-
PDF
- Split View
-
Views
-
Cite
Cite
Mayumi Tabuchi, Tomomi Abiko, Tomoyuki Yamaya, Assimilation of ammonium ions and reutilization of nitrogen in rice (Oryza sativa L.), Journal of Experimental Botany, Volume 58, Issue 9, July 2007, Pages 2319–2327, https://doi.org/10.1093/jxb/erm016
- Share Icon Share
Abstract
A major source of inorganic nitrogen for rice plants grown in paddy soil is ammonium ions. The ammonium ions are actively taken up by the roots via ammonium transporters and subsequently assimilated into the amide residue of glutamine (Gln) by the reaction of glutamine synthetase (GS) in the roots. The Gln is converted into glutamate (Glu), which is a central amino acid for the synthesis of a number of amino acids, by the reaction of glutamate synthase (GOGAT). Although a small gene family for both GS and GOGAT is present in rice, ammonium-dependent and cell type-specific expression suggest that cytosolic GS1;2 and plastidic NADH-GOGAT1 are responsible for the primary assimilation of ammonium ions in the roots. In the plant top, approximately 80% of the total nitrogen in the panicle is remobilized through the phloem from senescing organs. Since the major form of nitrogen in the phloem sap is Gln, GS in the senescing organs and GOGAT in developing organs are important for nitrogen remobilization and reutilization, respectively. Recent work with a knock-out mutant of rice clearly showed that GS1;1 is responsible for this process. Overexpression studies together with age- and cell type-specific expression strongly suggest that NADH-GOGAT1 is important for the reutilization of transported Gln in developing organs. The overall process of nitrogen utilization within the plant is discussed.
Introduction
Nitrogen is quantitatively the most essential nutrient for plants and a major limiting factor in plant productivity. The major form of inorganic nitrogen that is available for the growth of rice plants in paddy soil is the ammonium ion (NH4+). The ammonia (NH3) gas molecule is a weak base that protonates rapidly to form the NH4+ ion with a dissociation constant of 10−9.25 (Kleiner, 1981). According to the equation proposed by Freney et al. (1985), 99.4% of the total ammonia in water is in the protonated form at pH 7.0 and 25 °C, and the NH4+ ion is the major molecular species in paddy soil, as well as in most of the compartments of rice plants. On the other hand, excess NH4+ can apparently be toxic to some plants (Kronzucker et al., 2001). Thus, efficient NH4+ transport and subsequent assimilation systems should be highly regulated within the roots. The major forms of nitrogen in the xylem sap of rice plants are Gln and Asn (Fukumorita and Chino, 1982). Real-time monitoring of NH4+ transport by the positron emitting tracer imaging system (PETIS) showed that the signals of 13N taken up by rice roots were detected in the basal part of shoots within a short period, but the translocation was completely inhibited by methionine sulphoximine, an inhibitor of glutamine synthetase (GS) (Kiyomiya et al., 2001). These results strongly suggest that most of the NH4+ taken up by the roots can be assimilated within the organ by the reaction catalysed by GS. Since the discovery of the GS/glutamate synthetase (GOGAT) cycle by Lea and Miflin (1974), it is now well established that this cycle is the only route for the primary assimilation of NH4+ in plants grown under normal conditions (Ireland and Lea, 1999; Lea and Miflin, 2003).
In the top part of japonica rice, approximately 80% of the total nitrogen in the panicle arises from remobilization through the phloem from senescing organs (Mae and Ohira, 1981). The major forms of nitrogen in the phloem sap are again Gln and Asn (Hayashi and Chino, 1990). The synthesis of Gln in senescing organs is the essential step for this dynamic nitrogen recycling, as Asn is synthesized by the transfer of an amide group from Gln (Lea et al., 2007). In developing sink organs, the remobilized Gln is reutilized for many biosynthetic reactions, via the GS/GOGAT pathway (Lea and Miflin, 2003), which is mostly responsible for the metabolism of Gln in rice (Tobin and Yamaya, 2001).
As in other plants (Ireland and Lea, 1999; Yamaya and Oaks, 2004), a small gene family has been identified that encodes GS1 (Ishiyama et al., 2004b; Tabuchi et al., 2005) and NADH-GOGAT (M Tabuchi et al., unpublished results) in rice, in addition to the major species of GS2 and ferredoxin (Fd)-GOGAT in the chloroplasts of green tissues of this plant. These are OsGS1;1, OsGS1;2, and OsGS1;3, and OsNADH-GOGAT1 and OsNADH-GOGAT2. It has been shown that the expression profile of each gene was different in terms of age and tissue specificity, and response to NH4+, suggesting that each gene product apparently has a distinct function in rice. As discussed in earlier reviews, the major function of GS2 and Fd-GOGAT in chloroplasts is in photorespiratory nitrogen metabolism (Lea and Miflin, 2003), and other GS/GOGAT species are important for normal growth and development (Yamaya et al., 2002; Yamaya and Oaks, 2004). This is because mutants lacking either GS2 or Fd-GOGAT (Wallsgrove et al., 1987; Kendall et al., 1986; Leegood et al., 1995) were able to grow normally under non-photorespiratory conditions.
In this review, the functions of GS1 and NADH-GOGAT isoenzymes in rice are discussed. Because some of these genes are up- or down-regulated by exogenous NH4+ supply, possible mechanisms of the regulation are also discussed.
GS1;2 and NADH-GOGAT1 could be key players in the assimilation of NH4+ taken up by rice roots
Rice is able to take up NH4+ ions when grown in a paddy field, through the action of ammonium transporters (AMTs). Ninnemann et al. (1994) first identified a gene encoding a high-affinity AMT from Arabidopsis thaliana (AtAMT1;1) using functional complementation of a yeast mutant defective in NH4+ uptake. Since then, the isolation of AMT1 homologues from Arabidopsis (Gazzarrini et al., 1999), tomato (von Wirén et al., 2000), and rice (Sonoda et al., 2003a) has shown that the AMT1 family in plants consists of at least three to five members. In Arabidopsis (Sohlenkamp et al., 2000) and rice (Suenaga et al., 2003), several genes with high homology to bacterial AMT and yeast AMTs (methylammonium permease: MEP) were also identified (Lea and Azevedo, 2006). Expression of OsAMT genes in rice showed distinct profiles, i.e. root-specific and NH4+-inducible expression for OsAMT1;2, constitutive expression in roots and shoots for OsAMT1;1 and OsAMT2;1, and root-specific and NH4+-derepressed expression for OsAMT1;3. The up-regulation of OsAMT1;2 gene expression occurred as rapidly as 30 min following the supply of NH4+ ions, when NH4+-responsive expression was observed in specific cell types in the root tips, for example exodermis, sclerenchyma, endodermis, and pericycle (Sonoda et al., 2003a). There are two Casparian strips in rice roots, (i) between the exodermis and sclerenchyma and (ii) in the endodermis, requiring a symplastic system for solute transport in these regions (Morita et al., 1996). The AMT1;2 protein could be responsible for cell-to-cell transport of NH4+ ions. Pharmacological studies suggested that the up-regulation of OsAMT1;2 gene expression was caused by Gln rather than NH4+ ions (Sonoda et al., 2003b), as in the case of up-regulation of OsNADH-GOGAT1 in rice roots (Hirose et al., 1997). It seems likely that OsAMT1;2 mainly functions in NH4+ uptake from NH4+-enriched soils. On the other hand, OsAMT1;3, which is down-regulated by NH4+, may be present to support OsAMT1;1 in taking up NH4+ ions, when the availability of NH4+ in the soil is low.
Among the three GS1 genes in rice, OsGS1;1 was expressed in all organs, i.e. root, leaf blade, leaf sheath, and spikelet, with higher expression in the leaf blade during the vegetative stage of growth of the plants (Tabuchi et al., 2005). OsGS1;2 transcripts were also detected in all organs, with higher expression in the root following the supply of NH4+ at the seedling stage, while OsGS1;3 was specifically expressed in the spikelet (Fig. 1). The OsGS1;1 and OsGS1;2 transcripts showed reciprocal responses to NH4+ supply in the surface cell layers of roots (Ishiyama et al., 2004a). Transcripts of OsGS1;1 accumulated in the dermatogen, epidermis, and exodermis under NH4+-limited condition. In contrast, OsGS1;2 was abundantly expressed in the same cell layers under NH4+-sufficient conditions, replenishing the loss of OsGS1;1, 3–6 h after the supply of NH4+ ions. Within the central cylinder of the root elongation zone, both genes were up-regulated by NH4+. The kinetic properties of OsGS1;1 and OsGS1;2 that had been purified from the recombinant proteins overexpressed Escherichia coli showed that both enzymes could be classified as high-affinity subtypes for NH4+ ions (Ishiyama et al., 2004a) with relatively high Vmax values, as compared with the major high-affinity isoenzyme in Arabidopsis (Ishiyama et al., 2004b). Low-affinity forms of GS1 seen in Arabidopsis were absent in rice roots (Table 1).
Isoenzyme | Km | Vmax (nkat mg−1 protein) | ||||
Glu (mM) | NH4+ (μM) | ATP (μM) | Glu | NH4+ | ATP) | |
OsGS1;1 | 1.9±0.0 | 27±5 | 450±20 | 190.0±12.3 | 186.3±18.4 | 170.2±15.1 |
OsGS1;2 | 2.1±0.0 | 73±2 | 530±80 | 97.4±9.0 | 98.1±1.7 | 109.1±14.8 |
AtGS1;1 | 1.1±0.4 | <10 | 300±20 | 29.3±1.6 | 27.4±0.7 | 21.4±0.4 |
AtGS1;2 | 3.8±0.2 | 2450±150 | 1100±140 | 65.7±0.2 | 65.7±1.1 | 66.6±4.4 |
AtGS1;3 | 3.9±0.1 | 1210±40 | 850±30 | 162±24 | 93.9±10 | 100.0±0.04 |
AtGS1;4 | 0.6±0.1 | 48±6 | 400±50 | 79.2±1 | 65.7±1.5 | 73.9±4.2 |
Isoenzyme | Km | Vmax (nkat mg−1 protein) | ||||
Glu (mM) | NH4+ (μM) | ATP (μM) | Glu | NH4+ | ATP) | |
OsGS1;1 | 1.9±0.0 | 27±5 | 450±20 | 190.0±12.3 | 186.3±18.4 | 170.2±15.1 |
OsGS1;2 | 2.1±0.0 | 73±2 | 530±80 | 97.4±9.0 | 98.1±1.7 | 109.1±14.8 |
AtGS1;1 | 1.1±0.4 | <10 | 300±20 | 29.3±1.6 | 27.4±0.7 | 21.4±0.4 |
AtGS1;2 | 3.8±0.2 | 2450±150 | 1100±140 | 65.7±0.2 | 65.7±1.1 | 66.6±4.4 |
AtGS1;3 | 3.9±0.1 | 1210±40 | 850±30 | 162±24 | 93.9±10 | 100.0±0.04 |
AtGS1;4 | 0.6±0.1 | 48±6 | 400±50 | 79.2±1 | 65.7±1.5 | 73.9±4.2 |
Results for rice (OsGS1;1 and 1;2) and Arabidopsis thaliana (AtGS1;1 to 1;4) were adapted from Ishiyama et al. (2004a) and Ishiyama et al. (2004b), respectively. Purified recombinant enzymes were used for the assay. Kinetic values for the GS synthetic activities were determined by Eadie–Hofstee equations. One katal of enzyme activity is defined as 1 mol of Gln synthesized per second at 30 °C. Data are means ±SE (n=3).
Isoenzyme | Km | Vmax (nkat mg−1 protein) | ||||
Glu (mM) | NH4+ (μM) | ATP (μM) | Glu | NH4+ | ATP) | |
OsGS1;1 | 1.9±0.0 | 27±5 | 450±20 | 190.0±12.3 | 186.3±18.4 | 170.2±15.1 |
OsGS1;2 | 2.1±0.0 | 73±2 | 530±80 | 97.4±9.0 | 98.1±1.7 | 109.1±14.8 |
AtGS1;1 | 1.1±0.4 | <10 | 300±20 | 29.3±1.6 | 27.4±0.7 | 21.4±0.4 |
AtGS1;2 | 3.8±0.2 | 2450±150 | 1100±140 | 65.7±0.2 | 65.7±1.1 | 66.6±4.4 |
AtGS1;3 | 3.9±0.1 | 1210±40 | 850±30 | 162±24 | 93.9±10 | 100.0±0.04 |
AtGS1;4 | 0.6±0.1 | 48±6 | 400±50 | 79.2±1 | 65.7±1.5 | 73.9±4.2 |
Isoenzyme | Km | Vmax (nkat mg−1 protein) | ||||
Glu (mM) | NH4+ (μM) | ATP (μM) | Glu | NH4+ | ATP) | |
OsGS1;1 | 1.9±0.0 | 27±5 | 450±20 | 190.0±12.3 | 186.3±18.4 | 170.2±15.1 |
OsGS1;2 | 2.1±0.0 | 73±2 | 530±80 | 97.4±9.0 | 98.1±1.7 | 109.1±14.8 |
AtGS1;1 | 1.1±0.4 | <10 | 300±20 | 29.3±1.6 | 27.4±0.7 | 21.4±0.4 |
AtGS1;2 | 3.8±0.2 | 2450±150 | 1100±140 | 65.7±0.2 | 65.7±1.1 | 66.6±4.4 |
AtGS1;3 | 3.9±0.1 | 1210±40 | 850±30 | 162±24 | 93.9±10 | 100.0±0.04 |
AtGS1;4 | 0.6±0.1 | 48±6 | 400±50 | 79.2±1 | 65.7±1.5 | 73.9±4.2 |
Results for rice (OsGS1;1 and 1;2) and Arabidopsis thaliana (AtGS1;1 to 1;4) were adapted from Ishiyama et al. (2004a) and Ishiyama et al. (2004b), respectively. Purified recombinant enzymes were used for the assay. Kinetic values for the GS synthetic activities were determined by Eadie–Hofstee equations. One katal of enzyme activity is defined as 1 mol of Gln synthesized per second at 30 °C. Data are means ±SE (n=3).
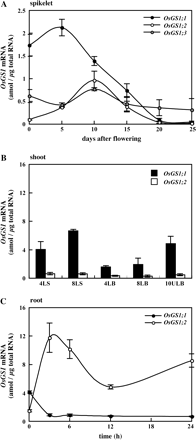
Accumulation of OsGS1;1 (filled circles or bars), OsGS1;2 (open circles or bars), and OsGS1;3 (grey circles) mRNAs in (A) spikelet during ripening, (B) in leaf blade (LB), leaf sheath (LS), and unexpanded premature leaf blade (ULB) at the fourth, eighth, or tenth leaf position from the bottom, and (C) in roots following the supply of 1 mM NH4Cl for 24 h in wild-type japonica rice plants. Quantitative real-time PCR was performed using gene-specific primers for OsGS1;1 (Ishiyama et al., 2004a) and OsGS1;2 and OsGS1;3 (Tabuchi et al., 2005), respectively. Means of independent triplicate samples and SD values (n=3) are indicated. Note that the units of the y-axis of (A) are not the same as those of (B) and (C).
To provide Glu for the GS1 reaction, GOGAT is required. In addition to the known gene (OsNADH-GOGAT1) for NADH-GOGAT in rice (Goto et al., 1998), another gene, OsNADH-GOGAT2 (accession number: AB274818), has recently been identified. As shown in Fig. 2, OsNADH-GOGAT1 was mainly expressed in developing tissues, such as the root-tip following the supply of NH4+, and in the premature leaf blade and spikelet at the early stage of ripening. In contrast, OsNADH-GOGAT2 was mainly expressed in the mature leaf blade and sheath. Thus, previous results obtained with roots (Ishiyama et al., 1998,, 2003) and developing organs (Hayakawa et al., 1994) are mostly explained by the behaviour of OsNADH-GOGAT1. In the roots, NADH-GOGAT1 protein was detected in plastids (Hayakawa et al., 1999) and accumulated in the dermatogen, epidermis, and exodermis cells following the supply of NH4+ ions (Ishiyama et al., 2003). Corresponding OsNADH-GOGAT1 transcripts started to accumulate in the cells of the root surface 3–6 h after the supply of NH4+ ions, and the protein reached a maximal level after 12–24 h. Fd-GOGAT protein, another GOGAT species, was detected in the meristem zone, central cylinder, and cortex of the roots. Double-labelling studies clearly showed that there was little overlap in the distribution of the Fd- and NADH-GOGAT proteins in the rice roots. To complete the NADH-GOGAT reaction, 2-oxoglutarate (2-OG) needs to be generated in the cells where NADH-GOGAT is localized. Recent observations suggest that mitochondrial NAD-isocitrate dehydrogenase is a candidate to generate 2-OG for this reaction (Abiko et al., 2005).

Accumulation of OsNADH-GOGAT1 (filled circles or bars) and OsNADH-GOGAT2 (open circles or bars) mRNAs in (A) spikelet during ripening, (B) in leaf blade (LB), leaf sheath (LS), and unexpanded premature leaf blade (ULB) at the fourth, eighth, or tenth leaf position from the bottom, and (C) in roots following the supply of 1 mM NH4Cl for 24 h in wild-type japonica rice plants. Quantitative real-time PCR was performed using gene-specific primers as follows: NADH-GOGAT1cDNAF6481-6502 5′-GTGCAGCCTGTTGCAGCATAAA-3′ and NADH-GOGAT1cDNAR6714-6694 5′-CGGCATTTCACCATGCAAATC-3′ for OsNADH-GOGAT1 mRNA; and NADH-GOGAT2cDNAF6480-6507 5′-CCTGTCGAAGGATGATGAAGGTGAAACC-3′ and NADH-GOGAT2cDNAR6631-6606 5′-TGCATGGCCCTACTATCTTCGCATCA-3′ for OsNADH-GOGAT2 mRNA. Means of independent triplicate samples and SD values (n=3) are indicated. Note that the units of the y-axis of (A) are not the same as those of (B) and (C).
Thus, OsAMT1;2, OsGS1;2, and OsNADH-GOGAT1 are rapidly and sequentially expressed in the two cell layers of the root surface, epidermal and exodermal cells, following the supply of NH4+ ions. Because the Casparian strip is located between the second and third cell layers (Morita et al., 1996), most of the NH4+ taken up by the roots is probably assimilated within the epidermal and exodermal cells. When incomplete assimilation of NH4+ ions occurs, as in the case of excess supply of the ions, and when NH4+ ions originate from other reactions, such as from phenylalanine ammonia-lyase required for secondary metabolism (Sakurai et al., 2001), GS1;1 or GS1;2 coupled with Fd-GOGAT (Ishiyama et al., 2003) may be responsible for the assimilation of NH4+ ions. The reduced form of nitrogen is then transported to the top of the plant through the xylem stream. A structure and schematic model for the localization of AMT1;2 and the GS and GOGAT assimilatory isoenzymes in different cell types of rice roots are presented in Fig. 3.
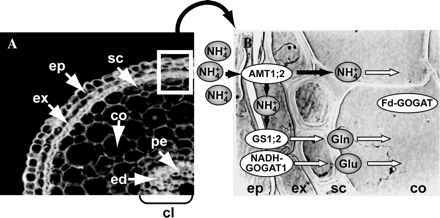
(A) Cross-section of seminal roots and (B) schematic cellular compartmentation of AMT1;2 and NH4+-assimilatory isoenzymes showing nitrogen flow in rice roots. Casparian strips are located between the exodermis and sclerenchyma and in the endodermis, respectively. Abbreviations: co, cortex; cl, central cylinder; ed, endodermis; ep, epidermis; ex, exodermis, pe, pericycle; and sc, sclerenchyma. Open arrows in (B) indicate that the corresponding transporters or metabolic flow are not clearly understood.
Gln signalling in rice
Expression of OsAMT1;1, OsAMT1;2, OsGS1;1, OsGS1;2, and OsNADH-GOGAT1 was up- or down-regulated by exogenous NH4+ ions, respectively, as mentioned above. Pharmacological studies suggested that Gln, but not NH4+ ions, could be the real signalling molecule involved in the regulation of expression of at least the OsAMT1 genes (Sonoda et al., 2003b) and OsNADH-GOGAT1 (Hirose et al., 1997). Using rice cell cultures, it was suggested that phosphorylation/de-phosphorylation of an unknown protein could be involved in the Gln-mediated up-regulation of OsNADH-GOGAT1 (Hirose and Yamaya, 1999). In other plants, Gln also regulates gene expression, i.e. AtAMT1;1 (Rawat et al., 1999), asparagine synthetase (Lam et al., 1998; Lea et al., 2007), GS1 isoenzymes (Oliveira and Coruzzi, 1999), and nitrate reductase in tobacco leaves (Vincentz et al., 1993). In contrast to nitrate signalling (Sakakibara, 2006), very little is clearly understood about Gln signalling in plants.
In enteric bacteria, the PII-uridylyltransferase/uridylyl-removing enzyme (GLND) acts as a sensor of Gln and the PII protein encoded by GLNB acts as both a sensor of 2-OG and a transmitter for Gln signalling from GLND (Arcondéguy et al., 2001). PII is reversibly uridylylated by GLND in response to Gln/2-OG status. These signals are transmitted via a two-component system and, consequently, regulate the expression of the GS gene (Arcondéguy et al., 2001). Taking into account this bacterial system, efforts have been made to identify a GLND-like protein as a Gln sensor and a PII-like protein as a Gln signal transmitter in the plant kingdom. In the genome databases of Arabidopsis and rice, there is no gene encoding a protein homologous to the entire polypeptide of bacterial GLND. However, PII-like proteins have been isolated from both Arabidopsis (Hsieh et al., 1998) and rice (Sugiyama et al., 2004) although they contain no uridylylation site. Thus, plants probably have a different Gln signalling system from the bacterial GLND-PII system (Sugiyama et al., 2004; Ferario-Méry et al., 2005).
Recently, ACT domain repeat proteins (ACRs) have been isolated from Arabidopsis thaliana (Hsieh and Goodman, 2002) and rice (Hayakawa et al., 2006). The term ‘ACT’ was derived from three bacterial enzymes/genes (Aravind and Koonin, 1999), i.e. aspartate kinase, chorismate mutase, and prephenate dehydrogenase (tyrA). It was predicted that ACT domains function primarily as amino acid-binding sites for allosteric regulation of these enzyme activities. In bacterial GLND, there are two ACT domains at the C-terminus, which may serve as a Gln-binding site (Chipman and Shaanan, 2001). Discovery of ACRs in plants would be the first step toward understanding the mechanism of Gln signal perception, but evidence is required on the interaction between Gln and ACR. Plant PII-like proteins located in chloroplasts (Hsieh et al., 1998; Sugiyama et al., 2004) and Arabidopsis PII-like proteins have been shown to bind 2-OG (Smith et al., 2003). However, the function of PII in the Gln/2-OG signalling system is still unclear, because the phenotypes of transgenic Arabidopsis overexpressing the PII-like protein gene, GLB1 (Hsieh et al., 1998), and knock-out mutants of this gene (Ferario-Méry et al., 2005) were not greatly altered. The PII-like protein in rice interacted with N-acetylglutamate kinase1 in chloroplasts (Sugiyama et al., 2004) and the same interaction was confirmed in Arabidopsis (Chen et al., 2006). However, the physiological role of this interaction is still unknown.
GS1;1 and NADH-GOGAT1 are important in the remobilization and reutilization of nitrogen in the top part of rice plants during senescence
We have proposed a hypothesis that during nitrogen remobilization GS1;1 is important for the synthesis of Gln, the major form of reduced nitrogen in the phloem sap (Hayashi and Chino, 1990), while NADH-GOGAT1 is important in developing sink organs in the reutilization of Gln in rice (Hayakawa et al., 1994). This hypothesis is mainly based on localization studies. The GS1 protein was detected in companion cells and vascular parenchyma cells of senescing leaf blades of rice (Kamachi et al., 1992; Sakurai et al., 1996) and wheat plants (Kichey et al., 2005), while the NADH-GOGAT protein accumulated in vascular parenchyma cells and Mestome sheath cells of developing young leaves and dorsal vascular cells of developing grains (Hayakawa et al., 1994). A schematic model of nitrogen remobilization and structure of the leaf blade and spikelet is shown in Fig. 4. An investigation into cell type expression of each specific gene for individual GS1 and NADH-GOGAT isoenzymes is now being conducted using promoter::reporter transformation and/or in situ hybridization methods.
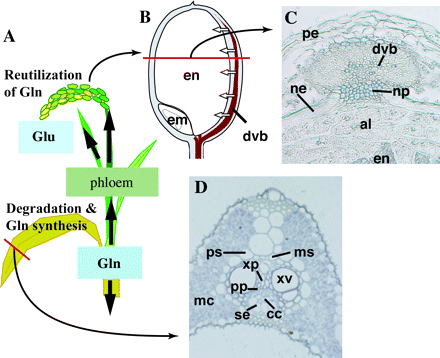
(A) Schematic model for nitrogen remobilization, (B) model of spikelet, (C) cross-section of the dorsal region of grain, and (D) cross-section of large vascular bundle of leaf blade of rice plants. GS1;1 protein was detected in companion cells, phloem-parenchyma cells, and xylem-parenchyma cells in senescing leaf blades (Sakurai et al., 1996), whereas NADH-GOGAT1 protein was located in xylem-parenchyma cells, phloem-parenchyma cells, and mestome sheath cells in developing leaf blade and developing vascular bundle, nucellar projection, nucellar epidermis, and aleurone layer in young grain (Hayakawa et al., 1994), respectively. White arrows in (B) indicate the direction of solute flux from developing vascular bundle to endosperm. Abbreviations: al, aleurone layer; cc, companion cells; dvb, developing vascular bundle; em, embryo; en, endosperm; mc, mesophyll cells; ms, mestome sheath cells; ne, nucellar epidermis; np, nucellar projection; pe, pericarp; ps, parenchyma sheath cell; pp, phloem-parenchyma cell; se, sieve element; xp, xylem-parenchyma cell; xv, xylem vessel element.
An important approach to gaining an understanding of the precise functions of gene products is to use mutants or genetically manipulated plants. In order to obtain more conclusive evidence on the function of NADH-GOGAT1, overexpression of OsNADH-GOGAT1 in the indica cultivar, Kasalath, in an age- and tissue-specific manner was tested (Yamaya et al., 2002), since some of the indica cultivars contained less NADH-GOGAT protein in developing leaf blades than the japonica cultivars (Obara et al., 2000). On the other hand, these indica cultivars contained twice as much GS1 protein in senescing leaves as the japonica cultivars. If our hypothesis was true, the indica cultivars would have a less efficient system of utilizing the remobilized Gln in sink organs. Several T0 transgenic lines overproducing NADH-GOGAT showed an increase in grain weight (80% as a maximum), indicating that this enzyme is indeed a key step for nitrogen reutilization (Yamaya et al., 2002). Although rice mutants deficient in OsNADH-GOGAT1 are not available at the present time, studies with an Arabidopsis knock-out T-DNA insertion mutant in the NADH-GOGAT gene (GLT1) showed that there was a specific defect in growth and glutamate biosynthesis under non-photorespiratory conditions (Lancien et al., 2002). These results support our hypothesis, in which NADH-GOGAT is important in the synthesis of Glu required for the normal growth and development of plants.
In the literature, overexpression of a GS1 gene has not always been linked to increased growth of plants. Transgenic Lotus corniculatus plants overexpressing soybean GS1 driven by a CAMV-35S promoter exhibited both an accelerated growth rate and early leaf senescence (Vincent et al., 1997). Growth improvements have been reported for poplar trees expressing a conifer GS1 (Gallardo et al., 1999) and for tobacco (Fuentes et al., 2001; Oliveira et al., 2002). In contrast, transgenic alfalfa expressing 35S::GS1 (Ortega et al., 2001) or transgenic rice expressing OsGS1;1 under the control of its own promoter (S Hanzawa and T Yamaya, unpublished results) exhibited no difference in growth and phenotype. For this reason, a reverse genetic approach has been used. However, except for rice (Tabuchi et al., 2005), and very recently for maize (Martin et al., 2006), there is no report on the isolation and characterization of any GS1 knock-out mutants, even with Arabidopsis. Failure to isolate the mutants in other plants could be caused by (i) a lethal mutation or (ii) complementation of the GS1 function by another member of the GS1 gene family. Fortunately, in rice, the mutations were not lethal, although meticulous care was required to select these mutants because of severe growth retardation as described below.
Knock-out mutants caused by the insertion of the endogenous retrotransposon Tos17 into the exon of OsGS1;1 were screened and characterized (Tabuchi et al., 2005). Mendelian segregation occurred in each progeny. Homozygously inserted mutants exhibited a severe retardation in growth rate and grain-filling when grown using normal nitrogen fertilizer concentrations, as in Fig. 5. Abnormal mRNA for GS1;1 was transcribed and the GS1 protein and its activity in the leaf blades were barely detected in these mutants. Expression of OsGS1;2 in the leaf blade, leaf sheath, and roots was identical between the mutants and wild-type plants. Reintroduction of OsGS1;1 cDNA under the control of its own promoter into the mutants successfully complemented the slow growth phenotype. These results indicate that GS1;1 is important for normal growth and grain-filling in rice. In addition, GS1;2 and GS1;3 were not able to compensate for the function of GS1;1.
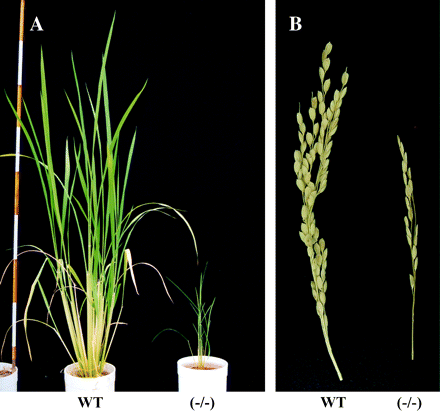
Phenotypic characteristics of OsGS1;1-knockout mutants. (A) Phenotype of wild type (WT) and the knockout mutant line (−/−) caused by the insertion of endogenous retrotransposon Tos17 into OsGS1;1 (Tabuchi et al., 2005) at 64 d after germination. The red and white areas of the scale bar are 10 cm, respectively. (B) Phenotype of panicle on a main stem of wild type (WT) and the knock out mutant (−/−). The mutants showed the retardation of ripening, small panicle size, and less grain filling, as reported previously (Tabuchi et al., 2005).
Future prospects
Nitrogen utilization within rice plants followed by NH4+ uptake and assimilation in the roots is a complex event that depends on many factors, such as the co-ordination of nitrogen with carbon, energy, and other types of metabolism during the growth and development of plants. Of course, the activities of a number of genes will be involved in the nitrogen utilization process, but current knowledge is limited, which makes it difficult to paint the whole picture even in rice, in which genome sequencing was completed and became public in 2005 (International Rice Genome Sequencing Project, 2005). The occurrence of small gene families makes it even more difficult to identify the specific physiological function of each gene product. For example, 29% of the 37 544 predicted genes in rice appear in clustered gene families (International Rice Genome Sequencing Project, 2005). In many cases, the expression of each gene is regulated in a cell type (tissue)-specific, age (development)-dependent, and environmental-adaptive manner, associated with the distinctive and/or redundant function of the gene products. Reverse genetics is a powerful approach, if the mutation is not lethal, to obtain conclusive evidence on the function of the corresponding gene product. Because the metabolic pathway of nitrogen assimilation is well understood from extensive studies of biochemistry and molecular biology, enzymes involved in this pathway can be characterized by reverse genetics, as in the case of GS2 (Wallsgrove et al., 1987) and Fd-GOGAT (Kendall et al., 1986) in barley (Leegood et al., 1995), GS1;1 (Tabuchi et al., 2005) in rice, and GS1-3 and GS1-4 in maize (Martin et al., 2006). However, it is also true that this approach is only of value on an individual gene/protein basis. Since the integration of the functions of many genes is required for the overall process of nitrogen utilization in plants, it is not easy to understand the whole picture from studies on an individual gene. Recent progress in so-called ‘omic’ studies will accelerate the identification of target genes, but there are still large numbers of genes involved after screening by transcriptomic analysis and considerable numbers of unannotated genes are always involved in many cases. More than 35% of the screened genes obtained following transcriptomic analysis using a set of wild-type and OsGS1;1-knockout mutants (M Tabuchi et al., unpublished results) and that of wild-type and RNA-interference-mediated knock-down mutants for a PII-like protein (K Sugiyama et al., unpublished results) were categorized as ‘function unknown’.
It is also very difficult to identify target genes that are involved in regulation, as well as the signal perception and transduction of nitrogen utilization. This is caused by the fact that target factors or proteins involved in signalling and regulation are not well known, unlike enzymes in the metabolic pathway. By consulting well-known systems in other organisms, efforts have been made to identify regulatory factors, such as a PII-like protein. The function of the PII-like protein in plants is unclear in the regulation of nitrogen metabolism, except for its possible involvement in the control of arginine synthesis (Sugiyama et al., 2004; Ferario-Méry et al., 2005). Comprehensive studies including both reverse and forward genetics are therefore required to understand regulatory mechanisms of nitrogen utilization.
An approach using the analysis of quantitative trait loci (QTLs) could be one way to isolate regulatory genes. QTL analysis using DNA markers is an efficient method for estimating the number of genes involved in target traits (Tanksley, 1993). Genetic variability of nitrogen use efficiency has been studied in rice (Obara et al., 2001), maize (Gallais and Hirel, 2004), barley (Mickelson et al., 2003), and wheat (Quarrie et al., 2005). In rice, the effect of QTLs was further confirmed by using chromosome-substituted lines in chromosome 2 (Obara et al., 2004). Target genes controlling uptake, assimilation, and further metabolism of nitrogen, as well as nitrogen use efficiency, could be isolated from the QTL regions on chromosomes in the near future using interspecific crosses. This has already been the case for a gene for fruit size of tomato (Frary et al., 2000), time to heading of rice (Yano et al., 2000), and that for grain productivity of rice (Ashikari et al., 2005).
We thank Miss T Umetsu, Mr T Honda, and Miss A Matsuzaki for technical assistance. We are grateful to Professor Peter Lea, University of Lancaster, UK, for helpful comments and critical reading of the manuscript. This work was supported by a Grant-in-Aid for Scientific Research on Priority Area (16085201) from the Ministry of Education, Culture, Sports, Science and Technology of Japan, and in part by The Project for Rice Genome Research (IP1016) from the Ministry of Agriculture, Forestry and Fisheries of Japan.
References
Author notes
This paper is dedicated to Dr Ann Oaks, Professor Emeritus of McMaster University, Canada, who passed away on 13 January 2006.
These two authors contributed equally to this work.
Comments