryptogams. Most RL responses show red/far-red reversibility, indicating phytochrome involvement (for a review, see Haupt, 1999). In the case of Adiantum capillus-veneris, a chimeric photoreceptor neochrome (a phytochrome chromophore-binding domain attached to N-terminus of full-length phototropin) mediates RL-induced phototropism and chloroplast movements (Kawai et al., 2003). Neochrome genes have also been found in the green algae Mougeotia, but the involvement of this chimeric photoreceptor in the light-regulated rotation of its chloroplast has not been demonstrated (Suetsugu et al., 2005b). Each Mougeotia cell contains a single ribbon-shaped chloroplast which rotates to expose its face to weak light or its edge to strong light. Whereas the weak light-induced rotation can be mediated either by a phytochrome or by a BL photoreceptor (a phototropin?), the strong light response requires cooperation of the two systems (see Haupt and Scheuerlein, 1990; Haupt, 1999, and references therein). A time-resolved excitation of both systems has shown that the interaction involves intermediates downstream of the photoreceptors (Gabryś et al., 1985, 1988).
In terrestrial angiosperms, phytochromes seem to play only a modulatory role in chloroplast movements. While in Arabidopsis plants lacking phytochrome A or B the avoidance response is enhanced, an overexpression of one of these photoreceptors enhances the accumulation response (DeBlasio et al., 2003). Phytochrome B has been shown to attenuate the strong light avoidance response in concert with both phototropins (Luesse et al., 2010).
Blue light photoreceptors controlling chloroplast responses
Phototropins are activated upon absorption of BL and UV-A light (Christie, 2007). Recently, evidence for phototropin-mediated physiological responses elicited by UV-C has also been found (Magerøy et al., 2010). Two phototropins have been identified in Arabidopsis: phot1 and phot2. Both control chloroplast accumulation, but only phot2 controls chloroplast avoidance (Sakai et al., 2001). Phot2 alone is also responsible for the dark positioning of chloroplasts in palisade mesophyll cells (Suetsugu et al., 2005a) and the positioning of the nucleus (Iwabuchi et al., 2007). Only phot1 mediates the rapid inhibition of hypocotyl growth (Folta and Spalding, 2001) and the high intensity BL-induced destabilization of mRNAs (Folta and Kaufman, 2003). Both phototropins act together in regulating phototropism, stomatal opening, leaf positioning, and expansion, although phot1 exhibits higher light sensitivity than phot2 in some of the shared responses, namely chloroplast accumulation, phototropism (Sakai et al., 2001), and leaf positioning (Inoue et al., 2008b; de Carbonnel et al., 2010). The role that these photoreceptors play in disease resistance is also emerging (Jeong et al., 2010).
Phototropins consist of two parts: a photosensory N-terminal part comprising two LOV (Light, Oxygen, Voltage) domains, and a C-terminal kinase domain. While the two LOV domains of phototropins have high sequence identity, they have slightly different photochemical properties (Salomon et al., 2000). LOV2 is the main domain responsible for photoreceptor activity (Christie et al., 2002). LOV1 can attenuate the photoactivation of the kinase (Matsuoka and Tokutomi, 2005), modulate the light sensitivity of the photoreceptor (Kaiserli et al., 2009), or act as a dimerization site irrespective of light conditions in vitro (Salomon et al., 2004). However, phot1 fragments lacking LOV1 have been shown to undergo dimerization nonetheless (Kaiserli et al., 2009). A function of the phot1 LOV1 domain in arresting chloroplast accumulation under high light conditions has also been postulated (Kaiserli et al., 2009). Both LOV domains share a common tertiary fold with a hydrophobic pocket—a binding site of flavin mononucleotide chromophore (Crosson and Moffat, 2001). The binding is non-covalent in the dark, and, upon photoexcitation, an adduct between FMN and a conserved cysteine residue of the photoreceptor is formed. This adduct formation is a primary step in signal transduction (Salomon et al., 2000). It results in structural changes in the LOV2 domain and the Jα-helix located downstream (Crosson and Moffat, 2002; Harper et al., 2003), leading to a disruption of LOV2–kinase interaction and subsequently to the activation of the kinase (Matsuoka and Tokutomi, 2005). LOV2 has been proposed to act as a dark inhibitor of the kinase (Matsuoka and Tokutomi, 2005; Tokutomi et al., 2008).
The phototropin kinase is in the AGCVIII group of protein kinases (AGC-protein kinase A, cGMP-dependent protein kinase, and protein kinase C) (Galavan-Ampudia and Offringa, 2007). The first target of the kinase is phototropin itself (Christie et al., 1998). Phosphorylation sites for phot1 have been identified in four areas of the protein: at the N-terminus, in the hinge1 region between LOV1 and LOV2, in the kinase activation loop, and at the C-terminus (Salomon et al., 2003; Inoue et al., 2008a; Sullivan et al., 2008). Of those, only phosphorylation of the serines in the kinase activation loop is essential for phot1-mediated responses (Inoue et al., 2008a). However, substitution of serines with aspartates, which would mimic phosphorylation, produces a protein that still requires light for its activity, suggesting that phosphorylation alone is necessary but not sufficient for the signalling. Light-induced displacement of the LOV2 domain has been posited as another key factor of signal transduction (Inoue et al., 2008a). For phot2, the substitution of homologous serines with alanine led to impaired phot2-mediated responses, but did not remove them completely (Inoue et al., 2011).
The protein phosphatase 2A (PP2A) dephosphorylates phot2, but not phototropin 1 (Tseng and Briggs, 2010). A mutant of one of the PP2A regulatory subunits, rcn1-1, which shows reduced PP2A activity, exhibits enhanced phototropism and stomatal opening in plants in which only phot2 is active. The authors suggested that this is due to higher steady-state levels of phosphorylated (active) phot2. Indeed, plants expressing phot2 under a PHOT1 promoter showed a much higher level of phot2 protein in etiolated seedlings and a higher light sensitivity of phototropic response than wild-type (WT) plants, indicating that phototropism is affected by the amount of the photoreceptor (Inoue et al., 2011). In chloroplast avoidance movement, a dose dependency of the kinetics on the amount of phot2 was reported (Kagawa and Wada, 2004; Kimura and Kagawa, 2009). Strikingly, when a null allele was used as a reference, the dependence was far from linear, with velocities of chloroplast relocations changing only twice while the concentration of the protein changes 100 times. Determining chloroplast positions at different light intensities in lines expressing different levels of phot2 would help to understand the role of the absolute amount of (phosphorylated) photoreceptor in the movement mechanism.
So far, the only identified, physiologically relevant target of phototropin1 kinase other than phot1 itself is the auxin efflux transporter ATP-BINDING CASETTE B19 (ABCB19), which is involved in phototropism (Christie et al., 2011). Several other phototropin-interacting proteins have been identified to date, including NPH3/RPT2, 14-3-3 proteins, PKSs, and cytoskeleton components (summarized in Inoue et al., 2010). Recently, an interaction between phot2 and COP1 E3 ubiquitin ligase has been reported (Jeong et al., 2010). Interestingly, any given protein seems to be involved only in some of the phototropin-mediated responses, for example PKS2 plays a role in leaf positioning and flattening, but not in stomatal opening or chloroplast movement (de Carbonnel et al., 2010). Apart from actin, none of the phototropin-interacting proteins reported to date has been shown to be involved in chloroplast relocations.
Experiments on domain swapping between phot1 and phot2 provided insight on the determinants of the specificity of phototropins and their sensitivity (Aihara et al., 2008). Chimeric proteins consisting of the N-terminus of phot1 and the C-terminus of phot2 or vice versa were capable of activating all phototropin responses tested. Interestingly, both chimeric proteins were capable of triggering chloroplast avoidance upon strong BL illumination. This shows that there is no defined region of the protein responsible for determining the ability to elicit the switch between low-light chloroplast accumulation and high-light chloroplast avoidance responses. Additionally, phototropin from the green alga Chlamydomonas reinhardii triggers both an accumulation and an avoidance response when expressed in Arabidopsis (Onodera et al., 2005). Aihara et al. (2008) have suggested that the avoidance response is a basic function of phototropins which is suppressed in phot1. According to this point of view, genes coding for PHOT2-like photoreceptors are found in plants belonging to all groups throughout the plant kingdom, whereas PHOT1-like genes are restricted to spermatophytes (Galvan-Ampudia and Offringa, 2007), suggesting that phot1 can be more specialized in sensing low light, and, due to its enhanced light sensitivity, high-light responses are suppressed to avoid their activation in low-light conditions.
The N-terminal photosensory part mostly determines the light sensitivity of the photoreceptor, consistent with differences in the photochemical properties of isolated LOV domains from phot1 and phot2 (phot2 showing faster dark recovery; Kasahara et al., 2002b). A protein consisting of the N-terminal part of phot1 and the C-terminus of phot2 was shown to trigger chloroplast avoidance also in response to medium light (Aihara et al., 2008). The phot2 kinase domain alone expressed in Arabidopsis produced constitutive phot2 responses, including chloroplasts in profile position in darkness (Kong et al., 2007).
The dark positioning of the chloroplast is mediated by phot2 (Suetsugu et al., 2005a) and depends on light conditions during growth (Trojan and Gabryś, 1996). For this process, the N-terminal part of the phot2 is required, but the kinase can be derived from phot1 (Aihara et al., 2008).
Phototropins are associated with the plasma membrane in the dark, despite the fact that they lack any transmembrane domains. Upon photoexcitation, a fraction of phot1 moves to the cytosol (Sakamoto and Briggs, 2002) and a fraction of phot2 relocates to the Golgi apparatus (Kong et al., 2006), although the association of phot2 with the Golgi seems to be irrelevant to its function (Aihara et al., 2008). Similarly, a relocation of phot1 to cytosol was shown to occur in darkness for phot1 mutants that require light for activity, arguing against its role in signalling (Kaiserli et al., 2009). The kinase domain of phot2 is responsible for its membrane localization (Kong et al., 2007). It seems that phot1 is degraded in the active state. Firstly, BL exposure leads to a drastic lowering of phot1 levels (Sakamoto and Briggs, 2002; Kang et al., 2008). This effect can be partially attributed to the light-induced lowering of the mRNA level (Kang et al., 2008). Also, attempts to express constitutively active forms of phot1 in plants have been unsuccessful, despite the presence of transcripts (Kaiserli et al., 2009). Phot2, in contrast, is up-regulated by light at both the transcript and protein levels (Jarillo et al., 2001; Kagawa et al., 2001; Kang et al., 2008). This is further evidence that each phototropin plays a dominant role at a different range of light intensities.
Downstream components of the signalling cascade
Targets for blue light-generated signals
To date, two different types of proteins, myosin and actin, have been discussed as potential targets of BL-generated signals that set chloroplasts into directional motion. Myosin was proposed on the basis of investigations using polyclonal antibodies against plant myosin VIII and/or animal myosins, which reveal a BL-specific redistribution of the interacting protein(s) at the surface of Arabidopsis chloroplasts (Krzeszowiec and Gabryś, 2007). The arrangements of myosins observed in the mesophyll of WT Arabidopsis differed depending on the light intensity. Myosins were associated with chloroplast envelopes in the mesophyll irradiated with weak BL, while chloroplasts were almost myosin free in tissue irradiated with strong BL. The effect was absent in RL and in a phot2 mutant which lacked phot2 and, consequently, the avoidance response of chloroplasts. However, the redistribution of myosins was similar to that of the WT at the surface of chloroplasts in the phot1 mutant. Thus, myosins have been proposed as the final element of the BL signal transduction pathway starting with phot2 and leading to the avoidance response of chloroplasts (Fig. 1).
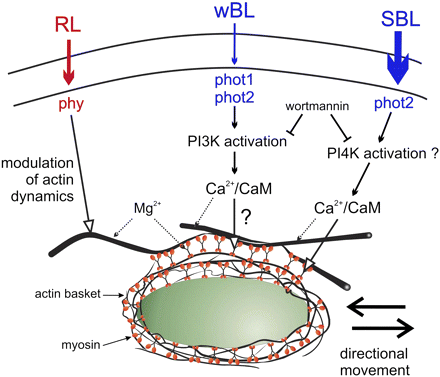
Schematic depiction of our working hypothesis showing myosin as a target of blue light signalling. Based on Grabalska and Malec (2004); Krzeszowiec and Gabryś (2007); Krzeszowiec et al. (2007); and Anielska-Mazur et al. (2009). The modulating role of phytochrome has been postulated on the basis of red-light-induced changes observed in the actin network architecture of the phot2 mutant (Krzeszowiec et al., 2007). CAM, calmodulin; PtdIns3K, PtdIns4K, phosphatidylinositol kinases; RL, red light; wBL, weak blue light; SBL, strong blue light; phy, phytochrome; phot, phototropin.
An entirely different target has been pointed out by Kadota et al. (2009). Using transgenic Arabidopsis plants expressing the green fluorescent protein (GFP)–mouse talin fusion protein, they identified short actin filaments (cp-actin, chloroplast actin) situated at the chloroplast periphery on the plasma membrane site. The quantity of cp-actin depended on the light intensity at the front and rear of chloroplasts. In strong light, the filaments transiently disappeared on irradiated chloroplasts which demonstrated increased motility in random directions. In phototropin mutants their dynamics differed according to the mutant type and light conditions: while no reorganization of cp-actin was seen in phot2 and phot1phot2 mutants lacking phot2, the reorganization was accelerated in the phot1 mutant (Ichikawa et al., 2011). Moreover, short actin filaments were not detected in the chup1 mutant, which shows a total immobility of chloroplasts (see next section).
The need for two different models of the mechanics of chloroplast motility is an obvious consequence of the different targets of BL-induced signals. If myosin is assumed to be the target, the mechanics would not be fundamentally different from those discussed for other organelles (for a review, see Krzeszowiec and Gabryś, 2011). On the other hand, a model entirely based on rearrangements of short actin filaments on the surface of chloroplasts would signify the operation of a unique, actin-based mechanism for chloroplast movements, different from those identified previously for other organelles (Suetsugu et al., 2010a).
Both concepts still require substantiation, even though the weight of evidence seems to favour the postulated role of cp-actin. The main support for myosin-based movement comes from experiments showing the BL-dependent redistribution of protein(s) interacting with anti-myosin antibodies. This mechanism is also given credence by the inhibition of BL-induced chloroplast responses by myosin blockers reported in previous works (Malec et al., 1996; Paves and Truve, 2007). However, this type of evidence cannot be considered conclusive, especially since the involvement of myosin in the movement mechanism did not gain support from experiments with the transient overexpression of headless myosin tails in Nicotiana benthamiana (Avisar et al., 2008). By this dominant negative inhibition approach, myosin XI-K was shown to play a major role in the movement of mitochondria, peroxisomes, and Golgi stacks in the epidermal cells of N. benthamiana. None of the tested tails of five XI myosins and the myosin VIII-1 affected light-induced chloroplast movements. This cannot be treated as final evidence against a myosin playing the role of a motor in chloroplast movements. Firstly, the involvement of another myosin type is still possible. Secondly, transient expression is known to take place chiefly in the epidermis, while undisturbed chloroplast responses have been demonstrated in the mesophyll tissue.
The evidence in support of the cp-actin model also has serious shortcomings. No BL-specific changes in actin organization were detected in Arabidopsis or in tobacco mesophyll cells using other markers (Krzeszowiec et al., 2007; Anielska-Mazur et al., 2009). Most experiments which demonstrate short actin filaments in action have been carried out on leaf petioles (Kadota et al., 2009), with the use of very high, non-physiological fluence rates (Ichikawa et al., 2011). Interestingly, the cp-actin described in petioles has not been found in mesophyll cells using Lifeact as an actin marker (Whippo et al., 2011). Thus, further work is necessary to identify the targets and the mechanism underlying the generation of directional motion.
Proteins mediating the signal transfer
Although light-induced chloroplast movements have been investigated for over a century, the elements acting downstream of photoreceptors are still not well characterized. The recognition of a signal transduction pathway has only recently gained momentum due to the discovery of many mutants with impaired movement responses.
In the last decade, two independent groups used a forward genetic approach to identify proteins located downstream of phototropins in the signalling pathway leading to chloroplast relocations. They used mutagenized populations of Arabidopsis for wide-screening experiments in which changes in light transmission through the leaf tissue were used to quantify chloroplast movements (Kagawa et al., 2001; DeBlasio et al., 2005). All mutants described below originate from these two screening approaches. The general position of the discovered proteins in the signalling pathway is summarized in Fig. 2.
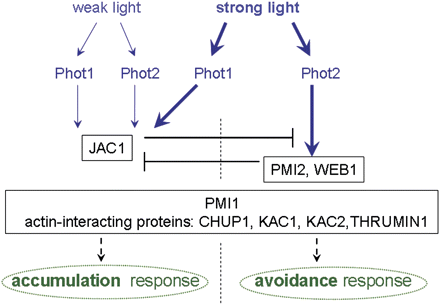
The proposed involvement of regulatory proteins in blue light-induced chloroplast movements. Both phototropins (phot1 and phot2) regulate the accumulation response while the avoidance response is controlled only by phot2. JAC1 is necessary for the accumulation response and acts as a switch between accumulation mediated by phot1 and avoidance mediated by phot2 in strong light (Kodama et al., 2010). The velocity of chloroplast movements under strong light is controlled by PMI2 and WEB1 (Luesse et al., 2006; Kodama et al., 2010). PMI1 (DeBlasio et al., 2005) and the actin-interacting proteins, CHUP1, KAC1, KAC2, and THRUMIN1, act non-specifically, influencing both chloroplast responses (Oikawa et al., 2003; Suetsugu et al., 2010b; Whippo et al., 2011).
Mutants in genes encoding actin-interacting proteins
The first mutant characterized as lacking chloroplast responses to light was chup1 (chloroplast unusual positioning1) (Oikawa et al., 2003). To date, CHUP1 is the only protein demonstrated to be indispensable for chloroplast movements and to be localized at the chloroplast outer membrane. Apart from showing no chloroplast responses to light, chup1 plants also have an unusual positioning of chloroplasts in the dark. Initially, chloroplasts were reported to be aggregated at the bottom of palisade and spongy mesophyll cells independent of light conditions, even when the leaves had been placed upside-down for 2 days (Oikawa et al., 2003). It has been proposed that chloroplasts are anchored to the plasma membrane via CHUP1 in darkness. After illumination they are released and can start moving. The domains responsible for binding to the plasma membrane and localization on the outer chloroplast envelope were identified and shown to be crucial for proper chloroplast positioning in darkness (Oikawa et al., 2008). Two additional domains located at the N-terminus seem to be responsible for movement responses: an F-actin-binding domain and a proline-rich domain. Whereas the F-actin-binding domain was shown to interact with F-actin and G-actin (Oikawa et al., 2003, 2008; Schmidt von Braun and Schleiff, 2008a), the proline-rich region interacts with profilin, an actin-binding protein (Schmidt von Braun and Schleiff, 2008a). CHUP1 is not involved per se in actin polymerization, but can act indirectly via profilin (Schmidt von Braun and Schleiff, 2008a). Moreover, even the C-terminal part of CHUP1, which does not interact directly with profilin, enhances the interaction between actin and profilin in vitro. Interestingly, a trunctated CHUP1 protein lacking the C-terminal zipper region did not complement the chup1 phenotype. Only recently has this region been shown to be involved in intramolecular interactions (Lehmann et al., 2011). As CHUP1 can form homodimers, such interactions were suggested to be essential for homodimer folding and, in consequence, for the proper exposure of domains responsible for actin and/or profilin binding. The organization of long actin filaments was normal in the mutant (Oikawa et al., 2003), but the short actin filaments surrounding the chloroplasts in petiole cells did not reorganize upon illumination (Kadota et al., 2009). A working model of CHUP1 functioning in chloroplast movements was proposed in which the protein is responsible for chloroplast anchoring to the plasma membrane; this docking is indispensable for proper chloroplast responses (Wada and Suetsugu, 2004). An important extension to the model was based on the identification of profilin as a protein interacting with CHUP1 (Schmidt von Braun and Schleiff, 2008a). Profilin was proposed as a target of phototropin signalling, while CHUP1 would act as a bridge between the actin cytoskeleton and the chloroplast, and as a modulator of the polymerization/depolymerization ratio of short actin filaments via profilin (Lehmann et al., 2011). The rate of actin polymerization, which is one order of magnitude faster than the velocity of chloroplast movements, was cited as an argument in favour of the direct function of CHUP1 in the movements (Schmidt von Braun and Schleiff, 2008b).
Besides chup1, mutants in genes encoding KINESIN LIKE PROTEINS FOR ACTIN BASED CHLOROPLAST MOVEMENT (KAC1 and KAC2) were also shown to have attenuated both types of chloroplast responses (Suetsugu et al., 2010b). KAC1 and KAC2 are expressed in root and in chloroplast-containing tissue, with the former gene having a significantly stronger expression (Suetsugu et al., 2010b). KAC proteins are specific to plants and localize in soluble and membrane fractions. Among other characteristics, they have a motor core domain at the N-terminus. Although they belong to the kinesin-14 family, some conserved amino acids in the motor domain are changed. As a result, KAC proteins neither interact with microtubules nor have ATPase activity. On the other hand, their C-terminal domains interact with actin in vitro. While in the kac1 mutant the accumulation response is attenuated, in kac2 both responses are comparable with those of WT plants. Thus, one could expect only the response to weak light to be impaired in the double mutant. However, the double kac1kac2 mutant had a clear phenotype showing no directional movements of chloroplasts in mesophyll cells under either weak or strong light. This has been attributed to KAC1 and KAC2 proteins acting redundantly but differing greatly in their expression levels (Suetsugu et al., 2010b). In addition to the lack of chloroplast responses, the dark positioning of chloroplasts was also impaired in the double mutant (in leaf petiole cells). As with chup1, chloroplasts were located at the cell bottom both in darkness and after illumination. This result points to the involvement of KACs, together with CHUP1, in chloroplast anchoring. Moreover, KAC proteins were shown to be involved in the formation of cp-actin filaments in leaf petioles in a light-dependent manner (Suetsugu et al., 2010b).
Another mutant in a gene encoding an actin-interacting protein was thrumin1, which had smaller amplitudes and velocities of chloroplast responses than the WT (Whippo et al., 2011). The C-terminal cysteine-rich domain of THRUMIN1 was demonstrated to be necessary for chloroplast movements. THRUMIN1 is localized to plasma membrane-associated microfilaments in a light- and phototropin-dependent manner. Its localization is controlled by both phototropins under exposure to strong light, as inferred from investigations performed on phototropin mutants. It would be interesting to see THRUMIN1 localization also in response to weak light. The anchoring of THRUMIN to the plasma membrane by S-myristoylation and/or N-palmitoylation is indispensable for its proper interaction with actin. The above authors have proposed that THRUMIN reorganizes actin filaments at the plasma membrane in a light-dependent manner and that such reorganization can influence CHUP1-mediated rearrangement of cp-actin around chloroplasts.
It is noteworthy that all mutants in proteins which interact with actin are defective in both chloroplast responses. Abundant evidence has been collected that each of these responses is controlled by a distinct mechanism (Walczak et al., 1984; Tlałka and Gabryś, 1993; Grabalska and Malec, 2004). Therefore, it is unlikely that any of these proteins is a specific element of a signal transduction pathway from one of the phototropins. Neither are any of these proteins responsible for directional transmission of the signal. Rather, they regulate the functioning of actin tracks by influencing actin dynamics.
Mutants in genes encoding proteins of unknown function
Chloroplast movements induced by both weak and strong BL are severely attenuated in the plastid movement impaired1 (pmi1) mutant, the first mutant described among those isolated from a pmi screen (DeBlasio et al., 2005). PMI1 is a protein whose function is as yet unknown, and which is highly conserved within the plant kingdom and expressed mainly in tissues containing chloroplasts. Among other domains, in silico analysis of the PMI1 protein predicts the presence of coiled-coil domains and of a C2 lipid-binding domain. The chloroplasts of pmi1 plants are evenly distributed along the anticlinal and periclinal walls in palisade cells, irrespective of whether they were acclimated to darkness, or illuminated with weak or strong BL. Nevertheless, very weak directional chloroplast movements have been reported. Light transmission through leaf tissue slightly decreased after illumination with weak BL, and slightly increased after illumination with strong BL. It is possible that the sensitivity of pmi1 is very similar to that of pmi2—that is, light intensities stronger than 100 μmol m−2 s−1 induce an avoidance response—but this has not yet been tested.
Mutants in two loci, pmi2 and web1 (weak chloroplast movements under blue light 1), have been characterized, with only avoidance responses impaired. PMI2 and WEB1 are expressed in stems, leaves, flowers, and roots (Luesse et al., 2006; Kodama et al., 2010). The presence of PMI2 and WEB1 transcripts in roots indicates that these proteins also function in processes other than chloroplast movements. PMI2 and WEB1 are plant-specific genes (Luesse et al., 2006; Kodama et al., 2010). It has been shown, using transient expression experiments with GFP-tagged proteins, that their products are localized in the cytoplasm (Luesse et al., 2006; Kodama et al., 2010). PMI2 and WEB1 have been classified as members of the plant-specific DUF 827 (DUF: domain of unknown function; Pfam PF05701) protein family (Kodama et al., 2010). In silico analysis predicts that PMI2 has long coiled-coil regions at the N-terminus, a putative nuclear localization signal (NLS), and a P-loop (a putative ATP-/GTP-binding motif A) at the C-terminus (Luesse et al., 2006). All pmi2 lines have been shown to have normal accumulation responses, but their responses to light intensities inducing avoidance in the WT (>10 μmol m−2 s−1 of BL) are severely attenuated. The differences in light-controlled chloroplast redistribution were most prominent in palisade cells (Luesse et al., 2006). The authors suggest that increasing the BL intensity above 100 μmol m−2 s−1 might induce a more robust avoidance movement. Actually, illumination with 1200 μmol m−2 s−1 of white light resulted in an increase in light transmission through the leaf tissue of the pmi2-3 mutant (Kodama et al., 2010). While the velocity of the accumulation response was almost unaffected in pmi2 plants (Luesse et al., 2006), the velocity of the avoidance response was very low. The WT chloroplasts reached a stationary position after 30 min illumination with 500 μmol m−2 s−1 of white light, whereas in pmi2-3 plants they were still relocating after 12 h (Kodama et al., 2010). Thus, strong light is perceived by the mutant, but the signal transfer is impaired. A very similar phenotype was found for web1 plants (Kodama et al., 2010). As with PMI2, WEB1 has coiled-coil domains and a predicted NLS domain. In both proteins the NLS domains seem to be non-functional, as the GFP-fused proteins have not been found to localize in the nucleus (Luesse et al., 2006; Kodama et al., 2010). A truncated PMI2 protein lacking the coiled-coil region did not complement chloroplast movements in the pmi2 mutant, indicating its key role in the movement response. Coiled-coil regions are known to be responsible for protein–protein interaction. As pmi2 and web1 have similar phenotypes and both proteins interact in the cytoplasm, it has been proposed that PMI2–WEB1 complexes function in chloroplast responses to light. However, no direct evidence has been presented so far to confirm that the formation of such complexes is necessary for chloroplast relocations (Kodama et al., 2010).
Only one Arabidopsis mutant shows a strongly impaired accumulation response while also having a normal avoidance response. This plant has a mutation in the J-domain protein required for chloroplast accumulation response 1 (jac1) gene (Suetsugu et al., 2005a). The gene is expressed in leaves and stems, but not in roots. JAC1 protein has a J-domain similar to auxilin at the C-terminus. All jac1 mutants tested showed no obvious directional movements after illumination with weak BL. They also exhibited a lower chloroplast velocity during the avoidance response and a much slower return of the chloroplasts to the dark position after strong light irradiation (Kodama et al., 2010). Moreover, the dark position of chloroplasts in jac1 palisade cells was shown to differ from that of the WT. Under experimental growth conditions, jac1 chloroplasts distributed randomly in the palisade cells instead of accumulating at the cell bottom, the distribution which has been described as characteristic of the WT (Suetsugu et al., 2005a). The experimental evidence provided does not fully support the postulated lack of accumulation response in jac1. The apparent insensitivity of the mutant’s chloroplasts to weak light might be caused by their different starting positions in the WT and jac1. Dose–response experiments using lower light intensities, and/or experiments with weak following strong light illumination would be required to confirm that jac1 chloroplasts indeed do not respond to weak light.
To sum up, pmi2 and web1 plants sense both weak and strong light, but are impaired in signal transfer, which results in slower directional chloroplast relocations under strong light. Interestingly, double jac1pmi2 and jac1web1 mutants have phenotypes almost identical to jac1 alone, namely a very weak accumulation response and a normal avoidance response (Kodama et al., 2010). Kodama et al. proposed a model of interactions between these proteins. In this model, JAC1 mediates the accumulation response and represses the signalling pathway for the avoidance response while the PMI2–WEB1 complex suppresses its activity in strong light conditions (see Fig. 2).
The auxilin-like J-domain appears to be crucial for a JAC1-dependent accumulation response. This domain has a highly conserved three-residue HPD (histidine, proline, and aspartic acid) motif. Based on the crystalline structure of JAC1, it has been postulated that, as with auxilin, this motif is responsible for interaction with HSC70 (Takano et al., 2010). HSC70 (70 kDa heat shock cognate) acts as a molecular chaperone. Its functioning in clathrin-dependent endocytosis is regulated by its interaction with auxilin. The JAC1 versions with mutations in the HPD motif did not rescue the chloroplast response to weak light in jac1 mutant plants (Takano et al., 2010). Thus, the authors proposed that clathrin-dependent trafficking of endomembrane vesicles is crucial to the accumulation response.
The fact that brefeldin A, an inhibitor of vesicle trafficking, did not inhibit light-induced chloroplast relocations (Kong et al., 2006) questions the involvement of endocytosis. Thus, the role of endocytosis in the signalling pathway leading to chloroplast relocation remains an open issue.
Secondary messengers
The role of calcium:
Calcium is a ubiquitous secondary messenger of cellular responses in both animal and plant systems (Helper, 2005). Prolonged elevation of Ca2+ results in irreversible damage to cells (Trump et al., 1984). Therefore, signalling mechanisms in cells are based on the generation of brief pulses of Ca2+ which enable calcium to act as a messenger. Studies in plants have revealed the significance of Ca2+ in various light responses, among them in those activated by BL and mediated by phototropins. Studies carried out with transgenic A. thaliana lines expressing cytoplasmic aequorin (a calcium-sensitive luminescent protein) helped significantly in understanding the relationship between the activation of phototropins and Ca2+ fluxes. The results suggest that each phototropin mediates a transient rise in the cytosolic Ca2+ concentration on perceiving BL, but the mechanisms of Ca2+ increases differ for both phototropins (Baum et al., 1999; Harada et al., 2003).
Baum et al. (1999) reported a phot1-mediated Ca2+ increase in de-etiolated seedlings of Arabidopsis (expressing cytosolic aequorin). Short BL pulses induced cytoplasmic Ca2+ transients that were strongly suppressed in the phot1 mutant but unaffected in cry1 and cry2 mutants. In addition, the overnight application of plasma membrane Ca2+ channel blockers (La3+, BAPTA, EGTA, and nifedipine) abolished these transients, thereby suggesting phot1 activation of plasma membrane Ca2+ channels which then allow Ca2+ influx inside the cell (Baum et al., 1999). Similar results were obtained using a microelectrode ion flux measurement (MIFE) technique to determine BL-induced Ca2+ flux near the surface (cotyledons and hypocotyls) of Arabidopsis seedlings (Babourina et al., 2002). BL irradiation generated similar Ca2+ fluxes in both the WT and the phot2 mutant but these fluxes were absent in phot1 and phot1phot2 mutants. Thus, the results indicated that in Arabidopsis seedlings, phot1 mediates Ca2+ uptake from the apoplast into the cytoplasm while phot2 does not participate in the effect. Subsequently, using the patch–clamp technique on A. thaliana mesophyll protoplasts, Stoelzle et al. (2003) showed BL-activated hyperpolarization-dependent calcium-permeable channels in the plasma membrane. The BL-induced inward Ca2+ currents were drastically reduced in the phot1 mutant and completely eliminated in the phot1phot2 double mutant, while no changes were observed with the cry1cry2 double mutant or in the presence of DCMU [3-(3,4-dichlorophenyl)-1,1-dimethylurea, a photosynthetic electron transfer inhibitor] (Stoelzle et al., 2003). This gave further credence to the idea that phot1-induced Ca2+ responses in mesophyll cells originate from a Ca2+ influx across the cell membrane. Concomitantly, using a pharmacological approach in Arabidopsis leaves (expressing cytosolic aequorin), Harada et al. (2003) suggested that phot1 and phot2 activate different mechanisms leading to an increase in Ca2+ inside the cell. Both phot1 and phot2 mediated a transient increase of cytoplasmic Ca2+, which lasted ∼50–70 s upon a BL pulse. While the application of inorganic Ca2+ channel blockers (CoCl2, LaCl2, and EGTA) and organic blockers (nifedipine) showed significant reduction of Ca2+ transients in the WT, phot1 and phot2 mutants, the use of phospholipase C (PLC) inhibitors (neomycin and U73122) decreased the transients only in the WT and the phot1 mutant. At the same time, no major changes were observed in a phot2 mutant (Harada et al., 2003). Thus, a basic model was postulated, stating that in Arabidopsis leaves both phot1 and phot2 can induce Ca2+ influx from the apoplast through plasma membrane Ca2+ channels, but phot2 might also trigger a release of Ca2+ from internal stores via PLC activation and a release of inositol-1,4,5 triphosphate [Ins(1,4,5)P3].
All the above findings strongly support the notion that phototropin activation results in changes in Ca2+ concentration inside the cell. However, they do not show any direct involvement of calcium in phototropin-mediated processes. Initial studies showing the direct participation of Ca2+ in BL-mediated chloroplast movements have been carried out on Lemna trisulca.Tlałka and Gabryś (1993) discussed the importance of the intracellular Ca2+ stores in the regulation of chloroplast movements in Lemna. A partial inhibition of chloroplast movements was observed after prolonged incubation (12 h) with EGTA, and similar effects were noted with the use of lanthanum (La3+). Moreover, ionophore A23187 applied together with EGTA for 1 h markedly hampered movements. The cell membrane is impermeable to EGTA, but lengthy incubation with a calcium ion chelator stimulates Ca2+ mobilization from internal stores, and its application along with ionophore depletes intracellular Ca2+ stores much more rapidly (Tlałka and Gabryś, 1993). Therefore, a major role for internal stores in providing Ca2+ during chloroplast relocations was proposed. Later data obtained by Tlałka and Fricker (1999) fully supported the above results. The inhibitors thapsigargin and caffeine which block the release of Ca2+ from internal stores, inhibited chloroplast movements within a few minutes of their application. On the other hand, EGTA, verapamil, and nifedipine showed only partial inhibition even after very long-term incubation (Tlałka and Fricker, 1999). These findings emphasize the importance of the release of Ca2+ from internal stores in managing BL-induced chloroplast movements in Lemna (Fig. 3A).
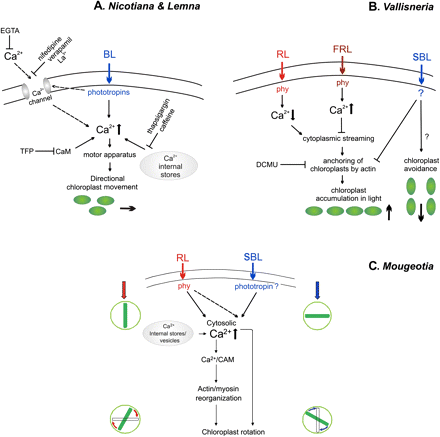
Graphic representation of concepts on the role of Ca2+ in chloroplast movements in various model plants, (A) The blue-sensitive angiosperms: Lemna trisulca (Tlałka and Gabryś, 1993; Tlałka and Fricker, 1999) and Nicotiana tabacum (Anielska-Mazur et al., 2009). (B) The red- and blue-sensitive water angiosperm Vallisneria (Takagi and Nagai, 1992; Sakai and Takagi, 2005). (C) The red- and blue-sensitive filamentous alga Mougeotia (Haupt, 1999). The L-shaped arrow indicates the signal transduction events following blue light receptor activation, of which the downstream elements have not been studied. BL, blue light; SBL, strong blue light; RL, red light; FRL, far red light; phy, phytochrome; TFP, trifluoperazine, a calmodulin (CaM) blocker.
The only study to focus on the role of calcium in chloroplast movements in higher land plants was performed on Nicotiana tabacum (Anielska-Mazur et al., 2009). Calcium applied exogenously did not influence chloroplast movements. Only when Ca2+ was added with an A23187 ionophore were chloroplast accumulation and avoidance movements impaired. This demonstrates that internal calcium stores are probably crucial to chloroplast relocations, since the ionophore applied exogenously along with Ca2+ perturbs the homeostasis of these ions in the cell. Short-term treatment with EGTA or TFP (trifluoperazine, a calmodulin blocker) eradicated both chloroplast responses (Fig. 3A). The most striking effect was observed when Ca2+ or Mg2+ were added after incubation with these compounds. Both ions partially restored chloroplast movements in the EGTA-treated tissues. The restoration of chloroplast movements by calcium ions after incubation with EGTA had also been shown previously for Adiantum (Kadota and Wada, 1992). Interestingly, in the case of TFP inhibition, the recovery effect of magnesium was twice as strong as that of calcium. An analogous effect of magnesium was reported previously for Lemna (Tlałka and Gabryś, 1993). These results also show that magnesium plays an important role in chloroplast movement. The effect of TFP suggests that calmodulin is involved in this signal transduction pathway, a result consistent with the formerly proposed role of calmodulin in the movement of Mougeotia chloroplast (Wagner et al., 1984).
Although no detailed model of the signalling mechanism has been established for cryptogams, a few reports suggest the importance of calcium during chloroplast movements. For instance, in Mougeotia, a dense row of calcium-rich vesicles was shown to be present at the chloroplast edges, and the depletion of these vesicles was directly correlated with a decline in chloroplast responses to light (Wagner and Klein, 1981). In a similar manner to Lemna, the application of plasma membrane Ca2+ channel blockers (nifedipine, diltiazem, ruthenium red, La3+, and Co2+) did not inhibit chloroplast movement in Mougeotia. This again suggests that Ca2+ from internal stores (vesicles) has a role to play in controlling the movements (Schönbohm et al., 1990) (Fig. 3C). In accordance with these results, Russ et al. (1991) observed an increase of cytosolic Ca2+ in Mougeotia upon pulse irradiation with UV-A (365 nm) and BL, independent of external calcium. Likewise, in another species, A. capillus-veneris, Sato et al. (2001) demonstrated that light-stimulated chloroplast movements were independent of external Ca2+ and unaffected by plasma membrane Ca2+ channel blockers (Gd3+ and La3+).
A unique mode of calcium fluxes and chloroplast movements was observed in an aquatic angiosperm Vallisneria gigantea (Fig. 3B) which shows RL and BL sensitivity (see Introduction). The cytoplasmic streaming activated by RL was regulated by a change in the intracellular Ca2+ concentration. RL induced the streaming, with a concomitant decrease in Ca2+ concentration in the cytoplasm, whereas far-red light counteracted the action of RL and was responsible for intracellular Ca2+ accumulation (Takagi and Nagai, 1983). In addition, the treatment of cells with a solution containing >10 μM Ca(NO3)2 stopped the cytoplasmic streaming (Takagi and Nagai, 1986). These fluxes of calcium in Vallisneria seemed to be based on the active transport of these ions through the cell membrane (Takagi and Nagai, 1988). Phytochrome was shown to be responsible for the regulation of cytoplasmic streaming together with photosynthesis which was postulated to maintain the energy supply for the calcium ion transporters (Takagi et al., 1990).
The above studies establish the importance of calcium ions in BL-induced chloroplast movements. The ions play a substantial role in maintaining the integrity of the cytoskeleton network and in the signalling pathway. The activation of phototropins allows an influx of Ca2+ inside the cell, with phot1 from the apoplast and phot2 from both the apoplast and internal stores (as reported with Arabidopsis, see above). However, reports from Lemna and lower plants point to intracellular calcium stores as playing a more important role than apoplastic calcium in BL-mediated chloroplast movements. This in turn suggests that phot1 and phot2 might be functionally interacting with each other in bringing about the optimized calcium response. In addition, microbeam irradiation induced localized chloroplast responses in mesophyll cells of Arabidopsis (Kagawa and Wada, 2000). Under strong BL, chloroplasts moved towards the irradiated area in the cell but did not enter it, while showing both accumulation and avoidance responses. This result may be interpreted in terms of specific Ca2+ signatures present in the adjacent areas which might determine the direction of chloroplast movements.
The role of phosphoinositides:
Phosphoinositide signaling in plants plays an important role in controlling many developmental processes and stress responses by influencing actin organization and vesicle trafficking (for a review, see Xue et al., 2009). In the classical pathway, phosphatidylinositol-4,5 bisphosphate [PtdIns(4,5)P2] serves as a substrate for the production of diacylglycerol (DAG) and Ins(1,4,5)P3 by PLC. Apart from PtdIns(4,5)P2, five different phosphoinositide species have been discovered in plants to date: PtdIns(4)P, PtdIns(3)P, PtdIns(5)P, PtdIns(3,4)P2, and PtdIns(3,5)P2. A set of phosphoinositide-metabolizing enzymes, kinases and phosphatases, operating at different positions of the inositol ring, controls the abundance of these molecules in the cell (Mueller-Roeber and Pical, 2002).
More and more links between phosphoinositide signalling and phototropin activity in plants have emerged recently. Four different phosphoinositide species, PtdIns(4,5)P2, PtdIns(4)P, PtdIns(3)P, and Ins(1,4,5)P3, have been shown to be involved in controlling stomatal movements (for a review, see Lee and Lee, 2008). In Arabidopsis, the inositol polyphosphate 5-phosphatase13 (5PTase13) acted downstream of phot1 in the rapid inhibition of hypocotyl growth and it showed antagonistic effects on BL calcium signalling mediated by this photoreceptor (Chen et al., 2008). Studies of calcium fluxes performed in Arabidopsis leaves pointed to the involvement of PLC in the signal transduction from phot2. PLC inhibitors suppressed the BL-induced increase in Ca2+ in the WT and phot1, but no inhibitory effect was observed in the phot2 mutant. Hence, it has been postulated that the phot2-generated release of calcium ions from internal stores is mediated via channels sensitive to phosphoinositol derivatives. On the other hand, it has also been suggested that the production of Ins(1,4,5)P3 may be controlled by phot2 via a direct modulation of PLC activity in the plasma membrane (Harada et al., 2003).
Evidence for the role of phosphoinositides in controlling chloroplast movement (Fig. 1) comes only from indirect studies using wortmannin (Grabalska and Malec, 2004; Anielska-Mazur et al., 2009). Wortmannin is a specific inhibitor of PtdIns 3-kinase at low concentrations. Wortmannin at 1 μM inhibited >95% of its activity in tobacco crude extracts (Matusoka et al., 1995). At higher concentrations (micromolar range) wortmannin was shown to inhibit type III PtdIns 4-kinases (Krinke et al., 2007). However, in animal systems, wortmannin became non-specific at higher concentrations and was capable of inhibiting other kinases as well (for a review, see Wipf and Halter, 2005).
A study on L. trisulca (Grabalska and Malec, 2004) demonstrated that chloroplast accumulation and avoidance movements are differently sensitive to this inhibitor. Under continuous BL, the chloroplast accumulation response was completely impaired by a low concentration (100 nM) of wortmannin, while the avoidance response remained unaffected. At 1 μM wortmannin, no chloroplast movement took place. These results suggest that a PtdIns 3-kinase may be involved in the chloroplast accumulation under weak light. Another, less specific target of wortmannin seems to play a leading role in controlling the avoidance movement under strong light. The results may imply that phosphoinositides themselves represent, or else act on, the factor which orients chloroplasts towards the accumulation position without influencing the motor apparatus, since the avoidance response still occurred at low concentrations of the inhibitor. Furthermore, 100 nM wortmannin arrested chloroplasts in the avoidance phase of the bi-phasic transient response generated by BL pulses of energy fluence >30 J. These findings suggest the existence of two different polarities which determine the direction of chloroplast movements either to the anticlinal or to the periclinal walls. A low concentration of wortmannin inhibited the phosphoinositides (particularly PtdIns 3-kinase) which could play a role in the formation of cell polarity for the accumulation phase, while a high concentration of wortmannin inhibited other non-specific targets, which may account for the polarity of the avoidance phase.
Work on N. tabacum has also demonstrated that the avoidance and accumulation responses are differently sensitive to wortmannin (Anielska-Mazur et al., 2009). At a 10 μM concentration, this inhibitor completely eliminated the accumulation response, while the avoidance response was reduced by half. Wortmannin was shown not to affect the organization of the actin cytoskeleton at this concentration. It is worth noting that both Ca2+ and Mg2+ added after wortmannin treatment restored chloroplast movements. This restoration was stronger for the avoidance response. Although it would be tempting to speculate that calcium and/or magnesium ions are responsible for defining the direction of movement, it has to be emphasized that they were delivered non-directionally. Hence, Ca2+ and/or Mg2+ seem to modulate some as yet undiscovered factor(s) which generate(s) the cell polarity required for a particular response, rather than generate that polarity themselves.
Cross-talk with other signalling pathways
The physiological function of directional chloroplast movements as a mechanism for the optimization of light capture in a changing environment was postulated over half a century ago. Under light-limiting conditions, dose–response curves of photosynthesis were shown to correlate with those for chloroplast rearrangements, demonstrating that the accumulation response helps plants to maximize photosynthesis (Zurzycki, 1955). This result was confirmed in long-term experiments using Arabidopsis phototropin mutants grown under low light conditions (Takemiya et al., 2005). The photoprotective function of the avoidance response was shown in comparative studies using plant species which either had or did not have light-directed chloroplast movements (Park et al., 1996). Those that did not were more susceptible to damage by excess light. Again, experiments using phototropin mutants confirmed the role of the avoidance response in coping with high light stress (Kasahara et al., 2002; Sztatelman et al., 2010).
Nevertheless, no direct feedback from photosynthesis in phototropin-regulated chloroplast redistribution has been found so far. Neither DCMU nor methylviologen (MV) affected movements in Arabidopsis (Ślesak and Gabryś, 1996). Moreover, DCMU was also ineffective in N. tabacum (Nauš et al., 2008). Thus, if any feedback regulation exists, light reactions seem not to be involved. Sugars produced in the dark phase seemed to be good candidates for this function as they can act as signalling molecules in plant cells (Rolland et al., 2006). Among other processes, they regulate photosynthesis via control of gene expression and/or enzyme activity. Two different experimental approaches were applied to test the role of sugars in chloroplast directional movements. In the first, detached Arabidopsis leaves were incubated on agar with sugars added (Banaś and Gabryś, 2007); in the second, Arabidopsis was grown in vitro on media supplemented with sugars (Eckstein et al., 2011). The sugar effect depended on the means of delivery, by diffusion as in the former model, or via the vascular system from roots as in the latter model. When Arabidopsis was grown in vitro with sugars added to the medium, the amplitude of the accumulation response slightly decreased as the concentration of glucose/sucrose increased, while the velocity remained unchanged (Eckstein et al., 2011). The opposite effect was found for the avoidance response. Both glucose and sucrose enhanced its amplitude but slowed down its kinetics. Much stronger effects were found after leaves were brought into direct contact with sugars. Sucrose and glucose inhibited both chloroplast responses in a time- and concentration-dependent manner (Banaś and Gabryś, 2007). However, this inhibition was not due to a disturbed actin cytoskeleton (Banaś et al., 2010). Studies with sugar analogues point to hexoses rather than disaccharides taking part in the sugar effect (Banaś and Gabryś, 2007). The involved signalling pathways remain uncertain. Although the amplitudes and velocities of both chloroplast responses were decreased by sugars, only the avoidance amplitude was shown to be controlled via a hexokinase-dependent signalling pathway (Banaś and Gabryś, 2007). Notably, overexpression of PHOT2 counteracted the sugar inhibition, suggesting that sugars may play an inhibitory role via down-regulation of PHOT2 expression. However, sucrose did not influence and glucose markedly increased the PHOT2 mRNA level in WT plants. The lack of correlation between the PHOT2 transcript level and the inhibitory effect of sugars implies that they act via modification of the component(s) of the phot2-mediated signal transduction pathway. The prolonged exposure necessary for the inhibitory effect of sugars indicates that it is a long-term effect which encompasses changes in gene expression. Thus, it seems that neither electron transport per se nor sugars are elements of fast feedback control of BL-induced chloroplast movements.
Interestingly, photosynthesis seems to be involved in the regulation of RL-controlled chloroplast movements. Photosynthesis inhibitors influenced chloroplast relocation in plants where they are activated by RL via phytochromes (Vallisneria) or neochromes (A. capillus-veneris). In Vallisneria, the RL-dependent reorganization of the actin cytoskeleton, necessary for trapping the organelles, was suppressed by DCMU. In consequence, chloroplasts continued their swimming instead of being immobilized (Dong et al., 1996). DCMU also inhibited directional chloroplast movements controlled by RL in Adiantum prothalia (Sugiyama and Kadota, 2011). This inhibition could be partially counteracted by sucrose or glucose. In addition to reversing the DCMU effect, both sugars enhanced not only light-induced chloroplast relocations, but also non-directional relocations to the periclinal walls in the dark (Sugiyama and Kadota, 2011). The authors conclude that the light phase of photosynthesis (photosynthetic electron transport) and photosynthesis products (sugars) promote the release of Adiantum chloroplasts from their dark position, but are not responsible for directional movements.
The biological significance of the inhibitory effect of sugars delivered by diffusion on BL-induced chloroplast movements remains uncertain (Banaś and Gabryś, 2007). Sugars may act as stress signals. Their levels in the tissue may be elevated by a disturbed source–sink balance or a pathogen attack (Rolland et al., 2006; Berger et al., 2007). Besides, sugars induce the expression of pathogen-related genes (Herbers and Sonnewald, 1998). Movement responses are energy consuming, and plants probably tend to direct energy into more vital processes under stress conditions.
Redox signals, another possible key player, have been tested only recently (Wen et al., 2008). Strong BL generates H2O2 at the plasma membrane and at the chloroplast surface. The amount of this reactive oxygen species (ROS) is significantly higher in phot2 and double phot1phot2 mutants lacking the avoidance response. Exogenously applied H2O2 at concentrations >0.1 mM enhanced the chloroplast response to strong light. ROS scavengers, catalase, ascorbic acid, and mannitol, inhibited this response when added just before the illumination. However, not only did mannitol not inhibit avoidance, it even promoted it in detached Arabidopsis leaves incubated for 2 d with this alcohol (Banaś and Gabryś, 2007). Morever, the absence of the inhibition of chloroplast movements by DCMU (Ślesak and Gabryś, 1996) speaks against a promoting role for H2O2 in strong light movement. A concentration of DCMU one order of magnitude lower than those which did not affect chloroplast relocation decreased the production of H2O2 by almost 50% (Wen et al., 2008). Further experiments are required to elucidate the role of redox signalling in chloroplast responses to light.
As mentioned above, an increase in H2O2 was particularly strong in mutants that lacked the avoidance response when exposed to strong light (Wen et al., 2008). If the inhibition of chloroplast movements by sugars resulted in an enhanced production of ROS, this might help a plant defend itself against attack by a pathogen. Increasing evidence supports the existence of cross-talk between light signalling and the plant defence system (for a review, see Karpiński et al., 2003; Kami et al., 2010). Phototropins have only recently been identified as potential actors in plant responses to pathogens. Phot2 was shown to mediate resistance to Turnip crinkle virus in a BL-dependent manner (Jeong et al., 2010). Both phototropins were demonstrated to co-immunoprecipitate with the RPS2 (Resistant to Pseudomonas Syringae 2) protein (Qi and Katagiri, 2009). However, mutations in BL photoreceptors did not alter resistance to that pathogen (Jeong et al., 2010). Neither are interactions between RPS2 and phototropins essential for chloroplast relocations, as rps2 mutants respond normally to both weak and strong light (AKB, unpublished results). Thus, the role of chloroplast movements in plant defence remains an open question.
Concluding remarks
The current state of research into the studies of signalling pathways which lead to chloroplast distribution can be compared with the situation faced by somebody who has bought a few jigsaw puzzles and had the pieces mixed up by naughty children. A great deal of work is still required to select the right pieces and arrange them into coherent images.
Financial support from EU funds within the framework of FP7, Marie Curie ITN, grant no. 215174 ‘Chloroplast Signals’ (COSI) is gratefully acknowledged. The Faculty of Biochemistry, Biophysics and Biotechnology of the Jagiellonian University is the beneficiary of structural funds from the European Union—grant no: POIG.02.01.00-12-064/08.
Comments