-
PDF
- Split View
-
Views
-
Cite
Cite
Li-Ting Chen, Ming Luo, Yu-Yuan Wang, Keqiang Wu, Involvement of Arabidopsis histone deacetylase HDA6 in ABA and salt stress response, Journal of Experimental Botany, Volume 61, Issue 12, July 2010, Pages 3345–3353, https://doi.org/10.1093/jxb/erq154
- Share Icon Share
Abstract
Histone modifications play an important role in the epigenetic regulation of gene expression. All histone modifications are reversible, which may therefore provide a flexible way for regulating gene expression during the plant's development and during the plant response to environmental stimuli. The reversible acetylation and deacetylation of specific lysine residues on core histones are catalysed by histone acetyltransferases and histone deacetylases (HDAs). HDA6 is an RPD3-type histone deacetylase in Arabidopsis. The Arabidopsis HDA6 mutant, axe1-5, and HDA6 RNA-interfering plants displayed a phenotype that was hypersensitive to ABA and salt stress. Compared with wild-type plants, the expression of the ABA and abiotic stress-responsive genes, ABI1, ABI2, KAT1, KAT2, DREB2A, RD29A, and RD29B, was decreased in axe1-5 and HDA6 RNA-interfering plants when treated with ABA or salt stress. It was found that both ABA and salt stress could enrich the gene activation markers, histone H3K9K14 acetylation, and H3K4 trimethylation, but decrease the gene repression marker, H3K9 dimethylation, of the ABA and abiotic stress-responsive genes. Our study indicates that HDA6-involved histone modifications modulate seed germination and the salt stress response, as well as ABA- and salt stress-induced gene expression in Arabidopsis.
Introduction
In eukaryotic cells, gene activity is controlled not only by DNA sequences but also by epigenetic markers, which can be transmitted to a cell's progeny during mitosis or meiosis (Berger, 2007). Molecular mechanisms underpinning epigenetic events include DNA methylation and the alteration of the nucleosome core histones through acetylation, methylation, phosphorylation, and ubiquitinylation. These changes in chromatin structure determine gene expression by activation or silencing. For example, the acetylation via histone acetyltransferases of histone tails is associated with active transcription, while deacetylation through histone deacetylase (HDA) complexes normally results in gene repression (Chen and Tian, 2007). Furthermore, histone modifications can be used as the marker to understand the activity of target genes. For example, histone H3 Lys9 (H3K9) acetylation, histone H3 Lys14 (H3K14) acetylation, and histone H3 Lys4 (H3K4) trimethylation are the markers for active genes, whereas H3K9 deacetylation, H3K14 deacetylation, and H3K9 dimethylation are the markers for silenced genes (Earley et al., 2006; Chen and Tian, 2007).
The ability of epigenetic changes to alter rapidly and reversibly, yet with the potential to keep a stable ‘memory’ through many cell divisions, could be a key component to the flexibility of plant responses to the environment (Bruce et al., 2007). Increased H3 acetylation was found after submergence of rice plants which led to increasing expression of stress-responsive genes (Tsuji et al., 2006). Up-regulation of stress-inducible genes in tobacco and Arabidopsis cells in response to abiotic stresses and ABA treatment correlates with dynamic changes in histone H3 and H4 modifications (Sokol et al., 2007). Recent studies suggested that histone deacetylases were involved in the Arabidopsis biotic and abiotic stress response (Zhou et al., 2005; Wu et al., 2008). Expression of histone deacetylases HDA19 and HDA6 was shown to be induced after attack by A. brassicicola or by wounding. It was demonstrated that HDA19 may interact with the transcription factors to regulate gene expression in the plant's response to abiotic and biotic stress (Song et al., 2005; Song and Galbraith, 2006; Kim et al, 2008,a). HOS15, a WD40-repeat protein, is crucial for the repression of genes associated with cold-stress tolerance through histone deacetylation in Arabidopsis (Zhu et al., 2008). The level of acetylated histone H4 is higher in the hos15 mutant than in wild-type plants, suggesting that histone acetylation/deacetylation plays a critical role for gene activation/repression in tolerance to cold stress in Arabidopsis (Zhu et al., 2008). More recently, it was found that histone modifications on histone H3 were altered with gene activation on the coding regions of drought stress-responsive genes under drought stress conditions (Kim et al., 2008b). Taken together, these studies show that epigenetic modifications of chromatin are important in plant stress responses.
In this study, the effects of abscisic acid (ABA) and salt stress on the histone acetylation and methylation of abiotic stress response genes were investigated. It was found that both ABA and salt stress can induce histone H3K9K14 acetylation and H3K4 trimethylation but decrease H3K9 dimethylation of some ABA and abiotic stress-responsive genes. axe1-5 is an HDA6 splicing mutant which carries a point mutation in the HDA6 splicing site (Murfett et al., 2001). It was found that axe1-5 and HDA6 RNA-interference (HDA6-RNAi) plants displayed a phenotype that is more sensitive to ABA and salt stress. Compared with wild-type plants, the expression of ABA and abiotic stress-responsive genes was decreased in axe1-5 and HDA6-RNAi plants. Our study indicated that gene expression induced by ABA and abiotic stress is associated with changes in histone modifications and HDA6 is involved in ABA and abiotic stress responses in Arabidopsis thaliana.
Materials and methods
Plant materials
Plants were germinated and grown at 23 °C under long-day conditions (16/8 h light/dark cycle). An hda6 mutant line, axe1-5, and an HDA6 RNA interference line, CS24039, were used in this study. axe1-5 is a splicing mutant which carries a point mutation in the HDA6 splicing site and it is in the Col background (Wu et al., 2008). CS24039 is a HDA6-RNAi line in the Ws background (Wu et al., 2008).
Measurement of germination rates
For seeds germinated on Petri dishes, all seeds were treated with 50% bleach solution for 15 min in order to sterilize them and they were then rinsed three times with distilled water. The sterilized seeds were incubated at 4 °C for 3 d to enhance germination and then planted on Petri dishes containing different growth media. The media contained half-strength Murashige and Skoog salt, 1% sucrose, 0.8% agar, and were supplemented with or without ABA and NaCl. All plates were transferred to a growth chamber and incubated at 23 °C under long-day conditions. For germinating rate tests, seeds were germinated on media with or without different concentrations of ABA (0.5 μM, 1 μM, and 2 μM) or NaCl (100 mM, 150 mM, and 200 mM) in a growth chamber under a long-day conditions, and seed germination rates were analysed after 2 d. For high salinity treatment, 5-d-old seedlings growing in Perti dishes were transferred to a medium containing 125 mM NaCl under long-day conditions, and the percentage survival of seedlings was measured after 5 d.
Semi-quantitative RT-PCR analysis
0.1–0.2 grams of Arabidopsis thaliana leaves were ground with liquid nitrogen in a mortar and pestle and mixed with 1 ml TRIZOL Reagent (Invitrogen, Carlsbad, CA, USA) to isolate total RNA. One microgram of total RNA was used for the first-strand cDNA synthesis after incubation at 65 °C for 10 min. cDNA was synthesized in a volume of 20 μl that contained the MoMLV reverse transcriptase buffer (Promega, Madison, Wisconsin, USA), 10 mM dithiothreitol, 1.5 μM poly(dT) primer, 0.5 mM dNTPs, and 2 U of MoMLV reverse transcriptase at 37 °C for 1 h. All PCR reactions were performed with 0.5 U of Taq polymerase, the buffer provided by the supplier, 0.2 mM dNTPs, and a pair of primers (0.1 μM each) in a final volume of 20 μl. PCR parameters differed for each gene. Thermocycling conditions were 94 °C for 4 min followed by 22–35 cycles of 94 °C for 30 s, 50–65 °C for 1 min, and 72 °C for 1 min, with a final polymerization step at 72 °C for 7 min. The gene-specific primer pairs are listed in Table 1.
Genes | Primers |
ABI1 | 5′-GCCATGTCGAGATCCATTGG-3′ and |
5′-GTGGCTGGGGATGCATCGTT-3′ | |
ABI2 | 5′-CAAGATCCATTGGCGATAGATACC-3′ and |
5′-CTTCCGGCGGAGAAAAGAGG-3′ | |
KAT1 | 5′-CTTCATGAAACTTAGAGGGCAAC-3′ and |
5′-GATAGAGAGAGCCAAAGTGG-3′ | |
KAT2 | 5′-AGGTTGCTCTGATGCAGATC-3′ and |
5′-CACAAAGGTCACGAATGCGG-3′ | |
DREB2A | 5′-GATGTGGATCAGAGTCACTTGG-3′ and |
5′-CTCGGATAGAGAATCAACAGTCG-3′ | |
RD29A | 5′-GCCACATTCTGTTGAAGAGG-3′ and |
5′-CCCACTTTAGACCTAGTAGC-3′ | |
RD29B | 5-GATACCTTCCGACCAGATAGC-3′ and |
5′-GTTGGGACTATGGGGTTTTCG-3′ | |
UBQ | 5′-GATCTTTGCCGGAAAACAATTGGAGGATGGT-3′ and |
5′-CGACTTGTCATTAGAAAGAAAGAGATAACAGG-3′ |
Genes | Primers |
ABI1 | 5′-GCCATGTCGAGATCCATTGG-3′ and |
5′-GTGGCTGGGGATGCATCGTT-3′ | |
ABI2 | 5′-CAAGATCCATTGGCGATAGATACC-3′ and |
5′-CTTCCGGCGGAGAAAAGAGG-3′ | |
KAT1 | 5′-CTTCATGAAACTTAGAGGGCAAC-3′ and |
5′-GATAGAGAGAGCCAAAGTGG-3′ | |
KAT2 | 5′-AGGTTGCTCTGATGCAGATC-3′ and |
5′-CACAAAGGTCACGAATGCGG-3′ | |
DREB2A | 5′-GATGTGGATCAGAGTCACTTGG-3′ and |
5′-CTCGGATAGAGAATCAACAGTCG-3′ | |
RD29A | 5′-GCCACATTCTGTTGAAGAGG-3′ and |
5′-CCCACTTTAGACCTAGTAGC-3′ | |
RD29B | 5-GATACCTTCCGACCAGATAGC-3′ and |
5′-GTTGGGACTATGGGGTTTTCG-3′ | |
UBQ | 5′-GATCTTTGCCGGAAAACAATTGGAGGATGGT-3′ and |
5′-CGACTTGTCATTAGAAAGAAAGAGATAACAGG-3′ |
Genes | Primers |
ABI1 | 5′-GCCATGTCGAGATCCATTGG-3′ and |
5′-GTGGCTGGGGATGCATCGTT-3′ | |
ABI2 | 5′-CAAGATCCATTGGCGATAGATACC-3′ and |
5′-CTTCCGGCGGAGAAAAGAGG-3′ | |
KAT1 | 5′-CTTCATGAAACTTAGAGGGCAAC-3′ and |
5′-GATAGAGAGAGCCAAAGTGG-3′ | |
KAT2 | 5′-AGGTTGCTCTGATGCAGATC-3′ and |
5′-CACAAAGGTCACGAATGCGG-3′ | |
DREB2A | 5′-GATGTGGATCAGAGTCACTTGG-3′ and |
5′-CTCGGATAGAGAATCAACAGTCG-3′ | |
RD29A | 5′-GCCACATTCTGTTGAAGAGG-3′ and |
5′-CCCACTTTAGACCTAGTAGC-3′ | |
RD29B | 5-GATACCTTCCGACCAGATAGC-3′ and |
5′-GTTGGGACTATGGGGTTTTCG-3′ | |
UBQ | 5′-GATCTTTGCCGGAAAACAATTGGAGGATGGT-3′ and |
5′-CGACTTGTCATTAGAAAGAAAGAGATAACAGG-3′ |
Genes | Primers |
ABI1 | 5′-GCCATGTCGAGATCCATTGG-3′ and |
5′-GTGGCTGGGGATGCATCGTT-3′ | |
ABI2 | 5′-CAAGATCCATTGGCGATAGATACC-3′ and |
5′-CTTCCGGCGGAGAAAAGAGG-3′ | |
KAT1 | 5′-CTTCATGAAACTTAGAGGGCAAC-3′ and |
5′-GATAGAGAGAGCCAAAGTGG-3′ | |
KAT2 | 5′-AGGTTGCTCTGATGCAGATC-3′ and |
5′-CACAAAGGTCACGAATGCGG-3′ | |
DREB2A | 5′-GATGTGGATCAGAGTCACTTGG-3′ and |
5′-CTCGGATAGAGAATCAACAGTCG-3′ | |
RD29A | 5′-GCCACATTCTGTTGAAGAGG-3′ and |
5′-CCCACTTTAGACCTAGTAGC-3′ | |
RD29B | 5-GATACCTTCCGACCAGATAGC-3′ and |
5′-GTTGGGACTATGGGGTTTTCG-3′ | |
UBQ | 5′-GATCTTTGCCGGAAAACAATTGGAGGATGGT-3′ and |
5′-CGACTTGTCATTAGAAAGAAAGAGATAACAGG-3′ |
Chromatin immunoprecipitation assay
Chromatin immunoprecipitation (ChIP) analysis was carried out as described by Gendrel et al. (2005). Chromatin extracts were prepared from young leaves treated with formaldehyde. The chromatin was sheared to an average length of 500 bp by sonication and immunoprecipitated with specific antibodies including anti-acetylated-histone H3K9K14 (Catalogue no. 06-599, Upstate, Charlottesville, VA, USA), anti-dimethyl-histone H3K9 (Catalogue no. 07-441, Upstate, Charlottesville, VA, USA) and anti-trimethyl-histone H3K4 (Catalogue no. ab8580, Abcam). The DNA cross-linked to immunoprecipitated proteins was analysed by real-time PCR. To assess non-specific binding, the immunoprecipitation reaction was also performed in the absence of antibody.
DNA obtained from ChIP was used as the template for real-time PCR analysis. The following components were added to a reaction tube: 9 μl of iQ™ SYBR Green Supermix solution (Bio-Rad, Catalogue no. 170-8882), 5 μM of specific primers and 7.5 μl of a 75 times diluted DNA template. Relative H3K9K14 acetylation and H3K4 trimethylation were normalized to an internal control (Tubulin 2, At5g62690). Unlike ChIP using antibodies against acetylated-histone H3K9K14 and trimethyl-histone H3K14, Tubulin 2 sequences were not well amplified from ChIP samples using the antibody against dimethyl-histone H3K9. Relative H3K9 dimethylation levels were therefore normalized to the input DNA. Thermocycling conditions were 95 °C for 3 min followed by 40–50 cycles of 95 °C for 30 s, 60 °C for 35 s, and 72 °C for 35 s, with a melting curve detected at 95 °C for 1 min, 55 °C for 1 min and detected during the denature time from 55 °C to 95 °C. The primers used for real-time PCR analysis are listed in Table 2. The experiment was repeated three times and the statistical significance was calculated using the t test.
Genes | Primers |
ABI1-P | 5′-GATATTTTACCGGTGGTC-3′ and |
5′-GACGTGTCGTAGTCCGAGTT-3′ | |
ABI1-E | 5′-CTTGTCTTCCTAGCTTCTTC-3′ and |
5′-CCTTTACCCAATCTGATCCC-3′ | |
ABI2-P | 5′-CTAGTGGTCAGTGTAGATG-3′ and |
5′-GTGTAACATGCCATATGTCAC-3′ | |
ABI2-E | 5′-CAATCTAACATCTGTCTC-3′ and |
5′-GATTCACCGTTGCAATAGCC-3′ | |
KAT1-P | 5′-CTTAGAGTTTCACTTCCCATGC-3′ and |
5′-GACAGAATATGTAAGGGGCC-3′ | |
KAT1-E | 5′-CATAAAGGTCCCAACAGAAG-3′ and |
5′-CTGGCTCCAAGAGATGGTAG-3′ | |
KAT2-P | 5′-CAACGAACGGTCTATATAAG-3′ and |
5′-CAGATTCTTTACCTGGACAGC-3′ | |
KAT2-E | 5′-GCAGCATTAATGGTGCAC-3′ and |
5′-TCACGTTGTGGTTCTGAC-3′ | |
DREB2A-P | 5′-CACATCTAGTAAGTGTGATG-3′ and |
5′-GAATCTTCTCGAAACACGCT-3′ | |
DREB2A-E | 5′-CCAAGTTTAAGTTCTCTTGCC-3′ and |
5′-CTTCTACGGTCTCGTTATAC-3′ | |
RD29A-P | 5′-TACAATTCGAATGAGAAGGATGTGC-3′ and |
5′-GCTTTTTGGAACTCATGTCGGTAGT-3′ | |
RD29A-E | 5′-GACTTTGACGTCACACCACG-3′ and |
5′-GTGTGTGTTGAGTGGTGGTT-3′ | |
RD29B-P | 5′– CGTAATTTTCTAGATCCGTC-3′ and |
5′-CAATTCAGCTATCAACTGTG-3′ | |
RD29B-E | 5′-CCATATGTCATCGTTCTCTC-3′ and |
5′-GGATGGTGAATTCTGATTGG-3′ | |
Tubulin | 5′-ACAAACACAGAGAGGAGTGAGCA-3′ and |
5′-ACGCATCTTCGGTTGGATGAGTGA-3′ |
Genes | Primers |
ABI1-P | 5′-GATATTTTACCGGTGGTC-3′ and |
5′-GACGTGTCGTAGTCCGAGTT-3′ | |
ABI1-E | 5′-CTTGTCTTCCTAGCTTCTTC-3′ and |
5′-CCTTTACCCAATCTGATCCC-3′ | |
ABI2-P | 5′-CTAGTGGTCAGTGTAGATG-3′ and |
5′-GTGTAACATGCCATATGTCAC-3′ | |
ABI2-E | 5′-CAATCTAACATCTGTCTC-3′ and |
5′-GATTCACCGTTGCAATAGCC-3′ | |
KAT1-P | 5′-CTTAGAGTTTCACTTCCCATGC-3′ and |
5′-GACAGAATATGTAAGGGGCC-3′ | |
KAT1-E | 5′-CATAAAGGTCCCAACAGAAG-3′ and |
5′-CTGGCTCCAAGAGATGGTAG-3′ | |
KAT2-P | 5′-CAACGAACGGTCTATATAAG-3′ and |
5′-CAGATTCTTTACCTGGACAGC-3′ | |
KAT2-E | 5′-GCAGCATTAATGGTGCAC-3′ and |
5′-TCACGTTGTGGTTCTGAC-3′ | |
DREB2A-P | 5′-CACATCTAGTAAGTGTGATG-3′ and |
5′-GAATCTTCTCGAAACACGCT-3′ | |
DREB2A-E | 5′-CCAAGTTTAAGTTCTCTTGCC-3′ and |
5′-CTTCTACGGTCTCGTTATAC-3′ | |
RD29A-P | 5′-TACAATTCGAATGAGAAGGATGTGC-3′ and |
5′-GCTTTTTGGAACTCATGTCGGTAGT-3′ | |
RD29A-E | 5′-GACTTTGACGTCACACCACG-3′ and |
5′-GTGTGTGTTGAGTGGTGGTT-3′ | |
RD29B-P | 5′– CGTAATTTTCTAGATCCGTC-3′ and |
5′-CAATTCAGCTATCAACTGTG-3′ | |
RD29B-E | 5′-CCATATGTCATCGTTCTCTC-3′ and |
5′-GGATGGTGAATTCTGATTGG-3′ | |
Tubulin | 5′-ACAAACACAGAGAGGAGTGAGCA-3′ and |
5′-ACGCATCTTCGGTTGGATGAGTGA-3′ |
Genes | Primers |
ABI1-P | 5′-GATATTTTACCGGTGGTC-3′ and |
5′-GACGTGTCGTAGTCCGAGTT-3′ | |
ABI1-E | 5′-CTTGTCTTCCTAGCTTCTTC-3′ and |
5′-CCTTTACCCAATCTGATCCC-3′ | |
ABI2-P | 5′-CTAGTGGTCAGTGTAGATG-3′ and |
5′-GTGTAACATGCCATATGTCAC-3′ | |
ABI2-E | 5′-CAATCTAACATCTGTCTC-3′ and |
5′-GATTCACCGTTGCAATAGCC-3′ | |
KAT1-P | 5′-CTTAGAGTTTCACTTCCCATGC-3′ and |
5′-GACAGAATATGTAAGGGGCC-3′ | |
KAT1-E | 5′-CATAAAGGTCCCAACAGAAG-3′ and |
5′-CTGGCTCCAAGAGATGGTAG-3′ | |
KAT2-P | 5′-CAACGAACGGTCTATATAAG-3′ and |
5′-CAGATTCTTTACCTGGACAGC-3′ | |
KAT2-E | 5′-GCAGCATTAATGGTGCAC-3′ and |
5′-TCACGTTGTGGTTCTGAC-3′ | |
DREB2A-P | 5′-CACATCTAGTAAGTGTGATG-3′ and |
5′-GAATCTTCTCGAAACACGCT-3′ | |
DREB2A-E | 5′-CCAAGTTTAAGTTCTCTTGCC-3′ and |
5′-CTTCTACGGTCTCGTTATAC-3′ | |
RD29A-P | 5′-TACAATTCGAATGAGAAGGATGTGC-3′ and |
5′-GCTTTTTGGAACTCATGTCGGTAGT-3′ | |
RD29A-E | 5′-GACTTTGACGTCACACCACG-3′ and |
5′-GTGTGTGTTGAGTGGTGGTT-3′ | |
RD29B-P | 5′– CGTAATTTTCTAGATCCGTC-3′ and |
5′-CAATTCAGCTATCAACTGTG-3′ | |
RD29B-E | 5′-CCATATGTCATCGTTCTCTC-3′ and |
5′-GGATGGTGAATTCTGATTGG-3′ | |
Tubulin | 5′-ACAAACACAGAGAGGAGTGAGCA-3′ and |
5′-ACGCATCTTCGGTTGGATGAGTGA-3′ |
Genes | Primers |
ABI1-P | 5′-GATATTTTACCGGTGGTC-3′ and |
5′-GACGTGTCGTAGTCCGAGTT-3′ | |
ABI1-E | 5′-CTTGTCTTCCTAGCTTCTTC-3′ and |
5′-CCTTTACCCAATCTGATCCC-3′ | |
ABI2-P | 5′-CTAGTGGTCAGTGTAGATG-3′ and |
5′-GTGTAACATGCCATATGTCAC-3′ | |
ABI2-E | 5′-CAATCTAACATCTGTCTC-3′ and |
5′-GATTCACCGTTGCAATAGCC-3′ | |
KAT1-P | 5′-CTTAGAGTTTCACTTCCCATGC-3′ and |
5′-GACAGAATATGTAAGGGGCC-3′ | |
KAT1-E | 5′-CATAAAGGTCCCAACAGAAG-3′ and |
5′-CTGGCTCCAAGAGATGGTAG-3′ | |
KAT2-P | 5′-CAACGAACGGTCTATATAAG-3′ and |
5′-CAGATTCTTTACCTGGACAGC-3′ | |
KAT2-E | 5′-GCAGCATTAATGGTGCAC-3′ and |
5′-TCACGTTGTGGTTCTGAC-3′ | |
DREB2A-P | 5′-CACATCTAGTAAGTGTGATG-3′ and |
5′-GAATCTTCTCGAAACACGCT-3′ | |
DREB2A-E | 5′-CCAAGTTTAAGTTCTCTTGCC-3′ and |
5′-CTTCTACGGTCTCGTTATAC-3′ | |
RD29A-P | 5′-TACAATTCGAATGAGAAGGATGTGC-3′ and |
5′-GCTTTTTGGAACTCATGTCGGTAGT-3′ | |
RD29A-E | 5′-GACTTTGACGTCACACCACG-3′ and |
5′-GTGTGTGTTGAGTGGTGGTT-3′ | |
RD29B-P | 5′– CGTAATTTTCTAGATCCGTC-3′ and |
5′-CAATTCAGCTATCAACTGTG-3′ | |
RD29B-E | 5′-CCATATGTCATCGTTCTCTC-3′ and |
5′-GGATGGTGAATTCTGATTGG-3′ | |
Tubulin | 5′-ACAAACACAGAGAGGAGTGAGCA-3′ and |
5′-ACGCATCTTCGGTTGGATGAGTGA-3′ |
Results
axe1-5 and HDA6-RNAi plants were hypersensitive to ABA and NaCl
To investigate the function of HDA6 in ABA and abiotic stress response, the sensitivity of axe1-5 and a HDA6 RNA interference line, CS24039, to ABA and salt was analysed. axe1-5 is a splicing mutant which carries a point mutation in the HDA6 splicing site and it is in the Col background (Murfett et al., 2001; Probst et al., 2004). CS24039 is an HDA6 RNA-interference (RNAi) line in the Ws background (Wu et al., 2008). It was shown that the axe1-5 mutation resulted in two transcripts with different sizes, whereas the HDA6 transcript levels in the CS24039 plants were significantly reduced. To determine ABA sensitivity, seeds were germinated and grown on media containing various concentrations of ABA. As shown in Fig. 1A, axe1-5 and HDA6-RNAi seeds displayed hypersensitivity to ABA when compared with their corresponding wild-type. At 2 μM ABA, the percentage germination of Col and Ws wild-type seeds was 60% and 50%, respectively, whereas the percentage germination of axe1-5 and HDA6-RNAi seeds was about 20% only. When treated with NaCl, it was found that axe1-5 and HDA6-RNAi seeds also displayed a lower percentage germination compared with their corresponding wild type (Fig. 1B). At 200 mM NaCl, the percentage germination of Col and Ws wild-type seeds was about 50%, whereas the percentage germination of both axe1-5 and HDA6-RNAi seeds was only about 10%.
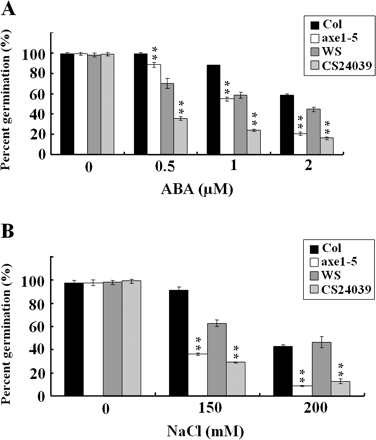
Seed germination rates of axe1-5 and HAD6-RNAi plants treated with ABA and NaCl. (A) Germination rates of 2-d-old Col wild-type, axe1-5, Ws wild-type, and HDA6-RNAi (CS24039) seedlings treated with ABA. Each data point represents the mean of a single experiment performed in triplicate (n ≥100). (B) Germination rates of 2-d-old Col wild-type, axe1-5, Ws wild-type, and HDA6-RNAi (CS24039) seedlings treated with NaCl. Each data point represents the mean of a single experiment performed in triplicate (n ≥100). Asterisks mark values that are significantly different from the wild type (t test, *P <0.05, **P <0.01).
It was tested further whether axe1-5 and HDA6-RNAi plants altered NaCl sensitivity at the seedling stage. As shown in Fig. 2, axe1-5 and HDA6-RNAi plants displayed a lower percentage survival in high salinity stress compared with their corresponding wild types. 5-d-old seedlings were transferred to a half-strength Murashige and Skoog medium containing 125 mM NaCl, and the percentage survival of seedlings was measured after 5 d. The percentage survival for Col and Ws wild-types was 68% and 98%, whereas the percentage survival for axe1-5 and HDA6-RNAi was 40% and 58%, respectively. It is interesting to note that the Col wild type had an equal germination rate upon exposure to salt but a lower survival rate when compared with the Ws wild type. This can be explained by the possibility that seed germination and vegetative growth are separate processes involving different molecular mechanisms.
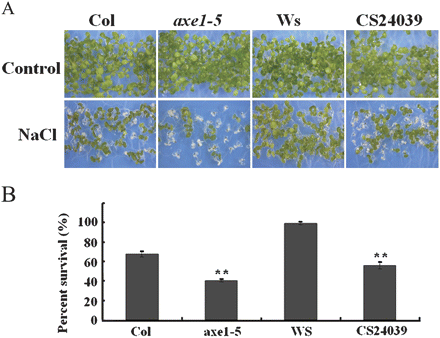
Phenotype comparison of axe1-5 and HAD6-RNAi plants in response to salt stress. (A) 5-d-old seedlings of Col wild-type, axe1-5, Ws wild-type, and HDA6-RNAi (CS24039) were transferred to a medium containing 125 mM NaCl, and the pictures were taken after 5 d. (B) 5-d-old seedlings were transferred to a medium containing 125 mM NaCl, and the percentage survival of seedlings was measured after 5 d. Each data point represents the mean of a single experiment performed in triplicate (n ≥100). Asterisks mark values that are significantly different from the wild type (t test, *P <0.05, **P <0.01).
axe1-5 and HDA6-RNAi plants displayed decreased expression of ABA and abiotic stress-responsive genes
The expression of ABA-responsive genes, such as ABA INSENSITIVE 1 (ABI1), ABI2, 3-KETO-ACYL-COA THIOLASE 1 (KAT1), KAT2, and RESPONSIVE TO DESSICATION 29B (RD29B) (Anderson et al., 1992; Yamaguchi-Shinozaki and Shinozaki, 1994; Merlot et al., 2001; Pilot et al., 2001), was determined in axe1-5 and HDA6-RNAi plants. Without ABA treatment, Col and Ws wild-types displayed similar expression patterns of ABI1, ABI2/RD29B, KAT1, and KAT2 as axe1-5 and HDA6-RNAi plants (Fig. 3). With ABA treatment, axe1-5 and HDA6-RNAi plants displayed a lower expression of ABA-responsive genes compared with their corresponding wild-types, suggesting that HDA6 is required for the full induction of ABA-responsive genes.
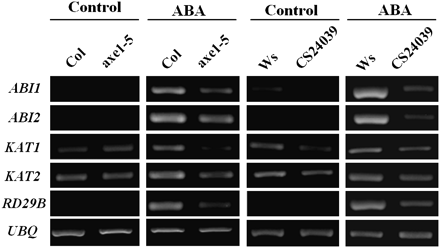
RT-PCR analysis of ABA-responsive gene expression in axe1-5 and HDA6-RNAi plants. 2-week-old Col wild-type, axe1-5, Ws wild-type, and HDA6-RNAi (CS24039) plants were treated with 100 μM of ABA for 3 h (Ws and CS24039) or 12 h (Col and axe1-5). Total RNA for RT-PCR analysis was isolated from leaf tissues. Ubiquitin (UBQ) was used as an internal control.
Genes that are involved in salt-stress signalling pathways, DRE-BINDING PROTEIN 2A (DREB2A), RD29A, and RD29B (Shinozaki and Yamaguchi-Shinozaki 2007), were also analysed in 2-week-old plants treated with 250 mM NaCl solution for 6 h. DREB2A is a salt-induced transcription factor and it regulates expression of RD29A and RD29B. Without salt treatment, no DREB2A, RD29A, and RD29B transcripts were detectable (Fig. 4). With salt treatment, DREB2A, RD29A, and RD29B were only slightly induced in axe1-5 and CS24039 plants. By comparison, the salt-induced expression of DREB2A, RD29A, and RD29B was more significant in Col and Ws wild-type plants. These data suggest that HDA6 is required for the full induction of salt-stress-responsive genes. The exposure to salt resulted in partial induction of DREB2A, RD29A, and RD29B genes in the axe1-5 mutant, whereas the induction in the RNAi mutant is lower compared with the axe1-5 mutant. It was shown that the axe1-5 mutation resulted in two transcripts with different sizes, whereas HDA6 transcript levels in the CS24039 plants were significantly reduced (Murfett et al., 2001; Wu et al., 2008). It is possible that axe1-5 is a leaky mutation and the two transcripts in axe1-5 may be partially functional.
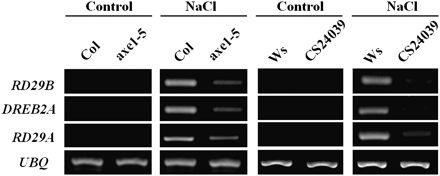
RT-PCR analysis of abiotic stress-responsive gene expression in axe1-5 and HDA6-RNAi plants. 2-week-old Col wild-type, axe1-5, Ws wild-type, and HDA6-RNAi (CS24039) plants were treated with 250 mM NaCl for 6 h. Total RNA for RT-PCR analysis was isolated from leaf tissues. Ubiquitin (UBQ) was used as an internal control.
ABA enriched H3K9K14 acetylation and H3K4 trimethylation but decreased H3K9 dimethylation of ABA-inducible genes
The gene activation markers, histone H3K9K14 acetylation and H3K4 trimethylation, as well as the gene repression marker, H3K9 dimethylation, were analysed in Col wild-type and axe1-5 plants. Previous studies indicated that histone acetylation and methylation can occur in both the proximal promoters and/or exons (Benhamed et al., 2006; Kim et al., 2008b; Wu et al., 2008). Histone modifications of the DNA sequences corresponding to the promoter (P) of about 400–1000 bp upstream of the translation starting site and the first exon (E) near the translation starting site of each target gene were therefore investigated. The positions and sizes of the fragments from promoter and exon areas are shown in Fig. 5. As shown in Fig. 6A, there was increased H3 acetylation in the ABI2 first exon (ABI2-E), KAT1 promoter (KAT1-P), KAT1-E, KAT2-E, RD29B-P, and RD29B-E in axe1-5 compared with the Col wild type, suggesting that the HDA6 mutation resulted in increased histone acetylation of some ABA-response genes. With ABA treatment, H3 acetylation levels of ABI2-E and RD29B-E were induced by ABA in the Col wild type, suggesting that ABA can enrich histone acetylation of some ABA response genes (Fig. 6A). However, ABA increased histone acetylation was not observed in axe1-5 mutants, indicating that HDA6 is required for the induction of hisotne acetylation by ABA. Increased histone acetylation in the ABA treatment suggested that ABA may enhance histone acetyltransferase activities or decrease histone deacetylase activities and HDA6 may be involved in this process directly or indirectly.
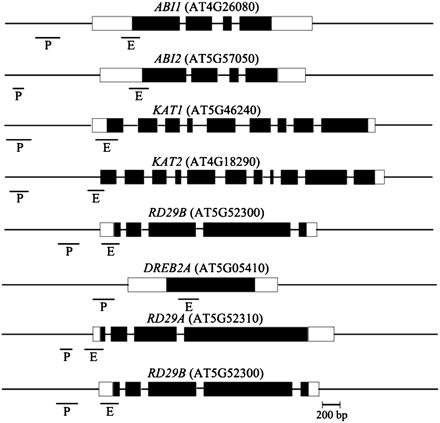
Relative positions of the amplified fragments of ABA- and salt-inducible genes in the ChIP assay. The positions and sizes of the amplified fragments from promoter (P) and exon (E) areas are indicated. The black boxes correspond to exons and white boxes to 5′ or 3′ UTR.
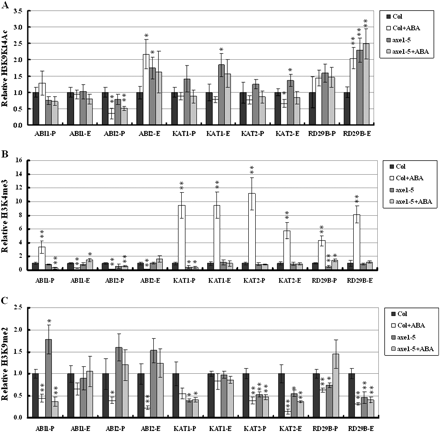
H3K9K14 acetylation, H3K4 trimethylation, and H3K9 dimethylation of ABA-inducible genes. Relative H3K9K14 acetylation and H3K4 trimethylation were determined by ChIP assays and normalized to an internal control (Tubulin 2). Relative H3K9 dimethylation levels were determined by ChIP assays and normalized to the input DNA. The value of Col wild type without treatment was arbitrarily given as 1. Data are the average of three biological replicates. Error bars represent standard errors. For ABA treatment, 2-week-old plants were treated with 100 μM ABA for 3 h. P indicates the promoter and E indicates the first exon. Asterisks mark values that are significantly different from the wild type without treatment (t test, *P <0.05, **P <0.01). The experiment was repeated three times with similar results.
Without ABA treatment, there was no difference in the H3K4 trimethylation of ABA response genes between Col wild type and axe1-5 mutants (Fig. 6B). H3K4 trimethyaltion of the ABI1-P, KAT1-P, KAT1-E, KAT2-P, KAT2-E, RD29B-P, and RD29B-E was significantly enriched by ABA in the Col wild type, but not in axe1-5. These results suggested that ABA can induce the H3K4 trimethylation in some ABA-responsive genes and HDA6 is required for this induction.
Without ABA treatment, there was increased H3K9 dimethylation in ABI1-P, ABI2-P, and ABI2E, but decreased H3K9 dimethylation in KAT1-P, KAT2-P, KAT2-E, and RD29B, in axe1-5 compared with Col wild type, suggesting that HDA6 effects on H3K9 dimethylation are gene-dependent (Fig. 6C). ABA could significantly decrease the H3K9 dimethylation of ABI1, ABI2, KAT2, and RD29B both in the promoter and first exon in the Col wild type (Fig. 5C). Similarly, ABA also decreased the H3K9 dimethylation of ABI1-P, ABI2-P, ABI2-E, and KAT2-E in axe1-5 plants (Fig. 6C). These results suggested that ABA can decrease the H3K9 dimethylation of ABA-responsive genes.
Salt enriched H3K9K14 acetylation and H3K4 trimethylation but decreased H3K9 dimethylation of salt-inducible genes
The effect of NaCl on histone H3K9K14 acetylation, H3K4 trimethylation, and H3K9 dimethylation of abiotic stress-inducible genes, DREB2A, RD29A, and RD29B, were also analysed. When treated with NaCl, there was increased H3 acetylation in the DREB2A-P, DREB2A-E, RD29A-P, RD29B-P, and RD29B-E in Col wild type (Fig. 7A), suggesting that NaCl can induce H3 acetylation of salt stress-responsive genes. By comparison, NaCl could not induce H3 acetylation of DREB2A, RD29A, and RD29B in axe1-5 plants, indicating that HDA6 is required for histone acetylation of salt-responsive genes.
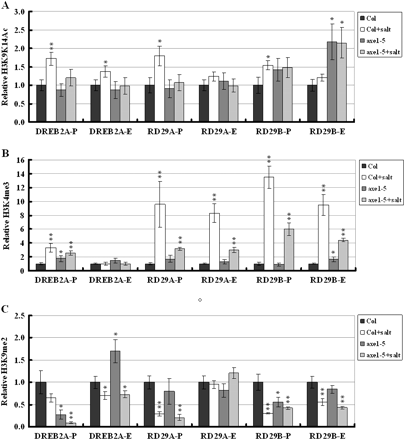
H3K9K14 acetylation, H3K4 trimethylation, and H3K9 dimethylation of abiotic stress-inducible genes. Relative H3K9K14 acetylation and H3K4 trimethylation were determined by ChIP assays and normalized to an internal control (Tubulin 2). Relative H3K9 dimethylation levels were determined by ChIP assays and normalized to the input DNA. The value of Col wild type without treatment was arbitrarily given as 1. Data are the average of three biological replicates. Error bars represent standard errors. For NaCl treatment, 2-week-old plants were treated with 250 mM NaCl for 6 h. P indicates the promoter and E indicates the first exon. Asterisks mark values that are significantly different from the wild type without treatment (t test, *P <0.05, **P <0.01). The experiment was repeated three times with similar results.
NaCl also enriched H3K4 trimethyaltion of DREB2A-P, RD29A-P, RD29A-E, RD29B-P, and RD29B-E in the Col wild type (Fig. 7B). Compared with the wild type, the increase of H3K4 trimethyaltion was lower in axe1-5. These results suggest that NaCl can induce H3K4 trimethylation in abiotic stress-responsive genes and HDA6 is partially required for this induction. When treated with NaCl, H3K9 dimethylation of DREB2A-P, DREB2A-E, RD29A-P, RD29B-P, and RD29B-E was decreased in Col wild-type as well as axe1-5 plants (Fig. 7C), suggesting that NaCl can decrease the H3K9 dimethylation of abiotic stress-responsive genes. The observation of that NaCl treatment decreased dimethylation of stress-responsive genes in both the wild type and axe1-5 mutants suggests that the hda6 mutation does not affect this decrease.
Discussion
Regulation of abiotic stress-responsive genes expression by dynamic histone modification could be an important mechanism for plants to respond to abiotic stress. Recent studies indicated that histone acetylation and deacetylation are involved in the plant's response to abiotic stress. In Arabidopsis, mutations in GCN5 and ADA2 that encode the components of histone acetyltransferase complexes affected the expression of the cold-regulated genes and plant cold tolerance (Vlachonasios et al., 2003). It was suggested that histone acetyltransferases may be recruited through transcription factors, such as CBF1, to cold-induced genes, through multiprotein complexes similar to those found in other eukaryotes (Stockinger et al., 2001). Overexpression of HD2C, a HD2-type histone deacetylase, resulted in a higher germination rate and longer root length compared with wild-type when treated with ABA (Sridha and Wu, 2006).
In this study, the function of HDA6 in the ABA and abiotic stress response was investigated. HDA6 mutants displayed hypersensitivity to ABA in seed germination. Furthermore, expression of ABA-responsive genes was decreased in axe1-5 and HDA6-RNAi plants. axe1-5 and HDA6-RNAi seeds also displayed higher sensitivity to NaCl in seed germination and lower percentage survival rates under salt stress. Gene expression analysis indicated that HDA6 is required for the full induction of salt-responsive genes. These results suggest that HDA6 is involved in the ABA and abiotic stress response.
The class I RPD3 family of Arabidopsis histone deacetylases have four members, HDA6, HDA7, HDA9, and HDA19 (Pandey et al., 2002). Expression levels of HDA6 and HDA19 are higher than these of HDA7 and HDA9, in different developmental stages (Wu et al., 2008). A recent study suggested that HDA19 may act in a protein complex with AtERF7, a transcription repressor (Song et al., 2005), to regulate abiotic stress response genes. It was found the AtERF7 interacts with the Arabidopsis homologue of a human global corepressor of transcription, AtSin3 (Silverstein and Ekwall, 2005), which, in turn, may interact with HDA19. It was proposed that AtERF7, AtSin3, and HDA19 can form a transcriptional repressor complex to regulate the ABA and drought response in Arabidopsis (Song et al., 2005). Reduced expression of AtERF7 and AtSin3 by RNA-interference caused ABA hypersensitivity including root development repression and delayed germination, a phenotype similar to axe1-5 and HDA6-RNAi plants. It remains to be determined whether HDA6 and HDA19 play a redundant role in the ABA and abiotic stress response and whether HDA6, like HDA19, may also form a similar transcriptional complex to regulate gene expression in Arabidopsis.
The large number of possible histone modification patterns allows highly specific and complex signalling mechanisms, thus it is not surprising that particular histone modification patterns may be associated with specific transcriptional effects. For example, H3K9 acetylation, H3K14 acetylation, and H3K4 trimethylation are regarded as positive markers of histone modification associated with gene activation, whereas H3K9 deacetylation, H3K14 deacetylation, and H3K9 dimethylation are negative markers (Chen and Tian, 2007). Kurdistani et al. (2004) analysed the genome-wide acetylation patterns of 11 lysines throughout the core histones in yeast cells. By clustering genes with similar acetylation patterns, it was found that functionally related genes are similarly acetylated (Kurdistani et al. 2004). This suggests that functionally related gene groups are regulated co-ordinately through histone modifications. In addition, correlating acetylation clusters with stress-induced expression levels suggests that many stress-induced genes belong to the same acetylation cluster, indicating the co-ordinated regulation of an assorted group of genes in response to a particular stress in yeast cells.
In this study, it was found that ABA can enrich H3K4 trimethylation but decrease H3K9 dimethylation of ABI1, ABI2, KAT1, KAT2, and RD29B in Arabidopsis. Similarly, salt stress can enrich H3K4 trimethylation but decrease H3K9 dimethylation of DREB2A, RD29A, and RD29B. In addition, H3 acetylation of ABI1, ABI2, and RD29B was enriched by ABA, while H3 acetylation of DREB2A, RD29A, and RD29B was enriched by salt stress. An increase in the gene activation markers, H3 acetylation and H3K4 trimethylation, as well as a decrease in the gene repression marker, H3K9 dimethylation, was consistent with transcriptional activation. Recently, it was found that the H3K9 acetylation and H3K4 trimethylation of the drought-responsive genes, RD29A, RD29B, RD20, and RAP2.4 were induced by drought treatment (Kim et al., 2008b). These results suggested that the induction of the ABA and abiotic stress-responsive genes is associated with the changes of histone modifications. More recently, Boyko et al. (2010) suggest that the progeny of plants exposed to salt shows hypermethylation of genes involved in histone regulation, such as SUVH2, SUVH5, SUVH6, FLD, and UBP26; FLD has the histone deacetylation domain, and thus it can be suggested that hypermethylation of the FLD promoter would result in lower FLD expression and thus in the increase in histone acetylation of the target histones.
In the HDA6 mutant, axe1-5, ABA- and salt-induced changes of H3K4 trimethylation were abolished or decreased, suggesting that HDA6 is required for H3K4 trimethylation associated with gene activation. Since both trimethyl H3K4 and acetyl H3K9K14 marks are often associated with gene activation, the repressive function of HDA6 may be enhanced by removing both marks through a synergistic interplay between histone demethylase and deacetylase enzymes. Similar cross-talks between histone deacetylation and demethylation have previously been reported to modulate gene expression in mammalian cells (Shi et al., 2005; Lee et al., 2006). In mammalian cells, the histone demethylase, LSD1, is an integral component of histone deacetylase corepressor complexes in which histone deacetylases and LSD1 may co-operate to remove activating acetyl and methyl histone modifications (Shi et al., 2005; Lee et al., 2006). Consistent with this model, it was found that the enzymatic activities of histone deacetylases and LSD1 are closely linked, as histone deacetylase inhibitors diminish histone demethylation activity and the abrogation of LSD1 activity decreases the deacetylation activity (Lee et al., 2006). These observations suggest that histone acetylation and methylation may cross-talk and mutually affect each other to regulate gene activities.
This work is supported by grants from the National Science Council of Taiwan (96-2311-B-002-013-MY2 and 97-2311-B-002-004-MY3) and the National Taiwan University (97R0066-36), and grants from the Natural Science Foundation of China (No. 30971564 and No. 90919038).
Comments