-
PDF
- Split View
-
Views
-
Cite
Cite
Yukinori Yabuta, Takahiro Mieda, Madhusudhan Rapolu, Ayana Nakamura, Takashi Motoki, Takanori Maruta, Kazuya Yoshimura, Takahiro Ishikawa, Shigeru Shigeoka, Light regulation of ascorbate biosynthesis is dependent on the photosynthetic electron transport chain but independent of sugars in Arabidopsis, Journal of Experimental Botany, Volume 58, Issue 10, July 2007, Pages 2661–2671, https://doi.org/10.1093/jxb/erm124
- Share Icon Share
Abstract
It has been known that leaves exposed to high light contain more L-ascorbic acid (AsA) than those in the shade. However, the mechanism of the light regulation of the AsA pool size in plants is largely unknown. In this work, the relationship between gene expression levels related to AsA biosynthesis and photosynthesis have been studied. When 2-week-old Arabidopsis plants grown under a 16 h daily photoperiod were moved into the dark, the AsA level in the leaves was decreased by 91% in 72 h, whereas it increased by 171% in the leaves of plants exposed to continuous light during the same period. Among the several enzymes of the AsA biosynthesis pathway, the transcript levels of GDP-D-mannose pyrophosphorylase, L-galactose 1-P phosphatase, L-galactono-1,4-lactone dehydrogenase, and the VTC2 gene were down-regulated in the dark. Treatment with inhibitors of photosynthesis, 3-(3,4-dichlorophenyl)-1,1-dimethylurea and atrazine, arrested a rise in the AsA pool size accompanying the decrease in the transcript levels of the genes of the above enzyme in the leaves. When the plants were transferred to a medium containing 0.5% (w/v) sucrose, the photosynthesis activities and the leaf AsA levels were lowered even under exposure to light compared with those in plants on the medium without sucrose. In contrast, the AsA level in leaves of the sugar-insensitive Arabidopsis mutant abi4/sun6 was unaffected by external sucrose. No significant difference in the expression profiles for AsA biosynthesis enzymes was observed between the wild-type and mutant plants by sucrose feeding. The results suggest that photosynthetic electron transport of chloroplasts is closely related to AsA pool size regulation in leaves.
Introduction
L-Ascorbic acid (vitamin C, AsA) is an important antioxidant and acts as a cofactor for several enzymes. In plants and eukaryotic algae, it may represent one of the major soluble carbohydrates, and functions in essential physiological processes such as photosynthesis, transmembrane electron transport, cell division and growth, and stress resistance (Smirnoff and Wheeler, 2000). In spite of its multiple roles, the biosynthesis pathway of AsA in plants had not been established until recently. It has been known that L-galactono-1,4-lactone (L-GalL) is the immediate precursor of AsA and is oxidized to AsA in mitochondria by L-GalL dehydrogenase (L-GalLDH) using cytochrome c as the electron acceptor (Østergaard et al., 1997; Imai et al., 1998; Yabuta et al., 2000). Wheeler et al. (1998) have described a novel biosynthetic pathway for AsA in higher plants by discovering L-galactose dehydrogenase (L-GalDH). The pathway, named the Smirnoff–Wheeler pathway, proposes that L-GalL is formed via L-Gal, which is derived from D-mannose (D-Man). Functional in planta evidence for the Smirnoff–Wheeler pathway was provided by Arabidopsis mutants such as those with mutations of the VTC1 and VTC4 genes, which encode GDP-D-Man pyrophosphorylase (GMP) and L-Gal 1-P phosphatase (GPP), respectively (Conklin et al., 1999, 2006). The vtc2 mutants are also affected in a novel enzyme, GDP-L-Gal phosphorylase (N Smirnoff, personal communication), so that almost all the enzymes related to the pathway, including GDP-D-Man-3′,5′-epimerase (GME), have been identified and analysed in detail.
Another proposed pathway for AsA biosynthesis in plants includes D-galacturonic acid (D-GalUA) as a precursor of L-GalL. Agius et al. (2003) demonstrated the existence of this pathway by showing a correlation between the D-GalUA reductase, a key enzyme of this pathway, and the AsA level of the developing flowers and fruit in strawberry. However, the D-GalUA pathway seems to be a salvage mechanism for carbon resulting from the breakdown of cell walls, such as occurs during fruit ripening (Agius et al., 2003). Radiotracer experiments also indicate that the D-GalUA pathway could only contribute a minor part of AsA synthesis in this tissue (Loewus, 1963; Smirnoff, 2003).
Because plant AsA biosynthesis pathways have become clear, the regulation mechanism of AsA pool size and the expression and regulatory properties of the enzymes involved are the next important subjects to resolve (Smirnoff et al., 2001; Ishikawa et al., 2006). A feedback control in AsA biosynthesis has been demonstrated by Pallanca and Smirnoff (2000). They observed that the rate of AsA biosynthesis from [U-14C]D-Glu decreased linearly with the increase in the pool size of AsA in embryonic pea seedlings. Furthermore, in the presence of AsA, a decrease in incorporation of the [14C]D-Man label into AsA was observed in Arabidopsis cell suspension cultures, indicating that AsA inhibited its own biosynthesis by a feedback control at the level of GME (Wolucka and Van Montagu, 2003). Recently, it was found that spinach L-GalDH is competitively inhibited by AsA (Mieda et al., 2004). These results strongly suggest that the AsA level in plant cells is regulated at several steps by feedback control. However, the mechanisms of the feedback control of AsA are not fully understood. In addition, it has been reported that other biological factors, such as jasmonic acid, contribute to accumulation of AsA (Reinbothe et al., 1994; Turner et al., 2002; Wolucka et al., 2005), although the mechanism has not been clarified yet.
The role of AsA in photosynthesis is well recognized as a photoprotectant in scavenging the deleterious active oxygen species that are generated as by-products during photosynthesis and as a key component in excess photonic energy dissipation mechanisms, such as the water–water cycle (Asada, 1999) and the xanthophyll cycle (Muller-Moule et al., 2002). Accordingly, leaves exposed to light contain more AsA than leaves in the shade (Grace and Logan, 1996; Smirnoff and Pallanca, 1996), and AsA levels in the leaves show a diurnal rhythm (Dutilleul et al., 2003; Tamaoki et al., 2003). However, the mechanism of light regulation of AsA pool size in plants is unknown. In this work, the relationship between gene expression levels related to AsA biosynthesis and photosynthesis in leaves of Arabidopsis plants was studied. Comparing the AsA level in leaves of wild-type plants with that in the sugar-insensitive mutant abi4/sun6, the AsA pool size was only affected by photosynthesis ability, not sugar levels in leaves. The results suggest that light regulation of AsA biosynthesis is dependent on the photosynthetic electron transport chain but independent of sugars in Arabidopsis.
Materials and methods
Plant material and growth conditions
Arabidopsis thaliana ecotype Columbia seedlings were grown on a Rock Fiber (Nittobo, Tokyo, Japan) containing inorganic salts (Murashige and Skoog, 1962) and 0.5 g l−1 2-morpholinoethanesulphonic acid (MES) (pH 5.7). After 2 d of stratification in the cold (4 °C), the plates were incubated in growth chambers under 16 h of 100 μmol m−2 s−1 light (25 °C) and 8 h of darkness (20 °C). Seeds of the Arabidopsis mutant, abi4/sun6, were obtained from the Nottingham Arabidopsis Stock Centre, Loughborough, UK.
RNA extraction and quantitative RT-PCR analysis
Total RNA was isolated from the rosette leaves of Arabidopsis seedlings (1.0 g fresh weight) as described previously (Yabuta et al., 2002). To eliminate any DNA contamination, 50 μg of total RNA was treated with DNase I (Takara, Ohtsu, Japan). The total RNA was purified with an RNeasy Plant Mini Kit (Qiagen) and converted into cDNA using ReverTra Ace (Toyobo, Osaka, Japan) with an oligo(dT)20 primer. Primer pairs for the quantitative reverse transcription-PCR (RT-PCR) were designed using Primer Express software (Applied Biosystems), and primer sequences are shown in Supplementary Table S1 at JXB online. Gene-specific primers were chosen such that the resulting PCR product had an approximately equal size of 100 bp. The quantitative RT-PCR was performed with an Applied Biosystems 7300 Real Time PCR System (Applied Biosystems), using the SYBR Premix Ex Taq (Takara). Actin2 mRNA, set to 100%, was used as an internal standard in all experiments. The quantitative RT-PCR experiments were repeated at least three times for cDNA prepared from three batches of plants.
Estimation of CO2 fixation rate
Net CO2 assimilation rates of leaves were measured by a modification of the method described previously (Miyagawa et al., 2001) using a Li-Cor LI-6400 (Lincoln, NE, USA) apparatus with the following measuring cell (6400-15 Arabidopsis Chamber, 0.785 cm2, Lincoln) parameters: 25 °C, 100 μmol m−2 s−1, 360 ppm CO2, 60% relative humidity, and an air flow of 200 ml s−1.
Leaf chlorophyll fluorescence measurements
Chlorophyll a fluorescence parameters were measured in leaves with a MINI PAM Chlorophyll Fluorometer (Walz, Effeltrich, Germany) as described by Schreiber et al. (1986). The fluorescence parameters were measured with saturating pulses of white light of approximately 2000 μmol m−2 s−1 and actinic white light of 100 μmol m−2 s−1. Maximum chlorophyll a fluorescence yield (Fm) was recorded during a short saturating pulse (0.8 s), which was applied 10 s after a leaf was first exposed to light (measurements of F0, i.e. minimum chlorophyll a fluorescence yield, were recorded at very low light intensity, i.e. <1 μmol m−2 s−1). Actinic light was applied directly after the saturating pulse. During the actinic light exposure, saturating pulses (0.8 s) were applied at 30 s intervals (F′m measurements). Parameters F′m and F′0 are light-adapted equivalent terms to Fm and F0. Variable fluorescence, Fv, is the difference between Fm and F0. ΦPSII was used as an indicator of quantum yield of electron transport from water through photosystem II.
Measurement of AsA and DHA levels
AsA and dehydroascorbate (DHA) were measured as described by Wise and Naylor (1987). The rosette leaves of Arabidopsis seedlings (0.5 g wet weight) frozen in liquid N2 were ground using a mortar and pestle with 5 ml of 6% (v/v) HClO4 and centrifuged at 10 000 g for 10 min at 4 °C. A 100 μl aliquot of the obtained leaf extract was added directly to 900 μl of a 200 mM succinate buffer (pH 12.7, adjusted with NaOH) in the spectrophotometer. The final pH was very near 6.0. The A265 was immediately recorded once, and again 5 min after the addition of 5 U of AsA oxidase from Cucurbita sp. (Wako, Osaka, Japan). For the determination of total AsA, the leaf extract was adjusted to pH 6.0 with 1.25 M K2CO3 and centrifuged at 10 000 g for 5 min. The supernatant was incubated with 10 mM dithiothreitol (DTT) dissolved in 50 mM 4-(2-hydroxyethyl)-1-piperazine ethanesulphonic acid (HEPES)-KOH buffer (pH 7.5) for 30 min at 25 °C. A 100 μl aliquot of the solution was directly added to 900 μl of 200 mM succinate buffer (pH 6.0) in the spectrophotometer. The resultant solution was assayed as described above. The difference between the total AsA and AsA contents was taken to be the DHA content.
Measurement of sugar levels
D-Glu, D-Fru, and Suc levels were estimated by enzymatic assays as described (Leegood, 1993). Briefly, the rosette leaves of Arabidopsis seedlings were ground to a fine powder in liquid nitrogen using a mortar and pestle. The powdered sample was then homogenized thoroughly in 6% (v/v) HClO4 (2 ml g−1 FW) and centrifuged at 27 000 g for 10 min. The clarified supernatant was neutralized to pH 7.0 with 5 N KOH containing 1 M triethanolamine, and 0.03 g ml−1 of charcoal was added. After rigorous vortex mixing, the sample was centrifuged again at 27 000 g for 10 min. The supernatant obtained was used for the estimation of the sugars. First, D-Glu was estimated using a coupling reaction that involved the conversion of D-Glu to D-Glu-6-phosphate and then oxidation by D-Glu-6-phosphate dehydrogenase using NADP+ as the electron acceptor. The reaction mixture contained 1.5 mM MgCl2, 1.1 mM ATP, 0.5 mM NADP+, 0.5 U of hexokinase in 100 mM TRIS-HCl buffer (pH 6.9), and 10 μl of sample in a final volume of 1 ml. The reaction was started by adding 2 U of D-Glu-6-phosphate dehydrogenase and incubated at 30 °C for approximately 10 min, monitoring the optical density at 340 nm. The amount of glucose was calculated from a calibration curve prepared with standard D-Glu in the range of 0.5–5 mM. For the estimation of D-Fru, 2 U of phosphoglucose isomerase was included in the incubation mixture. Suc was assayed after hydrolysing with 20 U of invertase in the same incubation mixture. The amounts of D-Fru and Suc were calculated from the increases in the D-Glu values obtained after the inclusion of phosphoglucose isomerase and invertase, respectively. Recovery of D-Glu, D-Fru, and Suc was estimated by adding known amounts of these sugars to the samples. L-Gal was measured using L-GalDH as described by Gatzek et al. (2002). Briefly, the rosette leaves of Arabidopsis seedlings were ground to a fine powder in liquid nitrogen using a mortar and pestle. The powdered sample was then homogenized thoroughly in butanol-saturated 1 M formic acid (5 ml g−1 FW) and centrifuged at 27 000 g for 10 min. After centrifugation, the pellets were re-extracted twice and the combined extracts freeze-dried. The residues were dissolved in 5 ml of water. These samples were incubated with 1 ml of 50 mM TRIS-HCl (pH 8.5) containing 0.2 mM NAD+ and recombinant L-GalDH for 1 h at 37 °C. The reaction was stopped by boiling for 2 min. The formation of NADH was measured by fluorescence (λex=340 nm, λem=460 nm). Recombinant L-GalDH was prepared as described previously (Mieda et al., 2004).
Data analysis
The significance of differences between data sets was evaluated by t-test. Calculations were carried out with Microsoft Excel software.
Results
Effect of light on AsA biosynthesis
When 2-week-old Arabidopsis plants grown under a 16 h daily photoperiod were moved to the dark, the AsA level in the leaves decreased steadily (Fig. 1A). After 3 d in the dark, the leaf AsA level decreased by 91%. On the other hand, the AsA level of the leaves was increased by 171% in the plants exposed to continuous light for 3 d. However, the DHA level of the leaves was the same in both light and darkness. To understand the mechanism of decline in the leaf AsA level in darkness, the levels of transcripts of various enzymes involved in the plant AsA biosynthesis pathway were verified (Fig. 1B). There were no significant differences in the transcript levels of GME and L-GalDH under light or darkness. The transcript level of L-GalLDH decreased under darkness. The transcript level of GMP and GPP increased under continuous light and decreased under darkness. Interestingly, VTC2 showed a similar transcriptional pattern to that of GMP and GPP.
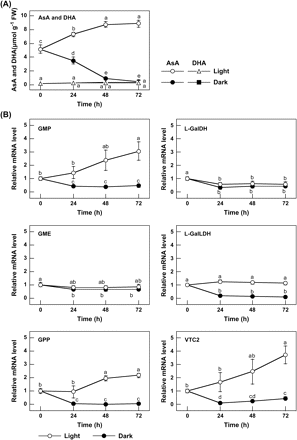
Effect of light on the AsA level and the expression of enzymes of the AsA biosynthesis pathway in Arabidopsis leaves. (A) AsA and DHA levels. Two-week-old Arabidopsis plants grown under 16 h of light at 100 μmol m−2 s−1 and 8 h of darkness were moved into the dark, or exposed to continuous light at 100 μmol m−2 s−1. The analysis started after 8 h of darkness (corresponding to the time of starting the light period), and then the leaves were extracted and assayed for the levels of AsA and DHA at the indicated times. Data are the mean values ±SD of three individual experiments (n=3). Different letters indicate significant differences (P <0.05). (B) Transcript levels of enzymes of the AsA biosynthesis pathway. Total RNA was extracted from the leaves of the above samples and then reverse transcribed into cDNA and used as templates for quantitative RT-PCR with specific primers designed from the coding sequences of the respective enzymes and Actin2, as detailed in the Materials and methods. For each sample, the transcript levels were normalized with those of Actin2. Data are the mean values ±SD of three independent experiments (n=3). Values without a common letter are significantly different according to t-test (P <0.05).
To understand the connection between photosynthesis and the AsA level, inhibitors of photosynthetic electron transport, 3-(3,4-dichlorophenyl)-1,1-dimethylurea (DCMU) and atrazine (ATZ), which block the electron flow toward plastoquinones in PSII were applied. Arabidopsis plants were pretreated during the dark period and then shifted to continuous light for the next 24 h. The treatment of plants with 10 μM DCMU or 10 μM ATZ significantly decreased the PSII quantum yield (expressed as ΦPSII) after 24 h under continuous light conditions (Fig. 2). Interestingly, the leaf AsA level showed no significant difference at 24 h even under exposure to continuous light, whereas in the control plants it was increased by 132% (Fig. 2). The results clearly indicated that the inhibition of photosynthetic electron transport resulted in the suppression of increase in the leaf AsA pool size. The treatment with DCMU and ATZ arrested the induction of the transcript levels of GMP, GPP, L-GalLDH, and VTC2. The transcript level of EIF4A, a translational initiation factor, was not affected by the treatment with these inhibitors (Fig. 2).
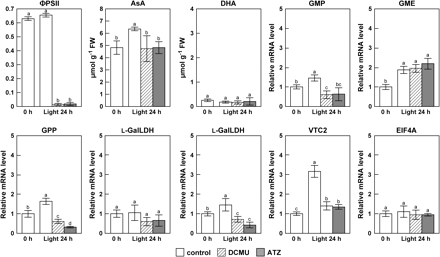
Effect of DCMU and ATZ inhibitors of photosynthetic electron transport on the PSII quantum yield (ΦPSII), the AsA level, and the expression of enzymes of the AsA biosynthesis pathway in leaves. Two-week-old Arabidopsis plants grown under 16 h of light at 100 μmol m−2 s−1 and 8 h of darkness were treated with 10 μM DCMU, 10 μM, ATZ or water (Control) during the dark period and then shifted to continuous light (100 μmol m−2 s−1) for the next 24 h. The above samples were analysed for the PSII activity, AsA levels, and transcript levels of the indicated enzymes by quantitative RT-PCR as detailed in the Materials and methods. The analysis started after 16 h of light (corresponding to the time of starting the dark period). Chlorophyll fluorescence parameters of leaves at the indicated times were measured with a chlorophyll fluorometer under the following conditions: 100 μmol m−2 s−1, 360 ppm CO2, 60% relative humidity at 25 °C. Data are the mean values ±SD of three individual experiments (n=3). Values without a common letter are significantly different according to t-test (P <0.05).
Effect of sugars on AsA biosynthesis
To examine the effect of sugars on the leaf AsA level, 2-week-old Arabidopsis plants were transferred to a medium with or without 0.5% (w/v) sucrose (Suc). The leaf D-glucose (D-Glu), D-fructose (D-Fru), and Suc levels declined by >90% in 48 h under darkness in both the plants that were not supplied with Suc (Suc− plants) and the plants supplied with Suc externally (Suc+ plants) (data not shown). External Suc had no effect on the decline of leaf AsA level in the dark for 48 h (data not shown). However, when the Suc+ and Suc− plants were returned to the light after 48 h in the dark, the levels of D-Glu, D-Fru, and Suc increased in both plants (Fig. 3A). In the Suc+ plants, the leaf D-Glu, D-Fru, and Suc levels were significantly higher than those in the Suc− plants during the next 48 h under light. Interestingly, in spite of the elevated levels of sugars, the leaf AsA levels in the Suc+ plants were 50% lower than those in the Suc− plants after 48 h in the light. On the other hand, AsA levels in the leaves of Suc− plants increased steadily and were restored to normal levels in 48 h under light. The DHA level of leaves was unaffected by the external Suc in the dark or light.
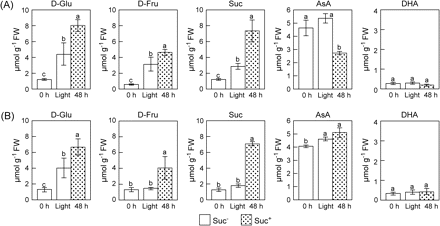
Effects of light and Suc on the levels of sugars and AsA in leaves of wild-type (A) and abi4/sun6 mutants (B). Two-week-old Arabodopsis plants and abi4/sun6 mutants grown under 16 h of light at 100 μmol m−2 s−1 and 8 h of darkness were transferred into a medium without Suc (Suc−) or with 0.5% (w/v) Suc (Suc+), and then moved into the dark. After 48 h of darkness, the plants were exposed to continuous light at 100 μmol m−2 s−1 for 48 h. At the indicated times, the leaves were extracted and assayed for the levels of D-Glu, D-Fru, Suc, AsA, and DHA. Data are the mean values ±SD of three individual experiments (n=3). Values without a common letter are significantly different according to t-test (P <0.05).
To verify the relationship between AsA pool size and the transcriptional levels of AsA biosynthesis enzymes in the leaves of Suc+ and Suc− plants, a quantitative reverse transcription-PCR (RT-PCR) analysis was performed. As a result, the transcription profiles of GMP, GPP, L-GalLDH, and VTC2 that can be seen in the dark/light change were unaffected by the external Suc (see Supplementary Fig. S1 at JXB online). In contrast to the result of AsA levels in Suc+ and Suc− plants under light conditions, the transcript levels of VTC2 and L-GalLDH in the leaves of Suc+ plants were slightly higher than those in Suc− plants, and no significant difference was found in the transcript levels of GME or L-GalDH between Suc+ and Suc− plants. Transcript levels of AsA–GSH cycle enzymes (APX, ascorbate peroxidase; AAO, ascorbate oxidase; MDAR, monodehydroascorbate reductase; DHAR, dehydroascorbate reductase; GR, glutathione reductase) were also evaluated under the same conditions by carrying out a quantitative RT-PCR analysis. The transcript levels of MDAR2, MDAR5, DHAR3, DHAR4, DHAR5, and GR1 in the leaves of Suc+ plants were significantly higher than those in the leaves of Suc− plants, and the transcription profiles of other genes were unaffected by the external Suc (Fig. 5). Overall, the expression profiles of the six genes in the leaves of Suc+ plants tended to be inversely proportional to the leaf AsA levels between Suc+ and Suc− plants, suggesting that the genes might not be associated with the control of AsA levels and contribute to a protection against oxidative stress caused by the low AsA level in the leaves.
APX2, DHAR2, and AAO2 were not expressed under the present experimental conditions (data not shown). Based on the results of gene expression analysis in Suc-feeding plants, it might be possible to estimate that sugar contents take part in control of the gene expression levels for some kinds of AsA biosynthesis and regeneration enzymes.
Effect of a sugar-insensitive mutant on both AsA biosynthesis and photosynthesis
It has been reported that cellular sugar levels control the photosynthesis process in plants (Van Oosten et al., 1997; Huijser et al., 2000; Rolland et al., 2002). Lower leaf AsA levels in the plants treated with DCMU and ATZ, and in the Suc+ plants with elevated sugar levels prompted a study of the relationship between AsA biosynthesis and photosynthesis. As shown in Fig. 4A, the rate of CO2 fixation and the ΦPSII in the Suc+ plants were significantly lower than those in the Suc− plants after 48 h of exposure to light following 48 h darkness.
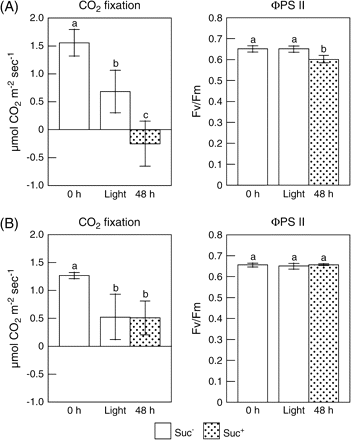
Effects of light and Suc on the CO2 fixation and the PSII quantum yield in leaves of wild-type (A) and abi4/sun6 mutants (B). Two-week-old Arabidopsis plants and abi4/sun6 mutants grown under 16 h of light at 100 μmol m−2 s−1 and 8 h of darkness were transferred into a medium without Suc (Suc−) or with 0.5% Suc (Suc+), and then moved into the dark. After 48 h of darkness, the plants were exposed to continuous light at 100 μmol m−2 s−1 for 48 h. Net CO2 fixation rates in leaves at the indicated times were measured with a photosynthesis measuring system under the following conditions: 100 μmol m−2 s−1, 360 ppm CO2, 60% relative humidity at 25 °C. Chlorophyll fluorescence parameters of leaves at the indicated times were measured with a chlorophyll fluorometer under the same conditions. Data are the mean values ±SD of five individual experiments (n=5). Values without a common letter are significantly different according to t-test (P <0.05).
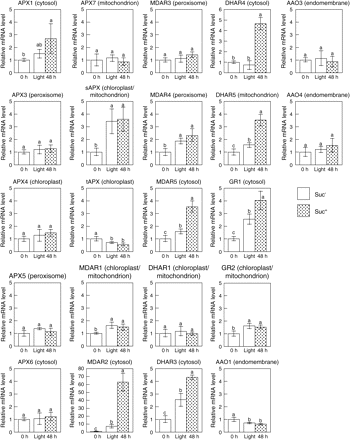
Effects of light and Suc on the expression of enzymes of the AsA–GSH cycle in leaves of wild-type plants. Two-week-old Arabidopsis plants grown under 16 h of light at 100 μmol m−2 s−1 and 8 h of darkness were transferred into a medium without Suc (Suc−) or with 0.5% Suc (Suc+), and then moved into the dark (Dark). After 48 h of darkness, the plants were exposed to continuous light at 100 μmol m−2 s−1 (Light) for 48 h. At the indicated times, leaf samples were analysed for transcript levels of the indicated enzymes by quantitative RT-PCR as detailed in the Materials and methods. Data are the mean values ±SD of three individual experiments (n=3). Values without a common letter are significantly different according to t-test (P <0.05).
It is well known that the addition of external sugar leads to a reduction in the photosynthetic activity accompanied by a decrease of transcript levels of plastocyanin, chlorophyll a/b-binding protein and the small subunit of Rubisco (Van Oosten et al., 1997; Oswald et al., 2001). In the Arabidopsis Suc-uncoupled mutant, sun6, photosynthesis is insensitive to high sugar levels due to a mutation in a hexokinase-dependent sugar response pathway (Van Oosten et al., 1997; Huijser et al., 2000). The SUN6 gene was cloned and analysis showed it to be identical to the previously described ABSCISIC ACID INSENSITIVE-4 (ABI4) gene (Huijser et al., 2000). Therefore, the effects of external Suc on the levels of sugar and AsA were analysed in leaves of abi4/sun6 mutants. The leaf D-Glu, D-Fru, and Suc levels declined by 77, 93, and 89%, respectively, in 48 h under darkness in both the Suc− and Suc+ mutants (data not shown). The CO2 fixation and ΦPSII in abi4/sun6 plants were not affected by the external Suc (Fig. 4B). Interestingly, as shown in Fig. 3B, the leaf AsA levels in the abi4/sun6 mutants on the Suc+ medium increased upon exposure to light at almost the same rate as those in the plants on the medium without Suc (Suc−). To evaluate the relationship between metabolic flux of the Smirnoff–Wheeler pathway and AsA level, the leaf L-Gal level was compared in abi4/sun6 and wild-type plants on Suc+ and Suc− medium after 24 h under light. The levels of L-Gal in leaves of abi4/sun6 plants on Suc+ and Suc− medium were 55.3±3.4 nmol g−1 FW and 25.7±2.0 nmol g−1 FW, respectively, indicating that the L-Gal level in the Suc+ mutants was approximately 2.2-fold higher than that in the Suc− mutants. Similar results were observed in the wild-type plants; the levels of L-Gal on Suc+ and Suc− medium were 57.1±2.7 nmol g−1 and 31.0±8.0 nmol g−1, respectively. Furthermore, the transcription profiles of the enzymes in the AsA biosynthesis pathway of the mutant were quite similar to those in the wild type and were unaffected by the external Suc (see Supplementary Fig. S2 at JXB online), suggesting that the control of the leaf AsA pool size is closely linked to photosynthesis ability.
Discussion
In this work the relationship between gene expression levels related to AsA biosynthesis and photosynthesis in Arabidopsis leaves has been studied. It is well known that leaf AsA levels depend on the light/dark conditions (Tabata et al., 2002; Fig. 1A). The AsA levels in leaves show a diurnal rhythm (Dutilleul et al., 2003; Tamaoki et al., 2003) and vary with the daylight conditions associated with long-term seasonal variations (Grace and Logan, 1996). Because AsA is highly unstable in its oxidized form (Pallanca and Smirnoff, 2000; Chen et al., 2003), even to maintain a constant AsA pool level the rate of biosynthesis of AsA would need to be increased during the episodes of high light. Even though the role of AsA in photosynthesis is well recognized (Asada, 1999; Niyogi, 1999; Shigeoka et al., 2002), the mechanism of the regulation of the AsA pool size in plants by light is not known. Tamaoki et al. (2003) reported that transcript levels of L-GalLDH in the young rosette leaves of Arabidopsis plants increase by approximately 2-fold during the day time. In contrast, no significant changes were observed in the transcript levels of L-GalLDH in tobacco leaves during the day–night cycles, whereas the leaf AsA level increased nearly 4-fold by the end of 16 h of daylight (Pignocchi et al., 2003). Also, Arabidopsis plants grown under high light contained 3-fold higher AsA levels compared with those in plants grown under low-light conditions, whereas there were no significant differences in the L-GalDH activity (Gatzek et al., 2002). In the present study, the AsA pool size in the leaves increased under continuous light and decreased under darkness (Fig. 1A). No significant differences were observed in the transcript levels of GME and L-GalDH under light and darkness (Fig. 1B). However, the transcript levels of GMP, GPP, L-GalLDH, and VTC2 changed in parallel with the changes in leaf AsA pool size during the dark and light periods (Fig. 1B). A mutation in the GMP gene that causes a decrease in GMP activity leads to a decreased level of AsA in an Arabidopsis mutant vtc1 (Conklin et al., 1999). A similar observation has been reported where an antisense suppression of GMP reduced the AsA level in transgenic potato plants (Keller et al., 1999). Furthermore, an antisense suppression of L-GalLDH led to decreased levels of AsA in tobacco BY-2 cells (Tabata et al., 2001). These findings, including the present data, suggest that the decrease in AsA pool size during darkness is at least caused by the down-regulation of L-GalLDH and GMP in Arabidopsis plants.
The photosynthetic electron transport, more specifically the plastoquinone redox status, is a cue for the regulation of a host of plastid and nuclear genes that encode the core proteins of PSI and II (Pfannschmidt et al., 1999), the photosynthetic electron transport itself (Petracek et al., 1998; Oswald et al., 2001), photomorphogenesis (Montane et al., 1998), and antioxidant enzymes (Karpinski et al., 1997, 1999; Madhusudhan et al., 2003; Yabuta et al., 2004). Therefore, the effects of inhibitors of photosynthesis on the levels of AsA in leaves were checked (Fig. 2). DCMU and ATZ treatment mimicked the effect of darkness, causing down-regulation of GMP, GPP, L-GalLDH, and VTC2 as well as the arrest of the increase in leaf AsA level. These results indicated that the gene expression levels are markedly affected by the redox status of the plastoquinone pool, and the changes of AsA level due to light should be dominantly controlled by photosynthesis itself, but not by the up-regulation of AsA biosynthesis genes.
Because AsA is one of the most abundant soluble carbohydrates in leaves, significant amounts of carbon source would be required to sustain its synthesis. Therefore, the effect of the supply of an external carbon source on the leaf AsA level was explored. In 48 h under darkness, leaf sugar levels declined by >90%, which accompanied the decline in AsA level. However, even though Suc+ plants had significantly higher leaf D-Glc, D-Fru, and Suc levels than those in the Suc− plants under light, AsA levels were not restored to normal levels. Taking into account the fact that the transcription levels of some enzymes in the Smirnoff–Wheeler pathway were unaffected by the external Suc (see Supplementary Fig. S1 at JXB online), it might be possible to consider that there is potential post-transcriptional regulation of the enzymes in the pathway or a possible influence of AsA degradation in determining AsA contents. The external supply of Suc incidently suppressed the photosynthesis in the Suc+ plants, as evident from the low CO2 fixation rates in these plants (Fig. 4A). The transcription levels of nuclear-encoded photosynthesis and glyoxylate cycle genes in leaves are regulated by the levels of hexose sugars sensed by hexokinase (Jang et al., 1997; Oswald et al., 2001). Furthermore, the ΦPSII in the leaves of Suc+ plants was significantly lower than that of Suc− plants (Fig. 4A). The fact that the DCMU or ATZ treatment, which blocks the reduction of the plastoquinone pool, caused the arrest of the increase in leaf AsA level also indicated that the photosynthetic electron transport is a strong factor for AsA pool size control. Because AsA biosynthesis starts from D-Glu 6-phosphate rather than D-Glu (Smirnoff and Wheeler, 2000), it is noteworthy that the effects of the PSII inhibitors could be mediated via inhibition of sugar-phosphate export from chloroplasts.
It has been demonstrated that the expression of photosynthesis genes and the rate of photosynthetic activity are insensitive to high Suc or D-Glc levels in abi4/sun6 seedlings (Van Oosten et al., 1997; Oswald et al., 2001). The ABI4/SUN6 gene encodes the transcription factor characterized by a conserved DNA-binding domain, the APETALA 2 domain (Finkelstein et al., 1998; Huijser et al., 2000). The leaf AsA pool size in the mutant was not affected even on the Suc+ medium (Fig. 3B), and the transcription profiles of the enzymes in the AsA biosynthesis pathway were quite similar to those in the wild type (see Supplementary Fig. S2 at JXB online). The leaf AsA pool size in the Suc-fed abi4/sun6 mutant plants also supports the dependence on photosynthesis. It is worth noting that the leaf L-Gal levels in both the mutant and wild-type plants are increased by the supply of Suc (Suc+), indicating that an enlargement of the flux of the Smirnoff–Wheeler pathway might not be the critical factor for the pool size of AsA. Supplementation with AsA precursors, such as L-GalL and L-Gal, to plants under dark conditions did not show any significant increase in the cellular AsA level, also supporting this view (Y Yabuta et al., unpublished data). On the other hand, because AsA biosynthesis starts from D-Glu 6-phosphate as mentioned above, one could consider that there might not be an absolute correspondence between sugar-phosphate supply from the chloroplast and the free sugar contents measured.
In conclusion, it has been demonstrated that photosynthetic electron transport is closely linked to the regulation of the AsA pool size in leaves, and that the role of photosynthesis in AsA biosynthesis is not merely to provide the carbon source. However, the signalling pathway and the control mechanism leading to the regulation of the AsA pool size need to be clarified.
Supplementary material
Primer pairs used for the quantitative PCR analysis and results of transcription profiles for the AsA biosynthesis pathway are shown in Supplementary Table S1, Fig. S1, and Fig. S2, respectively, at JXB online.
Abbreviations
- AAO
ascorbate oxidase
- APX
ascorbate peroxidase
- ATZ
atrazine
- AsA
l-ascorbate
- DCMU
3-(3,4-dichlorophenyl)-1,1-dimethylurea
- DHA
dehydroascorbate
- DHAR
dehydroascorbate reductase
- d-Fru
d-fructose
- l-Gal
l-galactose
- l-GalDH
l-galactose dehydrogenase
- l-GalL
l-galactono-1,4-lactone
- l-GalLDH
l-galactono-1,4-lactone dehydrogenase
- d-GalUA
d-galacturonic acid
- d-Glu
d-glucose
- GME
GDP-d-mannose-3′,5′-epimerase
- GMP
GDP-d-mannose pyrophosphorylase
- GPP
l-galactose 1-P phosphatase
- GR
glutathione reductase
- d-Man
d-mannose
- MDAR
monodehydroascorbate reductase
- Suc
sucrose
This work was supported by CREST, JST, and by a Grant-in-Aid for Scientific Research (grant no 15380078 to SS) from MEXT, Japan. This work was also supported in part by the ‘Academic Frontier’ Project for Private Universities: matching fund subsidy from MEXT, 2004–2008.
References
Author notes
These authors contributed equally to this work.
Comments