-
PDF
- Split View
-
Views
-
Cite
Cite
Ulrich Heber, Marina Azarkovich, Vladimir Shuvalov, Activation of mechanisms of photoprotection by desiccation and by light: poikilohydric photoautotrophs, Journal of Experimental Botany, Volume 58, Issue 11, August 2007, Pages 2745–2759, https://doi.org/10.1093/jxb/erm139
- Share Icon Share
Abstract
Mechanisms of protection against photo-oxidation in selected desiccation-tolerant lichens and mosses have been investigated by measuring loss of light absorption during desiccation and chlorophyll fluorescence as indicators of photoprotection. Apparent absorption (1–T) spectra measured in the reflectance mode revealed stronger absorption of photosynthetic pigments in hydrated than in desiccated organisms, but differences were pronounced only in a cyanolichen, less so in some chlorolichens, and even less in mosses. Since the amplitude of chlorophyll fluorescence is a product of (1–T) light absorption by chlorophyll and quantum yield of fluorescence, and since fluorescence is inversely related to thermal energy dissipation, when chemical fluorescence quenching is negligible, fluorescence measurements were used to measure changes in energy dissipation. Preincubation of the hydrated organisms and desiccation in darkness excluded the contribution of mechanisms of energy dissipation to photoprotection which are dependent on the presence of zeaxanthin or on the light-dependent formation of a quencher of fluorescence within the reaction centre of photosystem II. Fast drying in darkness or in very low light was less effective in decreasing chlorophyll fluorescence than slow drying. Heating the desiccated organisms increased fluorescence by inactivating the mechanism responsible for fluorescence quenching. Glutaraldehyde inhibited fluorescence quenching during desiccation. Prolonged exposure of a desiccated moss or a desiccated lichen to very strong light caused more photo-induced damage after fast drying than after slow drying. The photo-oxidative nature of damage was emphasized by the observation that irreversible loss of fluorescence was larger in air than in a nitrogen atmosphere. It is concluded from these observations that desiccation-induced conformational changes of a chlorophyll protein complex result in the fast radiationless dissipation of absorbed light energy. This mechanism of photoprotection is more effective in preventing photo-oxidative damage than other mechanisms of energy dissipation which require light for activation such as zeaxanthin-dependent energy dissipation or quencher formation within the reaction centre of photosystem II.
Introduction
Chlorophyll, when extracted from photosynthetic organisms in organic solvents, bleaches rapidly in the light in the presence of oxygen. Binding to proteins in photosynthetically active organisms stabilizes it. Photo-oxidative damage is minimized in hydrated plants by metabolic responses that function to afford photoprotection. In 1987, Barbara Demmig observed in Würzburg that the xanthophyll pigment zeaxanthin is linked to controlled thermal energy dissipation which is activated when light flux surpasses the capacity for carbon reduction (Demmig-Adams, 1990). Requirements for the activation of the mechanism permitting energy dissipation are the presence of zeaxanthin and the protonation of a special protein, the gene product of the PsbS gene, in the thylakoid membrane system of the photosynthetic apparatus (Niyogi, 1999; Holt et al., 2004; Li et al., 2004). Protonation of this protein is facilitated by proton-coupled electron transport into the thylakoids (Allen, 2003; Heber, 2003). Controlled protonation initiates thermal energy dissipation within the antenna of PSII. Its extent is regulated so as not to interfere with photosynthesis. Deprotonation in low light or darkness inactivates energy dissipation. This system is eminently suited to respond flexibly to changes in light flux during photosynthesis. An impressive amount of documentation has become available not only on the wide distribution of zeaxanthin-dependent thermal energy dissipation within green plants but also on its regulation (Gilmore and Govindjee, 1999; Bukhov et al., 2001; Ruban et al., 2002; Ma et al., 2003; Holt et al. 2005; Horton et al., 2005; Kopecky et al., 2005; Pascal et al., 2005; Cogdell, 2006; Heber et al., 2006a, and others). Nevertheless, light-induced photo-oxidative damage cannot be completely avoided during photosynthesis in an atmosphere which contains a high concentration of oxygen next to very little carbon dioxide (Asada, 2006). Repair processes involving degradation of dysfunctional protein and synthesis of functional protein are necessary for continued photosynthetic productivity (Aro et al., 1993; Long et al., 1994). These processes cannot proceed in the absence of water.
Whereas most photosynthesizing higher plants do not survive drying, a host of other autotrophic organisms tolerates complete desiccation in strong light. This is particularly true for many mosses, algae, and lichens. Because photosynthesis and repair of photo-oxidative damage is not possible in the absence of water, survival of desiccation-tolerant organisms which retain their chlorophyll in the desiccated state requires de-activation of excited states of photosynthetic pigments before destructive photoreactions can occur. Energy trapping by PSII reaction centres (RCs) which gives rise to the production of a strong oxidant and a strong reductant within the RC takes place within a few picoseconds (Zinth and Kaiser, 1993; Holzwarth et al., 2006). Further reactions of the oxidant, or production of highly oxidative singlet oxygen 1O2* during recombination between oxidant and reductant (Krieger-Liszkay, 2005), initiate oxidative damage. As energy dissipation must be faster than charge separation for photodamage to be avoided in desiccated organisms, it was concluded that thermal de-activation of excited states occurs within the subpicosecond, or femtosecond, time domain when water is absent. This means that the zeaxanthin-dependent mechanism of thermal energy dissipation cannot be the main mechanism of photoprotection of desiccated homoiochlorophyllous photoautotrophs because it is only weakly competitive with energy capture by PSII RCs.
Recently, it was observed that, in some desiccation-tolerant mosses, a mechanism of energy dissipation is activated during slow desiccation which is different from zeaxanthin-dependent energy dissipation. In contrast to the zeaxanthin-dependent mechanism which dissipates energy in the antenna of photosystem II, the site of energy dissipation was within photosystem II RCs. During desiccation, a photoreaction within the RC stabilizes radicals such as reduced pheophytin or oxidized chlorophyll which act as effective quenchers of fluorescence (Heber et al., 2006b). The radicals are stable as long as water is absent. They revert to the uncharged state by losing charge upon hydration.
Another mechanism of effective thermal energy dissipation in desiccated photoautotrophs which is independent of the presence of light is demonstrated here. It is activated during desiccation in darkness and is thought to involve conformational changes of a chlorophyll protein complex which are triggered by desiccation. These changes are considered to form the basis of a dissipating mechanism which is even faster than energy capture by photosynthetic reaction centres, i.e. faster than a few picoseconds (Holzwarth et al., 2006). This mechanism is present in chloro- and cyanolichens and in desiccation-tolerant mosses.
Materials and methods
The poikilohydric sun-tolerant moss Rhytidium rugosum (Ehrh.) Kindb. (family Rhytidiaceae) was obtained either from a sun-exposed habitat (Fig. 3C) or from a more shaded location on calcareous soil near Leinach, about 25 km from Würzburg. The moss Hypnum cupressiforme L. ap. Hedw. (Hypnaceae) was from a sun-exposed wall at the Botanical Garden of the University of Würzburg. The moss Hylocomium splendens (Hedw.) Br. Eur. (Hylocomiaceae) was collected from a forest floor under pine near Leinach. All three mosses are moderately homoiochlorophyllous (Proctor and Tuba, 2002) but lose chlorophyll very slowly owing to photo-oxidation when exposed for several days to full sunlight in the desiccated state. Rhytidium and Hypnum are completely desiccation-tolerant whereas Hylocomium is less stable when desiccation persists for prolonged periods of time. The foliose chlorolichens Parmelia sulcata Ach. and Hypogymnia physodes (L.) Nyl. (Parmeliaceae) were collected from the bark of trees in Würzburg or Leinach, the fruticose lichen Cladonia rangiformis Hoffm. (Cladoniaceae) from a sun-exposed habitat on calcareous soil near Leinach. The cyanolichen Peltigera neckeri Hepp ex Müll. Arg. (Peltigeraceae) was from a shaded site under trees in the Botanical Garden of the University of Würzburg. Dark adaptation of hydrated mosses or lichens (intended to minimize or eliminate zeaxanthin by its conversion to violaxanthin) was achieved by exposing thalli for prolonged times (several hours to a few days) to darkness before drying them either rapidly or slowly in the dark. Rapid drying was done by placing the superficially dried hydrated organisms into a desiccator over silica gel or P2O5. This was followed by rapid evacuation. A thermocouple was used to follow drying by recording the decrease and subsequent increase of temperature of a desiccating sample. Slow drying was done at room temperature in air at a relative humidity below 60% or a water potential below −70 MPa either in darkness or near darkness while fluorescence was recorded in the presence of a very low intensity modulated measuring beam of averaged PPFD=0.02 or 0.04 μmol m−2 s−1. Absence of zeaxanthin-dependent energy dissipation under the conditions of the experiments was checked by making sure that quenching of basal or Fo chlorophyll fluorescence was absent immediately after illumination with strong light pulses (Katona et al., 1992; Kopecky et al., 2005; Heber et al., 2006b). Active zeaxanthin-dependent energy dissipation is indicated by post-illumination Fo quenching.
Spectra (1–T) of lichens or mosses were measured using an Aminco fluorometer with fibre optics connected to the slits of the monochromators which had identical wavelength settings during the recordings.
Modulated chlorophyll fluorescence was measured after excitation at about 650 nm as fluorescence emission beyond 700 nm (using the far-red transmitting filter RG 9 of Schott, Mainz, Germany) by the pulse amplitude modulation fluorometer 101 (PAM) of Walz, Effeltrich, Germany (Schreiber et al., 1986). Fo measured in this mode contains a larger contribution of PSI fluorescence than Fo measured at the maximum of fluorescence emission at 690 nm (Pfuendel, 1998; Franck et al., 2002). Since desiccation-induced fluorescence quenching at 690 nm exceeds quenching at 740 nm (Heber and Shuvalov, 2005), ratios of Fo, hydrated/Fo, desiccated measured in the far-red are smaller than ratios at 690 nm. Short pulses (usually 1 s) of white light (filters: Calflex c and DT-Cyan of Balzers, Liechtenstein) from a halogen lamp (KL 1500 electronic of Schott, Mainz, Germany) were brought to the cuvette by fibre optics to probe for ΔF which originates from PSII RCs (Franck et al., 2002). The PPFD of the light pulses was usually 12000 μmol m−2 s−1 but 2 or 4 μmol m−2 s−1, when high light fluxes had to be avoided. Whenever necessary, the temperature of the samples was monitored by a thermocouple. For measuring fluorescence emission and excitation spectra, the spectrofluorometer SPF 500 of Aminco Instruments (Silver Spring, Maryland, USA.) was used. 430 nm light served for fluorescence excitation and 685 nm for measuring fluorescence emission. The spectra were taken at room temperature where the contribution of PSI to fluorescence is small.
Results
Desiccation of lichens or mosses is accompanied by changes of light absorption
The cyanolichen Peltigera neckeri, almost black in the fully hydrated state, became grey after desiccation. Absorption (1–T) spectra measured in the reflectance mode revealed stronger absorption of chlorophyll a and phycobilisomes in the hydrated than in the desiccated state (Fig. 1, for light absorption by chlorophyll). After rapid drying in vacuo over silica gel or P2O5, absorption was decreased, on average, by 46% at 685 nm (n=3). Slow drying for several hours reduced (1–T) even further, to 61% (n=3). This reduction in apparent absorption was due not to loss of chlorophyll but to a change in the reflective properties of the surface layer of the lichen above the layer containing cyanobacteria.
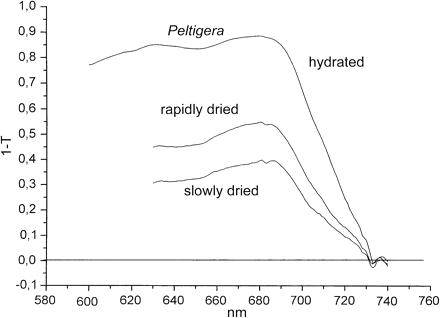
Absorption spectra (1–T) of hydrated and dried Peltigera neckeri as measured in reflection.
In similar experiments with the chlorolichen Parmelia sulcata, (1–T) was reduced at 685 nm by rapid desiccation in vacuo by 20±4.1% (n=6). Slow drying caused a further small reduction of (1–T) by slightly more than 2% (n=11). Light transmittance through the upper cortex of nine different lichen species has been measured by Dietz et al. (2000). Mean cortical transmittances ranged between 45% and 88%. In mosses, light flux to photosynthetic pigments was less impeded than in lichens. Slow drying of Rhytidium rugosum reduced apparent absorption by about 10% (Fig. 2), i.e. less than in cyano- and chlorolichens.
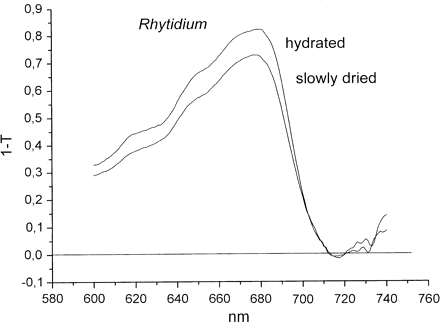
Absorption spectra (1–T) of hydrated and dried Rhytidium rugosum as measured in reflection.
Since the amplitude of chlorophyll fluorescence is a product of (1–T) and the quantum yield of fluorescence, and since loss of fluorescence indicates increased thermal energy dissipation as long as chemical fluorescence quenching can be excluded or neglected (Krause and Weis, 1991; Krause and Jahns, 2004), fluorescence intensity measurements give additional information on protection against excessive illumination. Changes of chlorophyll fluorescence were recorded during hydration and desiccation of photoautotrophs to monitor activation and de-activation mechanisms of thermal energy dissipation.
Activation of chlorophyll fluorescence quenching during desiccation in darkness or near darkness depends on how fast water is lost
In the experiment of Fig. 3, the lichens Parmelia sulcata (A) and Peltigera neckeri (B), and the moss Rhytidium rugosum (C), had been hydrated and kept in darkness for several h. Whereas the chlorolichen Parmelia and Rhytidium are ‘green’, Peltigera has a cyanobacterium as a photobiont which, in contrast to the two other photoautotrophs, does not possess a zeaxanthin cycle (Demmig-Adams et al., 1990). The lichens and the moss were then rapidly dried in vacuo within less than 12 min in darkness (shown visually by a change in colour, or by the reversible temperature decrease owing to transpirational cooling, as recorded by a thermocouple during evacuation). Drying was continued for several hours in vacuo to be certain of complete desiccation. Although fast drying decreased fluorescence, this decrease was less pronounced than the decrease produced during slow drying would have been (as recorded in control experiments, not shown). Strong short light pulses increased fluorescence transiently more in the desiccated organisms than they would have done after drying had been slow (compare with fluorescence at the end of the experiments of Fig. 3). The fluorescence spikes indicate charge separation in PSII RCs even after drying.
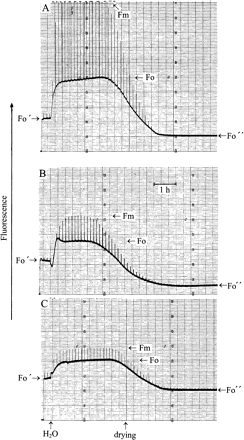
Modulated chlorophyll fluorescence of the chlorolichen Parmelia sulcata (A), the cyanolichen Peltigera neckeri (B), and the moss Rhytidium rugosum (C). Very strong light pulses (1 s) were given every 500 s.. After rapid desiccation of the organisms, basal fluorescence was at a low Fo′ level. Light pulses caused small fluorescence responses. Hydration increased fluorescence to the levels Fo and Fm. Slow desiccation suppressed both levels towards Fo″. For explanation, see text.
Rehydration increased both Fo and Fm fluorescence. ΔF/Fm also increased. This indicates increased charge separation in active PSII RCs (Genty et al., 1989). The ratio ΔF/Fm was 0.61 at maximum in Parmelia, 0.32 in Peltigera, and 0.17 in the moss. The differences may be caused by the different degrees of photoinhibition of PSII RCs the lichens and the moss had suffered in the field before they were collected for experimentation. Prolonged hydration increased ΔF/Fm but the maximum ratios common in higher plants (about 0.8; Björkman and Demmig-Adams, 1987) were only observed in rare cases (data not shown). Slow dehydration over a time span of about 120 min or more in near-darkness (Fig. 3; or full darkness, not shown) decreased both Fm and Fo fluorescence strongly. Fo levels attained after slow drying were 45% of the level observed after fast drying in Parmelia, 24% in Peltigera, and 73% in the moss. Also, pulse-induced fluorescence spikes were decreased after slow drying compared with the responses produced after fast drying. When the experiments of Fig. 3 were done in a reversed order, with slow drying preceding fast drying, the results were essentially similar to those shown, indicating that the sequence of fast or slow drying did not influence the results.
Two main points of the experiments of Fig. 3 need to be emphasized. (i) Loss of fluorescence during desiccation is larger than loss of (1–T). For instance, for the cyanolichen Peltigera the ratio (1–T)hydrated/(1–T)slowly dried is 2.25 at 680 nm in Fig. 1, whereas the ratio Fo, hydrated/Fo″, slowly dried is 6.5 in Fig. 3. For the moss Rhytidium, the ratio (1–T)hydrated/(1–T)slowly dried is 1.1 in Fig. 2 and the ratio Fo, hydrated/Fo″, slowly dried is 2 in Fig. 3. This compares fluorescence emission beyond 700 nm with absorption at 680 nm. However, loss of fluorescence during desiccation is usually larger at 685 nm than around 700 nm (Kopecky et al., 2005; Heber and Shuvalov, 2005). Therefore, the differences between desiccation-induced loss of absorption and loss of Fo fluorescence are, in fact, larger than those calculated above for emission beyond 700 nm. (ii) Less fluorescence is lost during fast drying of different poikilohydric photoautotrophs than during slow drying. This observation makes it possible to understand how desiccation activates energy dissipation. It shows that the time factor is important for the extent of photoprotection.
Slow drying simultaneously quenched Fo fluorescence and decreased or even eliminated RC activity as shown by the loss of pulse-induced fluorescence responses. Fo/Fo″ ratios were different in different species, but not fixed even within one species. Their variability suggests that the extent of energy dissipation during desiccation is subject to regulation.
Part of these desiccation experiments were performed in near darkness, others in full darkness. In the experiments of Fig. 3, a very low intensity measuring beam (PPFD <0.05 μmol m−2 s−1) was needed to record fluorescence responses during slow drying. The 1 s light pulses intended to probe for charge separation were insufficient to activate appreciable fluorescence quenching. They were spaced 500 s apart. The largest difference in fluorescence between fast and slow drying was observed in the cyanolichen Peltigera (Fig. 3B). The ratio Fo′/Fo″ was 3.5. By contrast, (1–T)685 nm, fast/(1–T)685 nm, slow was 1.4. The difference between these values shows that increased fluorescence quenching during slow drying can be attributed only in part to loss of absorption. The same holds true for chlorolichens such as Parmelia or the moss Rhytidium.
Fast drying was done in vacuo to accelerate the loss of water. Anaerobiosis led occasionally to some reduction of QA in PSII RCs before drying was complete. Oxidation of this reduced QA is seen as a transient loss of fluorescence owing to reoxidation of QA− in the experiment of Fig. 3B immediately after hydration. It was concluded that reduction of QA is not responsible for the differences in fluorescence between fast and slow drying because essentially similar results were obtained when fast and slow drying was done in complete darkness, or when a stream of warm air was used to accelerate drying rather than evacuation.
Fluorescence was quenched similarly by desiccation, whether light had been absorbed by chlorophyll a or by chlorophyll b
Bilger et al. (1989) had observed that light absorbed by chlorophyll b caused less fluorescence emission than light absorbed by chlorophyll a after a chlorolichen, Ramalina maciformis, had been desiccated. They concluded from 77 K fluorescence excitation spectra that desiccation had interrupted energy transfer from the antenna of PSII (which contains chlorophyll b) to PSII RCs. Using excitation spectra taken at room temperature, their original observations were confirmed (Kopecky et al., 2005) using other chlorolichens which had been collected in the field. However, after previous dark incubation of hydrated chlorolichens and subsequent desiccation in darkness had eliminated the participation of mechanisms of energy dissipation which require light for activation, there was a failure to obtain evidence for preferential quenching of light absorbed by chlorophyll b. Representative spectral information is given in Fig. 4A for the chlorolichen Parmelia sulcata. The upper spectrum shows 685 nm fluorescence for a rapidly dried thallus of Parmelia sulcata as a function of the wavelength of exciting light, the lower spectrum is the same for the same thallus after slow drying. Fluorescence emission was quenched at all wavelengths more by slow than by fast drying (see also Fig. 3). In particular, quenching was similar at 480 nm and at 440 nm. 480 nm light is absorbed by chlorophyll b, 440 nm light by chlorophyll a. Very similar differences in quenching between rapid and slow drying were observed in the excitation spectra of the moss Rhytidium rugosum (not shown). No preferential quenching of 480 nm excitation was observed in the moss.
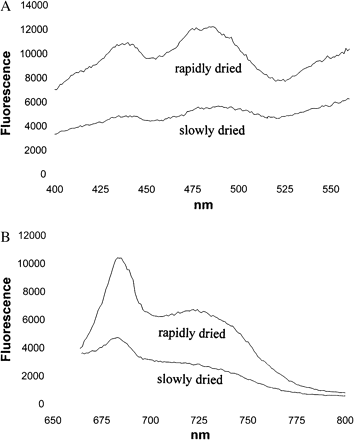
Fluorescence excitation spectra (A) of a thallus of Parmelia sulcata and fluorescence emission spectra (B) of Rhytidium rugosum after rapid drying (upper spectra) or after slow drying (lower spectra). Before drying in the dark, the hydrated organisms had been predarkened for 1 d.
Figure 4B shows the emission spectra of rapidly (upper spectrum) and slowly dried Rhytidium rugosum (lower spectrum). Fluorescence was excited at 430 nm. Emission had peaks at 685 nm and about 730 nm. Slow drying increased quenching similarly at 685 nm and 730 nm when compared to fast drying. In field-dried mosses, loss of fluorescence was often stronger at 685 nm than at 730 nm (Kopecky et al., 2005; Heber and Shuvalov, 2005).
Heating reverses the fluorescence decrease after drying has been slow
Recently, it was observed that heating desiccated Parmelia resulted not only in a reversal of the desiccation-induced fluorescence quenching but also reactivated PSII RCs as shown by pulse-induced fluorescence increases. These effects were reversible as long as heating had not been damaging (Heber and Shuvalov, 2005). Additional weight was given to these findings when it was noticed that the extent of desiccation-induced fluorescence decrease depended on how fast water was lost during drying (Figs 3, 4) In Fig. 5, the responses of fluorescence to heating, using desiccated autotrophs which had been dried either rapidly or slowly, are compared. In rapidly dried Parmelia short strong light pulses caused barely noticeable transient quenching of fluorescence below 20 °C. Increasing the temperature reversed the direction of the transient signals (Fig. 5A). Another shift in direction occurred around and above 60 °C. Fo fluorescence started to increase slightly at temperatures above 30 °C. A reversal of pulse-induced fluorescence changes beyond 60 °C suggested heat-induced damage.
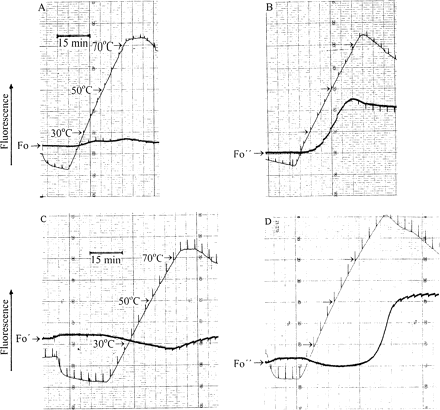
Simultaneous recordings of temperature and fluorescence of desiccated Parmelia sulcata (A, B) and desiccated Rhytidium rugosum (C, D). The temperature was first decreased and then increased. Strong 1 s light pulses were given every 200 s to monitor variable fluorescence. Pulse-induced fluorescence changed direction as the temperature was increased. (A, C) The lichen and the moss had been rapidly dried in vacuo. (B, D) The lichen and the moss were slowly desiccated as shown in Fig. 3. Simultaneous responses of temperature and fluorescence to brief light pulses show that the fluorescence trace is slightly displaced to the left of the temperature trace.
In the experiment of Fig. 5B, a slowly dried Parmelia sample was heated. In this case, Fo fluorescence also started to increase at 30 °C, but the increase was much more pronounced than in the experiment of Fig. 5A. Pulse-induced fluorescence responses were initially very small and negative, then larger and positive until at temperatures close to 70 °C they changed direction again.
In the experiment of Fig. 5C, the moss Rhytidium had been rapidly dried. Heating failed to increase fluorescence. Rather, fluorescence decreased until it started to increase at around 70 °C.
In Fig. 5D, Rhytidium desiccated under field conditions and collected in the sun was subjected to a heating experiment. In contrast to the experiment with the rapidly dried moss, heating induced a conspicuous fluorescence rise similar to that observed with the lichen Parmelia in Fig. 5B.
Heating experiments performed with slowly dried thalli of the chlorolichen Hypogymnia physodes and of the cyanolichen Peltigera neckeri and with the sun-dried mosses Hylocomium splendens and Hypnum cuppressiforme yielded temperature-induced fluorescence increases similar to those shown in Fig. 5B and D (data not shown).
Because of the inverse relationship between fluorescence and energy dissipation, the strong increase of fluorescence observed during heating of slowly or sun-dried autotrophs (Fig. 5B, D) suggests thermal inactivation of a mechanism of energy dissipation which had been activated during slow drying in the laboratory or drying under field conditions. Fast drying had not been effective for full activation (Figs 3, 5A, C).
Glutaraldehyde inhibits the desiccation-induced fluorescence decrease
Incubation of desiccated Parmelia for 90 min in 0.5% aqueous glutaraldehyde solution decreased light-dependent charge separation in PSII RCs, but did not fully eliminate positive pulse-induced fluorescence spikes. During slow drying, light-induced charge separation was lost and replaced by small reversible quenching responses. Importantly, desiccation-induced fluorescence Fo quenching was absent (data not shown). Glutaraldehyde is known as a fixative in electron microscopy. It possesses two reactive aldehyde groups and is capable, by crosslinking, of reacting with proteins.
After drying of glutaraldehyde-treated Rhytidium, NPQ was 0.25 compared to NPQ=1.95 in an untreated desiccated control experiment.
Different mechanisms contribute to the desiccation-induced fluorescence loss under field conditions
In the experiment of Fig. 6 with sun-exposed desiccated Parmelia which had been collected in the field, fluorescence was excited by a measuring beam of extremely low intensity, i.e. the sample was kept close to darkness (PPFD=0.03 μmol m−2 s−1). Low-intensity light pulses (1 s; PPFD=1.8 μmol m−2 s−1) were given every 500 s to monitor charge separation in PSII RCs. In the desiccated state, they had no effect on fluorescence. Hydration increased fluorescence from Fo′ towards Fo. The light pulses increased fluorescence transiently indicating charge separation and some QA reduction. At steady state, the ratio Fo/Fo′ was 7.3. While the lichen was hydrated in near darkness, zeaxanthin-dependent energy dissipation which had possibly been active in the desiccated state was slowly inactivated, presumably by dissociation of the complex between protonated PsbS-protein and zeaxanthin (Kopecky et al., 2005; Heber et al., 2006b). Post-illumination Fo quenching was present only initially (not shown). During subsequent slow drying, fluorescence decreased. In the dry state, the ratio Fo′/Fo″ was now 4.7, i.e. lower than before hydration. Apparently, the state of energy dissipation had decreased as a consequence of hydration in near darkness.
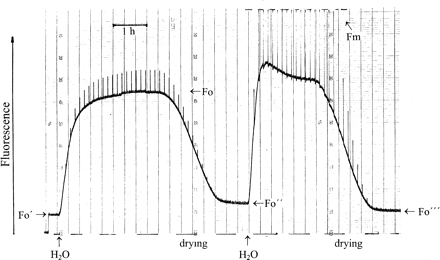
Modulated chlorophyll fluorescence of Parmelia sulcata. Fo′ shows fluorescence of the sun-dried lichen, Fo″ after hydration and slow drying in semidarkness, Fo″′ after a second hydration and slow drying in continuous light (PPFD=140 μmol m−2 s−1). 1 s light pulses given every 500 s had PPFD=2 μmol m−2 s−1 (first hydration) and 12 000 μmol m−2 s−1 (second hydration). PPFD in ‘Semi-darkness’ was 0.04 μmol m−2 s−1. For explanation, see text.
A second hydration cycle in the presence of background illumination increased fluorescence. The background light had PPFD=140 μmol m−2 s−1. Very strong light pulses (PPFD=12 000 μmol m−2 s−1) were also given every 500 s. Illumination served to activate mechanisms of energy dissipation which require light for activation. In recent work with the shade-tolerant moss Rhytidiadelphus squarrosus, it has been shown that acquisition of phototolerance in this moss requires light (Heber et al., 2006b). A photoreaction is responsible for the formation of a quencher in PSII RCs. Light is also needed to activate zeaxanthin-dependent energy dissipation. In fact, transient post-illumination quenching after every strong light pulse in Fig. 6 indicated activation of zeaxanthin-dependent energy dissipation (Katona et al., 1992; Heber et al., 2006b). Slow drying decreased fluorescence again. During drying, post-illumination quenching was stabilized This can be seen by a shift of the trace towards the left after every light pulse. In the desiccated state, fluorescence was lower than before the second hydration cycle. Fo/Fo″′ was 6.2, i.e. almost as high as it had been after the desiccated lichen had been collected in bright sunshine. The ratio Fo″/Fo″′ was 0.75. This means that, after desiccation in the light, 75% of total energy dissipation is still attributable to a mechanism which does not require light for activation. Only about 25% must be assigned to mechanisms which need light for activation.
In an experiment with the moss Rhytidium rugosum which was similar to the lichen experiment of Fig. 6, Fo″/Fo″′ was 0.70. About 30% of total energy dissipation was caused by light-requiring dissipation mechanisms. As this includes energy dissipation within PSII RCs (Heber et al., 2006b), zeaxanthin-dependent energy dissipation is unlikely to be a major contributor to photoprotection in desiccated photoautotrophs.
Irrespective of the activation of mechanisms of energy dissipation during desiccation, photo-reactions can still be observed in the absence of water. They have a very low quantum efficiency. In the experiment of Fig. 7A, desiccated Parmelia sulcata was strongly illuminated with PPFD=12 000 μmol m−2 s−1. At this high intensity, accumulation of photoproducts is proportional to photon flux until saturation is approached (data not shown). Two photoreactions are seen. An initial fast lowering of fluorescence immediately after the onset of illumination shows formation of a quencher. This is superposed by a slower fluorescence increase which indicates charge separation in functional PSII RCs and subsequent QA reduction. Fluorescence declined slowly during continued illumination. It reflects the slow accumulation of the quencher which had been seen initially only as a brief and fast quenching response. Turning the light off caused relaxation of the slow fluorescence increase which had indicated photoaccumulation of QA−. Nevertheless, light-induced quenching persisted after darkening, showing that the photo-accumulation of the quencher which had been observed only as a transient response was not, or not completely, reversible. When the experiment was repeated a second and a third time, light-dependent QA reduction was reversible, but less and less quencher accumulated in repeated illumination cycles.
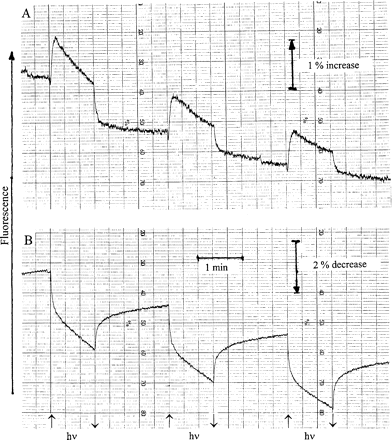
Responses of modulated chlorophyll fluorescence of Parmelia sulcata (A) and Rhytidium rugosum (B) to 1 min cycles of strong illumination (PPFD=12 000 μmol m−2 s−1).
In the experiment of Fig. 7B, the moss Rhytidium was subjected to the same sequence of illuminations. In this case, no photo-accumulation of QA− could be observed. Apparently, PSII RCs no longer functioned in energy conservation. Rather, a quencher was formed in the light. Such formation had already been described for the moss Rhytidiadelphus squarrosus (Heber et al., 2006b). Quencher formation was partially reversible on darkening: fluorescence did not fully recover. Repeated illuminations caused more quenching of fluorescence. Most of the light-induced quenching relaxed on darkening. This contrasts with the lichen data of Fig. 7A where irreversible fluorescence quenching slowly approached saturation. In Rhytidiadelphus squarrosus, where the photoaccumulation of a quencher has been described in some detail (Heber et al., 2006b), quencher formation became irreversible as desiccation progressed. Here, in Rhytidium rugosum, reversible quencher formation has been observed, but the moss had been desiccated from the outset. In all these cases, formation of a quencher in the light contributes to thermal energy dissipation in the absence of water. This was also true for the cyanolichen Peltigera neckeri (data not shown).
Slow drying of predarkened lichens and mosses is more photoprotective than fast drying
Figure 8A shows chlorophyll fluorescence of a sample of Parmelia sulcata which had been predarkened for several hours in the hydrated state before it was rapidly dried in the dark. Fast drying decreased fluorescence to the level Fo′ as shown in Fig. 3A. Strong illumination increased fluorescence only initially. Subsequently, the fluorescence trace reversed direction. After 20 min illumination with PPFD=12 000 μmol m−2 s−1, about 26% of initial fluorescence Fo′ were lost. Absorption of strong light raised the temperature of illuminated samples. This was monitored by thermocouples. After 20 min of strong illumination, temperature had increased above room temperature in different experiments by less than 10 °C. This is insufficient to damage desiccated lichens and mosses (Fig. 5). Darkening did not change fluorescence much in the experiment of Fig. 8A. Hydration increased fluorescence after a lag phase. Strong light pulses given every 200 s increased fluorescence transiently. ΔF/Fm were at maximum about 0.14 compared to 0.66 before drying the hydrated sample.
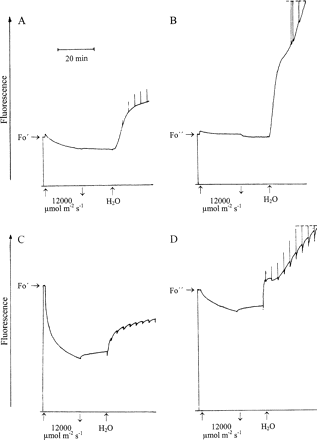
Responses of modulated chlorophyll fluorescence of Parmelia sulcata (A, B) and Rhytidium rugosum (C, D) to 20 min strong illumination and to hydration in semi-darkness. 1 s light pulses (PPFD=12 000 μmol m−2 s−1) produced transient effects on fluorescence as shown in the trace. (A, C) the organisms had been rapidly dried; (B, D) the organisms had been slowly dried. For effects of rapid and slow drying, see also Figs 1–5.
In Fig. 8B, a comparable experiment is shown. However, in this case, the Parmelia sample was dried slowly in darkness. Fo was suppressed to the level Fo″ (Fig. 3A). As in Fig. 8A, illumination increased fluorescence initially. However, the subsequent decrease was much less pronounced than in Fig. 8A. Darkening reversed most of the fluorescence increase seen on illumination. Hydration increased fluorescence much more than in Fig. 8A. The ratio of Fo to Fo″ was 4 at the end of the experiment (end of recording not shown, but compare with values close to 2 in Fig. 3A after rapid drying and almost 5 after slow drying). Strong light pulses also increased fluorescence more than in Fig. 8A. Maximum ΔF/Fm values approached 0.53 (not shown). The difference to the initial value of 0.66 which was observed before light stress is small compared to the value of 0.14 in the experiment of Fig. 8A. Obviously, PSII RCs of desiccated Parmelia suffered less damage after drying had been slow than after it had been rapid.
In Fig. 8C and D experiments very similar to the lichen experiments of Fig. 8A and B are shown for the moss Rhytidium rugosum After rapid drying, Rhytidium was illuminated for 20 min with PPFD=12 000 μmol m−2 s−1. Fluorescence decreased considerably. After 20 min illumination, it was 43% of its initial level. Darkening reversed only a small part of the light-induced quenching. Hydration increased fluorescence, but failed to raise it to and beyond the level observed before prolonged illumination. Short strong light pulses caused some transient fluorescence quenching. Loss of positive values of ΔF/Fm during prolonged illumination is attributed to photodamage suffered by PSII RCs during light stress.
After Rhytidium had been slowly dried, less fluorescence was lost during the 20 min period of light stress compared to fluorescence loss of the rapidly dried sample (Fig. 8B versus 8A). Again, darkening reversed only part of the light-induced fluorescence loss but hydration increased fluorescence beyond the level seen before prolonged illumination. Short light pulses increased fluorescence transiently indicating QA reduction. ΔF/Fm increased slowly and reached values beyond 0.15.
A comparison of the fluorescence parameters of Fig. 8 shows that slow drying in darkness of the lichen Parmelia and of the moss Rhytidium is more photoprotective than fast drying.
Damage suffered under strong irradiation by rapidly dried desiccation-tolerant lichens and mosses is predominantly, but not exclusively, caused by the activation of oxygen
In the experiments of Fig. 9, the gas atmosphere was either air or nitrogen while desiccated Parmelia (A) and Rhytidium (B) were exposed for 20 min to very strong white light. Both organisms had been rapidly dried. Loss of fluorescence during irradiation was much reduced when the gas atmosphere was nitrogen compared with air. Also, darkening reversed a larger percentage of quenching under nitrogen than in air. When nitrogen was replaced by air after darkening, fluorescence increased again. Apparently, an anionic quencher which had accumulated in the light lost its quenching properties after reacting with oxygen. Essentially, all of the quenching observed during illumination in nitrogen was reversible after darkening. This is in striking contrast to the irreversibility of most of the quenching produced by strong irradiation in air. Nevertheless, hydration failed to re-establish the pulse-induced charge separation in PSII RCs which finds expression in large positive pulse-induced fluorescence spikes after light stress as seen in Fig. 8B and D (hydration data not shown in Fig. 9). This shows that, as far as photodamage to PSII RCs is concerned, strong illumination in nitrogen was not distinctly less damaging to RC activity than illumination in air.
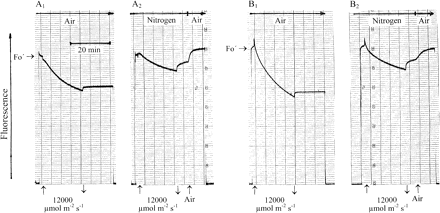
Effects of the gas atmosphere on responses of modulated chlorophyll fluorescence of rapidly dried Parmelia sulcata (A1, A2) and Rhytidium rugosum (B1, B2) to prolonged strong illumination.
Discussion
Mechanisms of radiationless energy dissipation in desiccation-tolerant lichens and mosses
As shown in the Results, desiccation of poikilohydric photoautotrophs often increases reflectance of light. Simultaneously, fluorescence is quenched. Increased reflectance lowers light pressure on the photosynthetic apparatus. Thereby it contributes to photoprotection. However, whereas loss of absorption was larger in a cyanolichen than in the chlorolichens and mosses examined, it was always small compared with loss of fluorescence, which indicates activation of radiationless dissipation of absorbed light energy because fluorescence is inversely related to energy dissipation when energy conservation is absent or negligible as it is in the absence of water. Therefore, the focus has been on mechanisms of desiccation-induced energy dissipation. In particular, it is shown that one of these mechanisms is based on structural changes of a chlorophyll protein complex. Activation of this mechanism does not require light. This makes it possible to distinguish it from other mechanisms of energy dissipation. Light is needed for the formation and stabilization of a quencher of fluorescence in PSII RCs during desiccation (Heber et al., 2006b). Zeaxanthin-dependent energy dissipation which is thought to be localized in the antenna of PSII is known to be regulated by a light-dependent protonation reaction (Li et al., 2004; Kopecky et al., 2005). Prolonged predarkening in the hydrated state of mosses or lichens which possess a zeaxanthin cycle de-activates previously active zeaxanthin-dependent energy dissipation. Drying in the dark does not permit activation. Therefore desiccation-induced energy dissipation of poikilohydric autotrophs which had been kept in the hydrated state in the dark and were then dried in darkness (or near darkness) is unrelated to light-dependent quencher formation and to zeaxanthin-dependent energy dissipation.
The extent of desiccation-induced fluorescence decrease observed in lichens and sun-tolerant mosses depends on how fast water is lost during drying. Slow desiccation produced more activation of a mechanism of energy dissipation than the rapid loss of water. Apparently, activation is time-dependent (Figs 1–3). This reveals the involvement of conformational changes.
Recently, Pascal et al. (2005), analysing the structure of the main light-harvesting complex of PSII of hydrated higher plants with the aim of understanding the mechanism of zeaxanthin-dependent energy dissipation concluded from the correlation between fluorescence quenching and conformational changes of the protein structure of LHCII upon crystallization that changes in structure of chlorophyll proteins can give rise to altered pigment–pigment interactions which, in turn, can lead to the formation of quenching centres. Cogdell (2006) considered this the molecular basis of energy dissipation. Nevertheless, at the present time the relationship between the findings of Pascal et al. (2005) and zeaxanthin-dependent fluorescence quenching is not clear. Holt et al. (2005) have proposed a completely different mechanism of energy dissipation. It is based on charge transfer from zeaxanthin to chlorophyll within a complex between protonated PsbS protein, zeaxanthin, and chlorophyll. Times of zeaxanthin oxidation were in the picosecond range. This is similar to charge transfer between P680 and pheophytin in PSII RCs during energy conservation.
For the understanding of the molecular basis of desiccation-induced energy dissipation in lichens and mosses, the model of Pascal et al. (2005) and of Cogdell (2006) for LCHII is useful. Chlorophylls responsible for light absorption are embedded in protein structures. Loss of water during desiccation is thought to alter the position of pigments to one another by altering the conformation of a specific pigment–protein complex. This is thought to lead to the formation of quenching centres.
The mechanism responsible for desiccation-induced fluorescence decrease is fully reversible. By re-establishing protein conformations that had been altered by desiccation, hydration increases fluorescence. Activation of the mechanism responsible for fluorescence quenching during desiccation is inhibited by glutaraldehyde which is known to react with proteins (Coughlan and Schreiber, 1984). Glutaraldehyde is capable, by crosslinking reactions, to ‘fix’ proteins. Inactivation of the mechanism by heating increases fluorescence of desiccated photoautrotrophs first reversibly, then irreversibly (Fig. 5B, D). Heating is known to unfold proteins. However, LCHII is not a likely candidate for fluorescence quenching in poikilohydric photoautrotrophs because drying of leaves which contain LCHII does not result in the quenching of Fo fluorescence (Kopecky et al., 2005).
The extent of desiccation-induced quenching of Fo fluorescence is different in different species of lichens and mosses. It varies even within species. In sun-exposed Cladonia rangiformis, the ratio of Fo in the hydrated state to Fo′ after desiccation approached or exceeded 20 in summer. It was much lower in winter. In different experiments with Parmelia sulcata, Fo, hydrated/Fo″, desiccated varied between 2 and 10. These ratios are actually mimimum values as they were measured beyond 700 nm where the contribution of PSI to Fo is not negligible (Pfuendel, 1998; Franck et al., 2002). When measured at 690 nm, where desiccation-induced quenching is usually stronger than beyond 700 nm (Heber and Shuvalov, 2005), the ratios are larger. From the variability of the ratios it appears that dissipation centres formed as a result of conformational changes during water loss differ in dissipation strength. Differences in dissipation strength show up as differences in fluorescence life times (see also Pascal et al., 2005). Increased quenching is the result of the shortening of fluorescence life times. In very low light, Fo, hydrated is in equilibrium with energy capture by open PSII RCs. Energy capture occurs within 1.5 ps (Holzwarth et al., 2006). When Fo″, desiccated is lower than Fo, hydrated, energy dissipation is faster than energy conservation in functional reaction centres, i.e. faster than 1.5 ps. When it is much lower, it is in the subpicosecond, in the femtosecond, range. In this situation, sunlight (about 1800 μmol m−2 s−1) is insufficient to cause appreciable charge separation in fully functional RCs. In accordance with this, photon fluxes as high as 12 000 μmol m−2 s−1 are needed to produce appreciable QA reduction in Parmelia (Fig. 7A). In the moss Rhytidium, where Fo, hydrated/Fo″, desiccated ratios are usually lower than in Parmelia, quencher formation provides additional photoprotection to that given by desiccation-induced Fo suppression (Fig. 7B; Heber et al., 2006b).
It is concluded that autotrophs which decrease Fo fluorescence on desiccation contain a special chlorophyll protein complex which changes its conformation on desiccation. This causes the reversible formation of quenching centres and leads to the effective dissipation of absorbed light energy. In combination with decreased light absorption, it increases phototolerance to the point where oxidation-sensitive pigments are stabilized in an atmosphere which contains 21% oxygen.
Localization of quenching which is based on conformational changes of a chlorophyll protein complex
Desiccation-induced quenching is remarkably similar in a moss, a chlorolichen, and a cyanolichen (Fig. 3). This suggests a common basis of quenching in the different taxa. Does it permit some preliminary conclusions regarding the localization of quenching centres? In the cyanolichen (Fig. 3B) the antenna of PSII is completely different from that of mosses and chlorolichens. It is composed of phycobilins rather than chlorophyll b-containing light-harvesting proteins. The reaction centre of PSII itself is unlikely to be the site of desiccation-induced Fo quenching because PSII RCs remain functional in chlorolichens (Fig. 7A). In mosses, a photoreaction results in the stabilization of a radical within the RC during desiccation which acts as a quencher (Fig. 7B; Heber et al., 2006b). Also, it is difficult to see how the D1/D2 proteins of the RC could have the structural flexibility required for the light-independent formation of quenching centres of variable quenching strength. In this situation, we propose tentatively a localization within the PSII core complex for the chlorophyll protein which is responsible for quenching.
Co-operation of different mechanism of energy dissipation

Similar results were obtained with the sun-tolerant moss Rhytidium rugosum. In a representative experiment, Fo/Fo′ was 4.6 before hydration in near darkness. Fo/Fo″ decreased to 2.85 after hydration. Hydration in the light increased the ratio to 4.05. The ratio 2.85/4.05 is 0.7. Again the mechanism of energy dissipation whose activation did not require the presence of light during desiccation was mainly responsible for photoprotection. In remarkable contrast to this, the shade-tolerant moss Rhytidiadelphus squarrosus, of the same family Rhytidiaceae, relied mainly on light-activated mechanisms of photoprotection (Heber et al., 2006b).
Sites of sensitivity to excessive light and damage suffered in the desiccated state

Indeed, the experiments of Fig. 8 show strong irreversible quenching of chlorophyll fluorescence during prolonged strong illumination in air particularly after fast desiccation had not permitted full activation of energy dissipation. Irreversibility of quenching is an indicator of damage. Loss of RC function was indicated by considerable loss of pulse-induced fluorescence quenching after hydration. After slow desiccation, damage was reduced. When the illumination experiments were repeated not in air but in nitrogen, loss of fluorescence in the light was much reduced (Fig. 9). Darkening under nitrogen reversed a considerable part of the quenching indicating that a quencher lost its quenching properties in a recombination reaction. More reversibility of quenching was observed when nitrogen was replaced by air. Apparently, a reaction with oxygen caused further loss of quencher. Thus, illumination in nitrogen reduced or eliminated the photo-oxidation which is characterized by the irreversible loss of fluorescence. Nevertheless, hydration failed to recover most of pulse-induced fluorescence responses (not shown): strong illumination of desiccated moss or lichen under nitrogen had failed to protect PSII RCs from loss of function. Apparently, as a strong oxidant, P680+ can cause damage either by unspecific oxidation reactions within the photosynthetic apparatus or, after recombination with reduced chlorophyll (Heber et al., 2006a) or Pheo− (Krieger-Liszkay 2005), via formation of singlet oxygen.
Abbreviations
- Chl
chlorophyll
- Fo
basal modulated chlorophyll fluorescence of hydrated photoautotrophs in equilibrium with energy capture by open reactions centres of PSII, indicating oxidation of the primary quinone acceptor QA of PSII
- Fo′,Fo″,Fo″′
Fo levels suppressed by desiccation
- Fm
maximum modulated chlorophyll fluorescence elicited by saturating light pulses indicating reduction of the primary quinone acceptor QA of PSII
- ΔF/Fm=(Fm–Fo)/Fm
quantum efficiency of charge separation in PSII
- NPQ = (Fm/Fm′−1)
non-photochemical fluorescence quenching
- PPFD
photosynthetically active photon flux density
- PSII, PSI
photosystems II or I
- T
transmission I/Io
This research was supported by Deutsche Forschungsgemeinschaft. It is the late result of co-operative field work with Professor OL Lange, University of Würzburg, who patiently encouraged the senior author to pay attention not only to higher but also to lower plants. We are grateful to Professor Wolfgang Bilger, University of Kiel, for advice and information and to Dr Rainer Wolf, Würzburg, for preparing images of Peltigera neckeri by raster electron microscopy.
References
Author notes
Dedicated to Professor Otto-Ludwig Lange on the occasion of his 80th birthday.
Comments