-
PDF
- Split View
-
Views
-
Cite
Cite
Tiziana Destro, Dinesh Prasad, Damiano Martignago, Ignacio Lliso Bernet, Anna Rita Trentin, Indu Kumari Renu, Massimo Ferretti, Antonio Masi, Compensatory expression and substrate inducibility of γ-glutamyl transferase GGT2 isoform in Arabidopsis thaliana, Journal of Experimental Botany, Volume 62, Issue 2, January 2011, Pages 805–814, https://doi.org/10.1093/jxb/erq316
- Share Icon Share
Abstract
γ-Glutamyl transferases (GGT; EC 2.3.2.2) are glutathione-degrading enzymes that are represented in Arabidopsis thaliana by a small gene family of four members. Two isoforms, GGT1 and GGT2, are apoplastic, sharing broad similarities in their amino acid sequences, but they are differently expressed in the tissues: GGT1 is expressed in roots, leaves, and siliques, while GGT2 was thought to be expressed only in siliques. It is demonstrated here that GGT2 is also expressed in wild-type roots, albeit in very small amounts. GGT2 expression is enhanced in ggt1 knockout mutants, suggesting a compensatory effect to restore GGT activity in the root apoplast. Supplementation with 100 μM glutathione (GSH) resulted in the up-regulation of GGT2 gene expression in wild-type and ggt1 knockout roots, and of GGT1 gene expression in wild-type roots. Glutathione recovery was hampered by the GGT inhibitor serine/borate, suggesting a major role for apoplastic GGTs in this process. These findings can explain the ability of ggt1 knockout mutants to retrieve exogenously added glutathione from the growth medium.
Introduction
Given its central role in cell homeostasis, glutathione (GSH) has received much attention from the scientific community, documented by numerous papers focusing on different aspects of GSH metabolism relating to plant nutrition and assimilation, plant development and cell cycle regulation, plant defence, adaptation to the environment, and redox control (May et al., 1998; Noctor et al., 1998; Vernoux et al., 2000; Beemster et al., 2003; Tausz et al., 2004; Mullineaux and Rausch, 2005; Meyer, 2008; Szalai et al., 2009; Foyer and Noctor, 2009). Many aspects of its metabolism have been fully elucidated in plants, and the biochemical steps leading to its synthesis have been described (Foyer and Noctor, 2001). In leaf cells, cytosol and chloroplast are a major source of GSH, which is transported to sink organs by phloem translocation (Herschbach and Rennenberg, 1995; Herschbach et al., 2000). The metabolic steps leading to xenobiotic detoxification through glutathione conjugation have also been described (Marrs, 1996; Dixon et al., 1998; Edwards et al., 2000).
The metabolic route relating to GSH degradation, which has been shown in animal systems to be fed by γ-glutamyl transferases (GGTs), has only recently received some attention in plant science. The initial assumption was that of a substantial difference between animal and plant organisms in terms of the subcellular localization, substrate affinity, and functions of GGT, but the description of its biochemical properties in plant tissues (Martin and Slovin, 2000) later pointed to broad similarities in GGTs from different organisms. An alternative pathway mediated by carboxypeptidase has also been demonstrated in Arabidopsis, which may account for greater GSH degradation (Ohkama-Ohtsu et al., 2008); this pathway is localized in the cytosol, however, and any conclusions are applicable to that compartment alone.
The significance of GGTs in plants has recently been addressed in several studies, in which Arabidopsis thaliana was used as a model (Storozhenko et al., 2002; Grzam et al., 2007; Martin et al., 2007; Ohkama-Otsu et al. 2007a, b). The Arabidopsis genome is known to carry four GGT isoforms, with specific subcellular and organ-level spatial expression.
GGT1 and GGT2 are apoplastic enzymes. Their genes are consecutive and they are very similar (96% identical), indicating a duplication event during evolution. Despite this marked similarity, GGT1 is bound to the cell wall and expressed in all organs (and most intensively at the conductive element level), whereas GGT2 is likely to be bound to the plasma membrane, and it is expressed mainly in the silique, where it accounts for 50% of all GGT activity (Martin et al., 2007).
GGT3 is considered a pseudogene because it has an unusually truncated sequence. GGT4 is expressed in vacuoles, where it contributes to the detoxification of xenobiotics following their conjugation with GSH by glutathione sulphotransferases (Grzam et al., 2007).
GGT1 knockout mutant protoplasts of A. thaliana are unable to retrieve GSH from the growth medium (Ohkama-Otsu et al., 2007a), but Arabidopsis plants lacking the functional GGT1 isoform (which was believed to be the only apoplastic GGT expressed in roots) can survive when grown in a nutrient solution supplemented with GSH as the sole source of sulphur (Martin et al., 2007). The ability of ggt1 plants to grow in media containing GSH, combined with the fact that ggt1 mutants exhibit no striking phenotype, has led to GGT1 being assigned a minor role in the recovery of extracellular GSH. Given a presumed functional redundancy in GSH retrieval mechanisms, it was concluded that extracellular GGT is dispensable, and it was argued that other mechanisms unrelated to GGT activity may be responsible for GSH uptake in ggt1 mutants. Another study found, however, that barley seedlings retrieved GSH from an external medium, but this function was severely impaired when serine/borate was added to inhibit GGT activity (Ferretti et al., 2009), suggesting that GSH recovery from an external medium relies on GGT activity.
Investigations were therefore carried out to determine whether the apoplastic, membrane-bound GGT2 isoform, normally strongly expressed in Arabidopsis seeds, might also be expressed in the roots to compensate for the loss of GGT1 function. To test this hypothesis, gene expression and enzyme histochemical analyses, in vivo and in vitro GGT activity measurements, and GSH uptake timing measurements were conducted in wild-type and ggt1 knockout mutant plants.
Materials and methods
Plant material and growth conditions
After 4 d of stratification at 4 °C in the dark, A. thaliana L. ecotype Columbia (Col-0) plants were grown hydroponically on rock wool, as described in Huttner and Bar-Zvi (2003), in controlled-environment chambers (10/14 h light/dark cycle at a temperature of 22/18 °C, with 130 μmol m−2 s−1 photosynthetically active radiation, 70% relative humidity). The nutrient solution used was a modified Gibeaut medium (Gibeaut et al., 1997) containing: 1.5 mM Ca(NO3)2; 1.25 mM KNO3; 0.75 mM Mg(SO4); 0.5 mM KH2PO4; 0.1 mM FeNaEDTA, fresh; 50 μM KCl; 10 μM MnSO4; 1.5 μM CuSO4; 2 μM ZnSO4; 50 μM H3BO3; 0.075 μM (NH4)6Mo7O24.
Roots, fully grown leaves, and seeds from untreated wild-type and ggt1 knockout plants were harvested for gene expression and enzyme histochemical analyses. Plants of both genotypes at the fully expanded rosette stage, ∼1 week before flowering, were used to monitor the time course of GSH depletion from the incubation medium, and for in vitro and in vivo GGT activity measurements.
The Arabidopsis Genome Initiative locus identifiers for the four members of the GGT gene family are as follows: GGT1 (At4g39640), GGT2 (At4g39650), GGT3 (AT1G69820), and GGT4 (At4g29210). The ggt1 knockout mutant line (ecotype Columbia) was found in the mutant collection (Alonso et al., 2003) established at the Salk Institute and available from the Nottingham Arabidopsis Stock Centre (http://nasc.nott.ac.uk; polymorphism SALK_080363). This line was used and characterized in a previous study, where it was named ggt1-2 (Martin et al., 2007). The homozygous plants containing the T-DNA insert located in the second exon of the GGT1 gene were screened by PCR using the gene-specific primers GGT1-F6 (5′-AATTTTGTTTTCGGGAAAGTTCGAG-3′) and GGT1-R6 (5′-CGCCTACTGATAAGGCTCCTTTCTT-3′), and the T-DNA left border primer (5′-GGCAATCAGCTGTTGCCCGTCTCACT-3′). T-DNA integration was confirmed by sequencing the amplification products of the T-DNA flanking region, according to Sanger et al. (1977) at the CRIBI, University of Padua, using the ABI PRISM Big Dye Terminator (Perkin Elmer) Kit.
Preparation of total RNA and cDNA synthesis
Total RNA was extracted from Arabidopsis plant tissues using the RNeasy Plant Mini Kit™ (Qiagen, Valencia, CA, USA), followed by a DNA removal step with the QIAGEN RNase-Free DNase Set™ according to the manufacturer's instructions.
The first-strand cDNA was synthesized from 2 μg of total RNA using the SuperScript™ III First-Strand Synthesis System (Invitrogen Corporation, Carlsbad, CA, USA) with an oligo(dT) primer, according to the manufacturer's protocol.
cDNA cloning and sequencing
To confirm its identity, the GGT3 amplification product obtained was subcloned in the pGEM-T Easy Vector (Promega, Milano, Italy), and the plasmids from six recombinant colonies were sequenced. Plasmids were prepared using the QIAprep Spin Miniprep Kit (Qiagen, Hilden, Germany) and sequenced according to Sanger (1977). Sequences were compared using the BLASTn computer program (NCBI, National Center for Biotechnology Information).
Semi-quantitative RT-PCR analysis
The semi-quantitative RT-PCR analysis was conducted using the primer sequences described in Table 1.
Gene | Gene locus | Primer name | Primer sequence 5'–3' |
GGT1 | At4g39640 | GGT1f | GTGTTCTGTTATCGGGATGCG |
GGT1r | GTTGTCCCGGCAATAATGTTGG | ||
GGT2 | At4g39650 | GGT2f | CCGTAACCCGAAATTGGCTGAT |
GGT2r | GTTGTACCGGCGATGATGTACA | ||
GGT3 | At1g69820 | GGT3f | GGCTCTCTCGGGGTTCATCGACTT |
GGT3r | TGCCGGTGGTGGCACAGT | ||
GGT4 | At4g29210 | GGT4f | CCAAATGCTCTCGAAGGTCA |
GGT4r | ACCCCTATGTGATCGCCATTG | ||
ACT11 | At3g12110 | ACTf | CTGGAGATGATGCACCAAGA |
ACTr | CCTCATCACCAACGTAAGCA |
Gene | Gene locus | Primer name | Primer sequence 5'–3' |
GGT1 | At4g39640 | GGT1f | GTGTTCTGTTATCGGGATGCG |
GGT1r | GTTGTCCCGGCAATAATGTTGG | ||
GGT2 | At4g39650 | GGT2f | CCGTAACCCGAAATTGGCTGAT |
GGT2r | GTTGTACCGGCGATGATGTACA | ||
GGT3 | At1g69820 | GGT3f | GGCTCTCTCGGGGTTCATCGACTT |
GGT3r | TGCCGGTGGTGGCACAGT | ||
GGT4 | At4g29210 | GGT4f | CCAAATGCTCTCGAAGGTCA |
GGT4r | ACCCCTATGTGATCGCCATTG | ||
ACT11 | At3g12110 | ACTf | CTGGAGATGATGCACCAAGA |
ACTr | CCTCATCACCAACGTAAGCA |
Gene | Gene locus | Primer name | Primer sequence 5'–3' |
GGT1 | At4g39640 | GGT1f | GTGTTCTGTTATCGGGATGCG |
GGT1r | GTTGTCCCGGCAATAATGTTGG | ||
GGT2 | At4g39650 | GGT2f | CCGTAACCCGAAATTGGCTGAT |
GGT2r | GTTGTACCGGCGATGATGTACA | ||
GGT3 | At1g69820 | GGT3f | GGCTCTCTCGGGGTTCATCGACTT |
GGT3r | TGCCGGTGGTGGCACAGT | ||
GGT4 | At4g29210 | GGT4f | CCAAATGCTCTCGAAGGTCA |
GGT4r | ACCCCTATGTGATCGCCATTG | ||
ACT11 | At3g12110 | ACTf | CTGGAGATGATGCACCAAGA |
ACTr | CCTCATCACCAACGTAAGCA |
Gene | Gene locus | Primer name | Primer sequence 5'–3' |
GGT1 | At4g39640 | GGT1f | GTGTTCTGTTATCGGGATGCG |
GGT1r | GTTGTCCCGGCAATAATGTTGG | ||
GGT2 | At4g39650 | GGT2f | CCGTAACCCGAAATTGGCTGAT |
GGT2r | GTTGTACCGGCGATGATGTACA | ||
GGT3 | At1g69820 | GGT3f | GGCTCTCTCGGGGTTCATCGACTT |
GGT3r | TGCCGGTGGTGGCACAGT | ||
GGT4 | At4g29210 | GGT4f | CCAAATGCTCTCGAAGGTCA |
GGT4r | ACCCCTATGTGATCGCCATTG | ||
ACT11 | At3g12110 | ACTf | CTGGAGATGATGCACCAAGA |
ACTr | CCTCATCACCAACGTAAGCA |
For all PCR amplification reactions, 1 μl of the cDNA obtained was used in a 20 μl final volume, using 1.0 U of Platinum® Taq DNA Polymerase (Invitrogen Corporation). Different numbers of cycles (from 24 to 40) were tested to choose those corresponding to the exponential phase for each gene, then all semi-quantitative PCR analyses were performed for 35 cycles. Reactions were carried out with the Gene Amp PCR system 9700 (PE Biosystems, Branchburg, NJ, USA) starting with an initial denaturation step at 94 °C for 3 min, then cycling at 94 °C for 30 s, 59 °C for 30 s, 72 °C for 30 s; these steps were followed by a final incubation at 72 °C for 7 min. The A. thaliana β-actin gene (At3g12110) was used as an endogenous internal standard to normalize the gene expression results obtained. A 20 μl aliquot of the PCR products was resolved by electrophoresis on gels stained with ethidium bromide, then scanned and quantified using the Image J64 software (National Institute of Health, Bethesda, MD, USA). To confirm the results of the expression analysis, PCRs were carried out on cDNAs obtained from two different RNA extractions from plant tissues in two independent experiments and repeated three times for each cDNA.
Exogenous glutathione supplementation
For the depletion study, hydroponically grown wild-type and ggt1 Arabidopsis plants were placed in test tubes containing 5 ml of 100 μM GSH, with or without 10 mM serine/borate as the competitive GGT inhibitor. A 50 μl aliquot of the solution was then drawn off after 0, 1, 3, 6, and 8 h of incubation and stored at –80 °C for several days before thiol chromatographic analysis. For in vivo GGT activity, plants were incubated with 100 μM GSH and harvested after 3 h and 6 h of incubation; single plant roots were transferred to a 5 ml tube containing 4.4 ml of bioassay solution.
HPLC and thiol measurement
Following reduction with tributylphosphine, 50 μl of growth medium was derivatized with the SBD-F (ammonium 7-fluoro 2,1,3-benzooxadiazole-4-sulphonate) fluorophore; low molecular weight thiol-SBD derivatives were then separated by chromatography according to the method described in Masi et al. (2002). Samples were collected from at least five distinct biological replicates.
Enzyme histochemical analysis of GGT activity
Leaves, roots, and seeds from A. thaliana were embedded in optimal cutting temperature medium (OCT, Cellpath, Newtown, UK) with no prior chemical fixation, then placed in 15 mm cryomoulds, snap-frozen, and stored at –80 °C until use. Sections were collected on glass slides with an adhesive coating and air dried overnight. Following immersion in cold pure acetone for 15 s and rinsing for 30 s in 50% acetone solution, the slides were stained essentially according to the procedure described by Rutenburg et al. (1969). After washing in distilled water, GGT activity was identified by placing the tissues in a freshly prepared staining solution containing 0.46 mM γ-glutamyl-4-methoxy-2-naphthylamide as a substrate, and 6.3 mM glycyl-glycine (gly-gly) as a γ-glutamyl acceptor, in phosphate buffer pH 7.4. Diazotization was done with the diazonium salt Fast Garnet GBC (0.35 mg ml−1 incubation medium), extending the reaction for 90 min at room temperature, with gentle shaking. A control stain was obtained by incubating the tissue sections in a medium containing 10 mM serine/borate (Tate and Meister, 1978).
Enzyme histochemical analysis was also performed in wild-type and ggt1 leaves after incubating them overnight in pure acetone to remove chlorophyll and wounding them with a razor blade to enable infiltration of the incubation medium.
All images were acquired with a Leica DM4000B digital microscope, equipped with a Leica DC300F camera and Leica Image Manager 50 software (Leica Microsystems).
Enzyme extraction and in vitro GGT activity assay
The sequential extraction procedure adopted for this study was based on the one used in Martin et al. (2007), with some modifications. A 1 g aliquot of root tissue from both genotypes was frozen in liquid nitrogen, pulverized in an ice-cold mortar, and homogenized in 2 ml of extraction buffer (20 mM TRIS-HCl pH 8.0, protease inhibitor cocktail, 1% Triton X-100). The homogenate was kept at 4 °C for 60 min, with gentle shaking, then centrifuged at 15 000 g for 20 min at 4 °C. The supernatant containing the soluble and membrane-bound protein fraction was collected and assayed for GGT activity.
The pellet was rinsed twice with the same buffer, then incubated with 20 mM TRIS-HCl pH 8.0 buffer containing 3 M NaCl for 60 min to solubilize the proteins ionically bound to the cell wall. It was found that 3 M NaCl enabled an ∼30% higher recovery than 1 M NaCl (data not shown). This fraction was then assayed for GGT activity. The fractions obtained by rinsing the pellets were also tested, but they generally revealed a negligible residual activity, if any (data not shown).
GGT activity was assayed according to Huseby and Strömme (1974), involving the spectrophotometric measurement of absorbance at 407 nm of p-nitroaniline released from the γ-glutamyl-p-nitroanilide (GPNA) substrate.
The reaction was started by adding 0.2 ml of enzyme extract to 1 ml of solution A (4.6 mM GPNA in 100 mM TRIS-HCl pH 8.0, 10 mM MgCl2) and 0.1 ml of solution B (575 mM gly-gly; the pH was adjusted to 8.0 by adding NaOH). The change in absorbance at 40 nm in the assay medium was recorded for 10 min.
In vivo GGT activity measurements
GGT activity in roots was measured in vivo, as described elsewhere (Ferretti et al., 2009). The method is essentially an adaptation of the procedure used to measure in vitro activity (Huseby and Strömme, 1974), and is based on the observation that the root apoplastic space is readily accessed by the external solution.
Single plant roots were placed in a test tube containing 4 ml of solution A (4.6 mM GPNA in 100 mM NaH2PO4 pH 8.0) and 0.4 ml of solution B (575 mM gly-gly in 100 mM NaH2PO4 pH 8.0); the solution was cycled through home-made piping from the cuvette to the spectrophotometer cell using a peristaltic pump operated at a flow rate of 3 ml min−1. The change in absorbance at 407 nm induced by releasing p-nitroaniline into the assay medium was recorded for 10 min.
Statistical analysis
Data were submitted to analysis of variance (ANOVA), and the Tukey honest significant difference (HSD) multiple comparisons procedure was used to discriminate between means. A significance level of P <0.05 was adopted for all comparisons. The Statgraphics Plus rel. 5.0 Software package (Manugistics Inc., Rockville, MD, USA) was used for the statistical analysis.
Results
The comparison between the hydroponically grown A. thaliana wild-type and ggt1 knockout mutants showed that the latter exhibited no clearly visible phenotype, but a progressive senescence, demonstrated by a shorter time to flowering, which took ∼4 d less (Fig. 1).
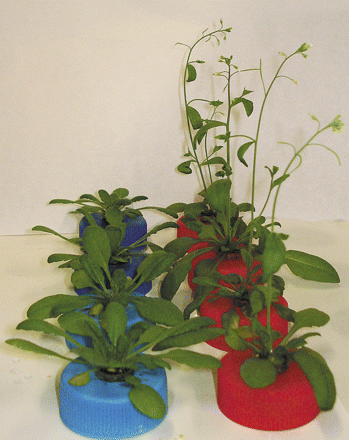
Arabidopsis thaliana wild-type (left) and ggt1 knockout mutant plants (right) grown in hydroponics.
GGT gene expression in Arabidopsis thaliana
The relative expression of the known GGT isoforms in A.thaliana was investigated by semi-quantitative PCR analysis in different tissues (roots, leaves, and seeds).
The expression data in Fig. 2 indicate that GGT1 is expressed in all tissues, albeit with a different intensity (leaves>roots>seeds). GGT3 is only detected in seeds. GGT4 is detected in all tissues, but considerably more in roots. GGT2 transcripts give rise to a very faint band in roots at 30 PCR cycles, but a clear band could be seen after increasing to 40 cycles. GGT3 transcripts yielded a clear band in siliques and very faint bands in leaves and roots. The authenticity of the GGT3 transcripts was confirmed by sequencing the 396 bp amplification product, which was 100% identical to the nucleotide sequence of At1g69820. No GGT2 expression was ever seen in leaves, not even at 40 cycles.
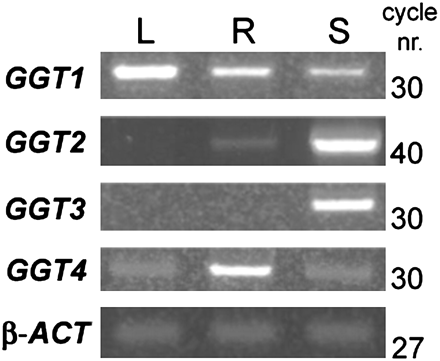
GGT gene expression patterns in different tissues. Relative abundance of each GGT transcript in leaf (L), root (R), and silique (S). The number of PCR cycles is indicated on the right. All reactions were performed at 30 cycles, but the At4g39650 PCR was extended to 40 cycles to highlight the presence of its transcripts in root tissues. The same quantity of mRNA from different tissues was used to obtain cDNA, and the products were amplified with each GGT gene-specific primer pair. β-Actin was used as the internal standard. GGT1, At4g39640; GGT2, At4g39650; GGT3, At1g69820; GGT4, At4g29210; β-ACT, β-actin.
Enzyme histochemical localization of GGT activity
Figure 3 shows the enzyme histochemical detection of GGT activity in different organs and tissues of A. thaliana wild-type plants. Red to brownish staining results from the diazotization reaction of the unstable diazonium salt Fast Garnet GBC with p-nitroaniline released from GPNA by GGT activity. Staining was seen at the root apex (Fig. 3A), in conductive tissues closely associated with vascular bundles (Fig. 3B), and in the epidermal cell layer (Fig. 3E); in seeds, intense staining was apparent in the endosperm (Fig. 3C, D). The specificity of staining was demonstrated by using 10 mM serine/borate to inhibit GGT activity (Fig. 3F).
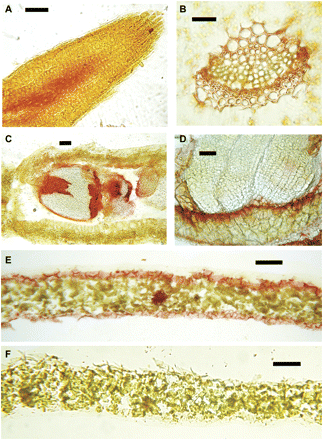
Enzyme histochemical detection of GGT activity in different tissues of A. thaliana. Tissue sections were incubated in a solution containing γ-glutamyl-p-nitroanilide and stained with Fast Garnet GBC. Reddish to purplish-brown staining indicates deposition of diazonium salts at the site of GGT activity: (A) root tip, longitudinal section; (B) vascular bundle, leaf cross-section; (C) silique, longitudinal section; (D) silique, seed magnified; (E) leaf, cross-section; (F) leaf cross-section in 10 mM serine/borate to inhibit GGT activity. Scale bars: A–D=100 μm; E, F=200 μm.
Enzyme histochemical analysis was also performed to compare wild-type and ggt1 knockout mutants. As shown in Fig. 4A and C, root sections only exhibited staining in the vascular tissue in wild-type plants. Whole leaves (Fig. 4B, D) were analysed by wounding the leaves with a razor blade to enable infiltration of the incubation medium. Red staining was seen around the wounded region in wild-type, but not ggt1 knockout leaves.
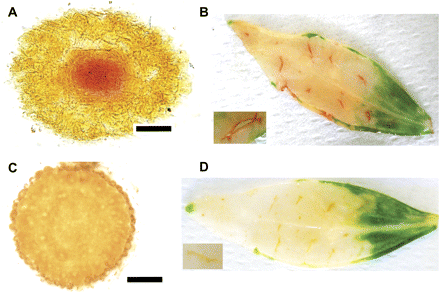
Enzyme histochemical detection of GGT activity in wild-type and ggt1 knockout roots and leaves. (A) Root apex transversal section, wild type; (B) wounded leaf, wild type; (C) root apex transversal section, ggt1; (D) wounded leaf, ggt1. Inset pictures in B and D are enlargements of a wounded area. Scale bars=100 μm.
GSH depletion time course analysis
The ability of wild-type and ggt1 roots to retrieve exogenous GSH is illustrated in Fig. 5. The wild-type Arabidopsis roots depleted a solution containing 100 μM GSH more quickly than the ggt1 knockout mutants; adding the serine/borate inhibitor almost completely prevented the uptake process (Fig. 5A). GSH depletion from the growth medium was paralleled by an increase in cysteinyl-glycine and cysteine (Fig. 5B, C, respectively). This increase was lower in ggt1 mutants compared with the wild type, and almost completely abolished in the presence of the serine/borate inhibitor.
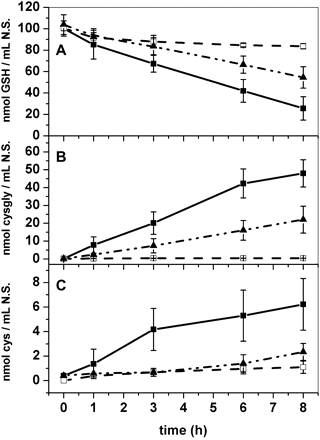
Timing of glutathione depletion from the incubation medium. Wild-type, with or without 10 mM serine/borate inhibitor, and ggt1 roots were incubated in 100 μM glutathione; the solution was sampled at different times and used for thiol quantification by HPLC analysis. (A) Glutathione content; (B) cysteinyl-glycine content; (C) cysteine content in the external solution. Data are expressed as nmol ml−1 solution. Filled squares, wild-type roots; filled triangles, ggt1 roots; open squares, wild-type roots with 10 mM serine/borate inhibitor (n=10).
In vivo measurements of apoplastic GGT activity
To test the hypothesis that GGT activity is partially restored in ggt1 knockout mutants, the roots of intact plants were incubated with a GPNA solution and the rate of p-nitroaniline release was measured after 3 h and 6 h of incubation; this procedure was described in a previous study on barley seedlings (Ferretti et al., 2009).
Figure 6 shows the GGT activity induced in ggt1 roots: it was hardly detectable in vivo under normal conditions, but it increased noticeably after 3 h and 6 h of incubation with GSH. GGT activity in the wild-type roots was unaffected after 3 h and 6 h of incubation (not shown).
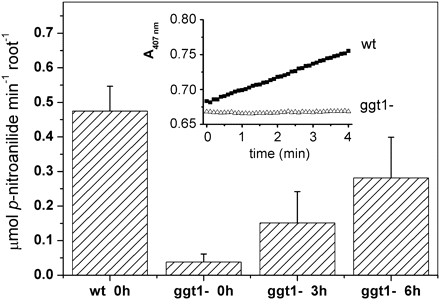
In vivo measurement of GGT activity in wild-type and ggt1 knockout A. thaliana roots. GGT activity is measured in vivo as the release of p-nitroaniline by Arabidopsis roots placed in a spectrophotometric cuvette containing 4.6 mM γ-glutamyl-p-nitroanilide; the solution was cycled through home-made piping from the cuvette to the spectrophotometer cell using a peristaltic pump (see Materials and methods for details). Plants incubated with 100 μM glutathione were harvested after 3 h and 6 h of incubation; single plant roots were transferred to a 5 ml tube containing 4.4 ml of bioassay solution. The change in absorbance at 407 nm induced by releasing p-nitroaniline into the assay medium was recorded for 10 min. Inset graph: spectrophotometric recording of the assay solution in contact with wild-type or ggt1 roots. Values are means ±SE of at least five replicates.
GGT activity in roots from wild-type and ggt1 knockout plants was also measured after 24 h of incubation with 100 μM GSH, adopting a sequential extraction and using Triton X-100 and 3 M NaCl in order to discriminate between membrane-bound and soluble GGT activity from cell wall-bound activity. In the Triton X-100 fraction, GGT activity was similar in the wild-type and ggt1 root extracts, but 24 h of treatment with 100 μM GSH prompted a 3- to 4-fold increase in GGT activity by comparison with untreated plants. In wild-type extracts, GGT activity was much higher in the fractions obtained by 3 M NaCl extraction than in those obtained using Triton X-100; it was almost undetectable in the ggt1 extracts.
Semi-quantitative gene expression analysis following GSH supplementation
Based on the finding that GGT activity was partially restored in ggt1 roots after incubation with GSH, a gene expression analysis of the four ggt genes was performed in wild-type and ggt1 roots and shoots after GSH supplementation in the growth medium (Fig. 7).
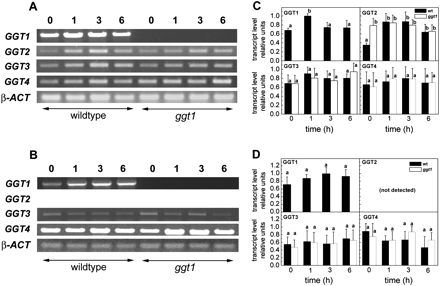
Gene expression analysis by semi-quantitative PCR of the four ggt genes in Arabidopsis thaliana wild-type and ggt1 knockout mutants, after 100 μM glutathione supplementation. Roots and leaves were harvested before treatment and then 1, 3, and 6 h after adding 100 μM glutathione to the growth medium. Gene expression analysis of the four ggt genes: PCR amplification products and relative expression levels in roots (A, C) and leaves (B, D). GGT1, At4g39640; GGT2, At4g39650; GGT3, At1g69820, GGT4, At4g29210; β-ACT, At3g12110. Data are presented as means ±SD from at least five independent measurements; error bars represent the SD. Different letters indicate significant differences between the wild type and ggt1 knockout lines (P <0.05); significance was evaluated with the Tukey test. See Materials and methods for further details.
As expected, inserting a T-DNA sequence in the mutant genotype results in the early truncation of the reading frame and complete loss of GGT1 transcripts. Incubation with 100 μM GSH led to a transient GGT1 stimulation in wild-type roots, observable after 1 h. GGT2 was constitutively up-regulated in ggt1 roots, and was stimulated by GSH supplementation in the wild type. No GGT2 transcripts were ever detected in leaves. Some stimulation of GGT3 and GGT4 expression was generally observed in roots, but the differences were not statistically significant.
Discussion
Histochemical detection of GGT activity in A. thaliana
The histochemical detection of GGT activity is an effective tool for investigating GGTs in their active form and in different organisms. Here, enzyme histochemical analyses were used to compare wild-type and ggt1 Arabidopsis tissues (Figs 3, 4) to complement the findings of published reports on gene expression analyses. Consistently with previous observations in barley (Ferretti et al., 2009) and maize organs and tissues (Masi et al., 2007), Arabidopsis wild-type leaves exhibited intense staining in the vascular tissue, epidermis, and stomata, while virtually no staining was apparent in the ggt1 leaves (Fig. 4), confirming that GGT1 is the isoform most expressed in leaves, where GGT2 is not expressed at all. Consistently with previous observations in maize (Masi et al., 2007) and barley (Ferretti et al., 2009) roots, enzyme histochemical detection revealed a strong GGT activity in Arabidopsis wild-type root tips, but very little in ggt1 knockout roots (Fig. 4A, C). Overall, the histochemical analysis demonstrated that GGT activity correlates with the spatial distribution of GGT transcripts, as previously reported (Martin et al., 2007), suggesting that GGT activity is indeed regulated at the gene expression level—although post-translational changes, which are known to be induced by GGTs in animal cells, cannot be ruled out.
Regulation of GGT2 expression in A. thaliana roots by GSH availability and lack of functional GGT1
T-DNA insertion results in the disruption of the functional GGT1 gene in Arabidopsis, but GSH uptake is still possible in ggt1 mutants, albeit at a slower rate than in wild-type plants. Adding serine/borate (a competitive GGT inhibitor) significantly hampers this process, however (Fig. 5A). The present findings indicate that another protein with GGT activity (other than GGT1) sustains GSH degradation, as shown by the parallel rise in cysteinyl-glycine content in ggt1 plants. GGT3 (which is considered a pseudogene) is unlikely to contribute any activity and GGT4 is restricted to the vacuole, where it assists in the degradation of GSH conjugates (Grzam et al., 2007). It is worth mentioning that GGT3 transcripts were detected in this work (and verified by sequencing), in contrast to the report from Ohkama-Otsu et al. (2007a), but in accordance with the findings of Martin et al. (2007), who observed β-glucuronidase (GUS) activity in lines carrying the GUS:GGT3 fusion construct. Whether a functional protein originates from GGT3 transcripts is not known, but it would seem unlikely because it lacks a sequence that is important in conferring catalytic activity (Okada et al., 2007).
Here, it is experimentally demonstrated that GGT2 is also expressed in roots, and it is up-regulated both when the GGT1 gene is disrupted and in the presence of extracellular GSH. A transient up-regulation of GGT1 expression (observable after 1 h of incubation; see Fig. 7C) in wild-type roots was also induced by GSH supplementation, suggesting that the two genes might be similarly regulated by this stimulus.
The extracellular location of GGT1 and GGT2 activity can be exploited to measure root GGT activity in vivo by monitoring the release of p-nitroaniline from its γ-glutamyl precursor, GPNA (Ferretti et al., 2009). This approach is effective in demonstrating large differences in GGT activity in A. thaliana wild-type versus ggt1 roots. Incubation with 100 μM GSH restored in vivo GGT to nearly 50% by comparison with the wild-type after 6 h (Fig. 6), and this finding is consistent with the gene expression data in Fig. 7 and the data in Table 2, indicating a greater GGT activity in the membrane-bound protein fraction after GSH supplementation.
GGT activity in wild-type and ggt1 knockout root extracts with and without treatment with 100 μM GSH
Treatment | Extraction medium | GGT activity (nmol p-nitroaniline g−1 FW min−1) | |
Wild type | ggt1 | ||
Control | + Triton X-100 | 44.22±8.70 | 34.19±5.44 |
+ 100 μM GSH | + Triton X-100 | 151.28±38.89 | 121.69±29.86 |
Control | + 3 M NaCl | 297.69±77.77 | 7.23±1.48 |
+ 100 μM GSH | + 3 M NaCl | 335.65±41.76 | 6.91±4.64 |
Treatment | Extraction medium | GGT activity (nmol p-nitroaniline g−1 FW min−1) | |
Wild type | ggt1 | ||
Control | + Triton X-100 | 44.22±8.70 | 34.19±5.44 |
+ 100 μM GSH | + Triton X-100 | 151.28±38.89 | 121.69±29.86 |
Control | + 3 M NaCl | 297.69±77.77 | 7.23±1.48 |
+ 100 μM GSH | + 3 M NaCl | 335.65±41.76 | 6.91±4.64 |
Roots were incubated in 100 μM GSH medium and harvested after 24 h. GGT activity was measured in vitro on different fractions obtained from wild-type and ggt1 roots with and without GSH treatment using the following sequential extraction procedure: roots were homogenized with Triton X-100 containing TRIS buffer pH 8, enabling the extraction of both soluble and membrane-bound proteins. After centrifugation, the supernatant was collected and the pellet was incubated twice with the same buffer to eliminate any residual activity completely. Then the pellet was incubated with 3 M NaCl containing TRIS buffer to solubilize proteins bound to the cell wall. Activity was measured as the release of p-anitroaniline from the artificial γ-glutamyl-p-nitroanilide substrate by monitoring the change in absorbance at 407 nm of the assay solution at 10 min intervals. The values given in the table are the means of four independent experiments. See Materials and methods for additional details.
GGT activity in wild-type and ggt1 knockout root extracts with and without treatment with 100 μM GSH
Treatment | Extraction medium | GGT activity (nmol p-nitroaniline g−1 FW min−1) | |
Wild type | ggt1 | ||
Control | + Triton X-100 | 44.22±8.70 | 34.19±5.44 |
+ 100 μM GSH | + Triton X-100 | 151.28±38.89 | 121.69±29.86 |
Control | + 3 M NaCl | 297.69±77.77 | 7.23±1.48 |
+ 100 μM GSH | + 3 M NaCl | 335.65±41.76 | 6.91±4.64 |
Treatment | Extraction medium | GGT activity (nmol p-nitroaniline g−1 FW min−1) | |
Wild type | ggt1 | ||
Control | + Triton X-100 | 44.22±8.70 | 34.19±5.44 |
+ 100 μM GSH | + Triton X-100 | 151.28±38.89 | 121.69±29.86 |
Control | + 3 M NaCl | 297.69±77.77 | 7.23±1.48 |
+ 100 μM GSH | + 3 M NaCl | 335.65±41.76 | 6.91±4.64 |
Roots were incubated in 100 μM GSH medium and harvested after 24 h. GGT activity was measured in vitro on different fractions obtained from wild-type and ggt1 roots with and without GSH treatment using the following sequential extraction procedure: roots were homogenized with Triton X-100 containing TRIS buffer pH 8, enabling the extraction of both soluble and membrane-bound proteins. After centrifugation, the supernatant was collected and the pellet was incubated twice with the same buffer to eliminate any residual activity completely. Then the pellet was incubated with 3 M NaCl containing TRIS buffer to solubilize proteins bound to the cell wall. Activity was measured as the release of p-anitroaniline from the artificial γ-glutamyl-p-nitroanilide substrate by monitoring the change in absorbance at 407 nm of the assay solution at 10 min intervals. The values given in the table are the means of four independent experiments. See Materials and methods for additional details.
These findings suggest that GGT2 expression at least partially compensates for the loss of GGT1, and that it contributes to the retrieval of extracellular GSH, which also acts as a positive signal for GGT gene expression. The inducibility of GGT genes by GSH is also supported by some data available in the literature: high levels of GGT1, GGT2, and GGT3 expression were observed in natural conditions in developing trichomes (Martin et al., 2007), where the GSH content is nearly 300 times higher than in the epidermal layer of the leaves (Gutiérrez-Alcalá et al., 2000).
Compensatory GGT2 up-regulation occurs in roots, but not in leaves, where GGT2 transcripts were never detected in any of the present cases (Fig. 7), a finding consistent with data available in the literature. The lack of the GGT2 isoform in leaves when GGT1 is also missing suggests that a γ-glutamyl cycle for the retrieval of extracellular GSH might be unnecessary in leaves, which can rely on their intracellular biosynthetic machinery to control GSH availability. The GGT2 expression observed in this work is also consistent with the weak GUS expression already reported in roots of Arabidopsis lines carrying the GUS:GGT2 construct (Martin et al., 2007).
The contribution of GGT2 (known to be strongly expressed in siliques) to total GGT activity in roots may be low, but not negligible, and it may increase through substrate availability. GGT1 and GGT4 are therefore not the only functional GGTs in roots. It follows that, despite major similarities between GGT1 and GGT2 in terms of gene sequences and apoplastic location, the two GGT isoforms exhibit some significant differences. For a start, they are associated with a different cell structure, since GGT1 is ionically bound to the cell wall, whereas GGT2 seems to be associated with membranes. They exhibit a different spatial and temporal expression; and their expression is differently regulated, GGT2 being inducible in roots by GSH, its natural substrate, whilst GGT1 expression among tissues is rather constitutive (although some inducibility by GSH has been observed in this work) and linked to developmental stage. The higher GGT2 expression in ggt1 roots under basal conditions might also indirectly result from the increase in GSH content in the extracellular space.
The possible contribution of GGT2 activity to the recovery of extracellular GSH in ggt1 mutants might explain the ability of the latter (see Martin et al., 2007), or ggt1/5Opase, or ggt1/ggt4 double mutants and ggt1/ggt4/5OPase triple mutants to grow in media containing GSH as the sole source of sulphur (Ohkama-Ohtsu et al., 2008).
Taken together, these observations suggest that GGT1 and GGT2 isoforms cooperate in GSH recovery from the extracellular space in A. thaliana roots. Although additional mechanisms for GSH recovery may be at work in different plant organs and at different developmental stages, in the present experimental conditions GGTs seem to have a major role in the retrieval of extracellular GSH.
This means that the existence of apoplastic GGTs in roots should be taken into due account in GSH feeding experiments. Previous studies (e.g. Lappartient et al., 1999; Bashandy et al., 2010) should therefore be reconsidered bearing in mind that external GSH can be effectively degraded extracellularly and resynthesized starting from the constituent amino acids after their recovery by means of amino acid transporters.
GGT and GSH degradation pathways
The role of the apoplastic GGTs, GGT1 and GGT2, remains an open question. It is tempting to suggest that they could participate in GSH retrieval and inter-organ redistribution, as in animal organisms, but this function is harder to assess in plants. In leaves, where GGT2 is never expressed under any conditions or at any developmental stage, even GGT1 silencing does not result in any visible phenotype. The glutathione level in these organs can be maintained, however, by the cysteine ultimately provided by photosynthesis. The effects in leaves might therefore be minimized by an autonomous capacity for GSH biosynthesis and supply.
In organs other than leaves, such as siliques and roots, where both GGT1 and GGT2 are expressed, it is likewise impossible for the time being to draw any definitive conclusions. When the major GGT1 isoform is lacking in roots, GGT2 can compensate for the loss and ensure some activity, which is probably sufficient to minimize the effects of the mutation. In siliques, both isoforms are equally expressed, and silencing of either isoform is compensated for by the other.
A conclusive analysis on the role of extracellular GGT might be possible on ggt1/ggt2 double mutants, but, because the two genes are consecutive, the chances of obtaining a double mutant by conventional cross-breeding are virtually nil. Different strategies should therefore be explored (e.g. multiple gene silencing by RNA interference) in order to strongly reduce the expression of both isoforms.
At this stage, it is impossible to establish whether apoplastic GGTs are just one of a number of systems, or the most important one that plant cells use to retrieve extracellular GSH. It may also be that GGTs have as yet unknown functions that need to be clarified. In the extracellular space, where oxidizing conditions dominate, a γ-glutamyl cycle may be functional to some kind of apoplastic redox control and/or to transfer redox signals from the outside world to the cytosol. The only visible phenotype observed in ggt1 plants (i.e. is a shorter life cycle) may actually be interpreted as an overall difficulty in the plant's adaptation to the environment.
This work was funded by the MURST ex-60%. IKR and DP were supported by a fellowship granted by the Italian Ministry of Research. ILB was granted a post-doctoral fellowship by the University of Padua. Critical reading of the manuscript by Cornelia Herschbach and Sonja Veljovic-Jovanovic is gratefully acknowledged. We also thank Serena Varotto for kindly helping in microscope image acquisition.
Comments