-
PDF
- Split View
-
Views
-
Cite
Cite
Anke Buchholz, Characterization of the diffusion of non-electrolytes across plant cuticles: properties of the lipophilic pathway, Journal of Experimental Botany, Volume 57, Issue 11, August 2006, Pages 2501–2513, https://doi.org/10.1093/jxb/erl023
- Share Icon Share
Abstract
Systemic crop protection products are commonly sprayed onto foliage, whereupon the active substances must penetrate into the leaves in order to become biologically active. Penetration of the plant cuticle is the rate-limiting step. The diffusion of organic non-electrolytes within cuticles is a purely physical process that can be described and analysed in the same way as is done for diffusion in synthetic polymer membranes. Solute mobilities in cuticles vary considerably between plant species. For a given species they decrease with increasing solute size, and this size selectivity holds for all of the plant species investigated so far. Wax extraction from leaf cuticles increases the mobility of solutes tremendously, but size selectivity is not affected. Furthermore, diffusion within plant cuticles is extremely temperature dependent. An analogous increase in solute mobility can be achieved by using accelerators, which enhance the fluidity of the polymer matrix and of the waxes. The effects of temperature and plasticizers on the diffusion of non-electrolytes in wax and the cutin matrix have been used to characterize the nature of the lipophilic pathway. The ‘free volume’ theory can be used to explain the influence of the size and shape of the solute, and its dependence on temperature. The physico-chemical nature of the diffusion pathway has been shown, by thermodynamic analysis, to be identical for a wide range of solute lipophilicities. This approach also explains the mode of action and the intrinsic activity of plasticizers.
Abbreviations
- CM
cuticular membrane
- k*
solute mobility
- MX
matrix membrane
- UDOS
unilateral desorption of the outer surface
- Vx
molar volume
Introduction
Foliar uptake is a complex process determined by several mutually affecting parameters. General characteristics—required for the optimization of foliar uptake—can only be obtained when interdependencies have been excluded. Extensive work, focusing on the diffusion of non-electrolytes across plant cuticles, has been done, which this paper attempts to review. The relevant characterization of cuticle penetration for polar compounds is given separately by Schönherr (2006). After a general introduction on the function and character of plant cuticles, the applied methodology is presented. Parameters affecting plant cuticle penetration are displayed individually so that finally the complex scenario of foliar uptake can be outlined in a simplified way.
Function of plant cuticles
All aerial surfaces of the primary parts of terrestrial higher plants are covered with a cuticle, the function of which is to prevent uncontrolled water loss (Riederer and Schreiber, 2001). The plant cuticle is not only exposed to abiotic factors like sunlight, wind, rain, etc. but it also interacts with microbes, fungi, and insects (Juniper, 1995; Kolattukudy et al., 1995; Kerstiens, 1996; Schreiber et al., 2004). Furthermore, the plant cuticle is the initial contact point between an agrochemical and the plant and, where uptake into plant tissues is required for biological activity, it is the main barrier to penetration.
Morphology, chemistry, and structure of plant cuticles
Cuticles are not simple homogeneous structures like synthetic polymer films. Various structural types occur in gymnosperms and angiosperms, which were categorized by Holloway (1982) into six morphological types. Investigators of the cuticular ultrastructure of different plant species have used different terms to describe these layered structures. Wattendorf and Holloway (1980) provided a comparative overview of the different terminologies used. The following generalization as given by Jeffree (1986) (Fig. 1) distinguishes three main zones. The cuticle proper with epicuticular and intracuticular waxes often has a lamellate layer and typically extends to a thickness of 50–150 nm (Jeffree, 1996). The cuticular layer is bonded to the periclinal walls of the epidermal cells by a pectin-rich layer. This pectinaceous layer is equivalent to the middle lamella, with which it is continuous. Enzymatic hydrolysis of this layer enables the cuticular membranes (Orgell, 1955), which comprise the cuticle proper and the cuticular layer, to be isolated.
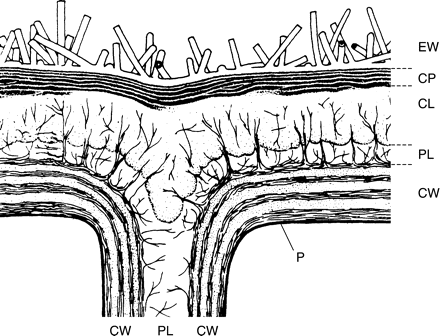
Generalized structure of a plant cuticle (modified according to Jeffree, 1986). EW, Epicuticular wax; CP, cuticle proper with lamellate structure; CL, cuticular layer traversed by cellulose microfibrils; PL, pectinaceous layer and middle lamella; CW, cell wall; P, plasmalemma.
The epicuticular wax film is a distinct layer on the surface of the cutin matrix (Jetter et al., 2000). Depending on their chemical composition, epicuticular waxes may form an amorphous film, granules, or crystalline structures of various shapes (Baker, 1982; Jeffree, 1986). This fine-surface structure might be modified with increasing age (Bukovac et al., 1979), by environmental stress (Hoad et al., 1992), and also by agrochemical spray solutions (Whitehouse et al., 1982; Tamura et al., 2001). Epicuticular waxes have a considerable influence on the wettability of plant surfaces, often causing poor retention or spreading of spray droplets (Bukovac et al., 1979; Turunen and Huttunen, 1990; Holloway, 1994), but they rarely affect a compound's rate of penetration into leaves (Baur, 1998). The penetration barrier (Schönherr and Schmidt, 1979) is made up of the cuticular membrane, which consists of the cutin polymer matrix (Holloway, 1993) and associated waxes (Bianchi, 1995). However, the permeability of this barrier is independent of the overall thickness of the cuticle (Becker et al., 1986; Knoche et al., 2000). The intracuticular waxes are predominantly located in the outer layers of the cuticle where they form the transport-limiting layer or skin (Schönherr and Riederer, 1988). This wax layer acts as a barrier to both diffusion and solubility (Shafer and Schönherr, 1985), and it has been established that the solid and crystalline wax aggregates within this layer determine the transport properties of the plant cuticle (Riederer and Schreiber, 1995).
Penetration across plant cuticles
The penetration of non-electrolytes across plant cuticles requires three steps: sorption into the cuticular lipids, diffusion across the cuticular membrane, and finally desorption into the apoplast of the epidermal cells (Kirkwood, 1999; Schönherr et al., 1999). Rates of penetration depend upon solute mobility in the cuticle and on the driving force (product of partition coefficient and concentration gradient; see also below). Solute mobility can be measured using unilateral desorption from the outer surface (UDOS). This method takes advantage of the fact that cuticles are asymmetric membranes (see above) and distinguishes two functional layers: the limiting skin and the sorption compartment (Fig. 2). In the cuticles studied, the thin limiting skin comprised only about 10% of the total mass of the cuticular membrane (CM), and both sorption capacity and solute mobility were very small. The sorption compartment underneath the limiting skin amounted to about 90% of the mass of the CM, its sorption capacity was high, and solute mobility was much higher than in the limiting skin (Schönherr and Baur, 1994). Model compounds are applied to the morphological inner surface of the CM and are subsequently sorbed in the sorption compartment. When they are homogeneously distributed within the sorption compartment they are unilaterally desorbed from the morphological outer surface.
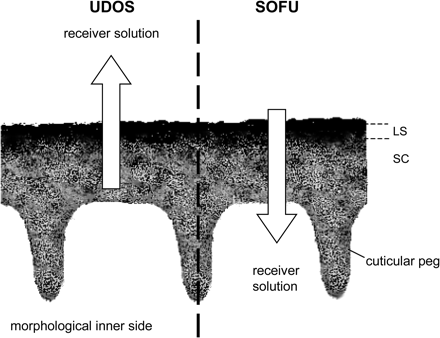
Functional layers (not to scale) of cuticular membranes as differentiated in the UDOS (unilateral desorption of the outer surface) and SOFU (simulation of foliar uptake) methods: limiting skin (LS) and sorption compartment (SC). Occurrence and dimension of cuticular pegs, and protrusion of the cuticular layer into anticlinal cell walls of epidermal cells, are species dependent. The arrows denote the direction of diffusion according to the concentration gradient. For one time, the sorption compartment (UDOS) and, for the other time, the formulation residue facing the limiting skin (SOFU) serve as donors. With UDOS rate constants of desorption, k*, and with SOFU rate constants of penetration, k, can be determined.
The benefit of this method is that the contributions of mobility and solubility to the rates of foliar uptake of pesticides can be determined separately, due to the fact that the measured rate constants of desorption (k*) are independent of the partition coefficient. When the donor is applied to the outer surface of the CM and solutes are desorbed from the morphological inner side (SOFU=simulation of foliar uptake), rates of desorption also follow first-order kinetics, but they depend on both solubility and mobility (Baur et al., 1997b).
Plant species
When solute mobility in cuticles is studied using the UDOS method, astomatous CM must be used. Enzymatic isolation does not affect the transport properties of cuticles (Kirsch et al., 1997) but it is feasible only with a limited number of species, i.e. those where a continuous pectinaceous layer is present. These are most often perennial plants. The range of solute mobilities (e.g. for bifenox) measured for the CMs studied covered three orders of magnitude (Fig. 3). These different mobilities are species-specific characteristics and do not correlate with either cuticle thickness or the amount of wax embedded within, and covering, the cuticular matrix. For example, Pyrus communis, Malus domestica, Ilex aquifolium, and Ilex paraguariensis exhibited a very similar amount of extractable wax (about 24%) but its contribution to the barrier property of the CM varied by factors of 49 up to 4897 (Table 1). No clear relationship has been found to exist between the particular composition of the soluble lipids and the respective cuticular permeability (Haas and Schönherr, 1979; Riederer and Schneider, 1990; Hauke and Schreiber, 1998). Histochemical methods have also been used to try to understand the relationship between chemical composition and permeability (Leece, 1976; Krüger et al., 1996).
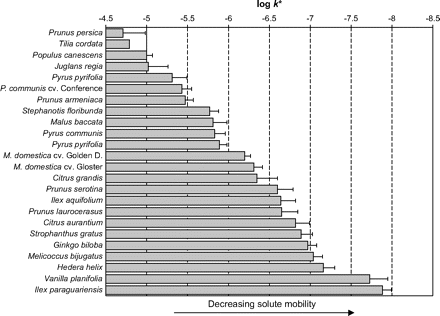
Solute mobilities, logk*, of the model compound bifenox (logKCW, 4.44; molar volume, 216 cm3 mol−1) measured in 24 plant species (CM) at 25 °C. Error bars denote 95% confidence intervals.
Comparison of solute mobilities (k*; ±95% CI) for bifenox within CM and MX of 13 plant species at 25 °C
Plant species | CM log k* | 95% CI | MX log k* | 95% CI | Factor | Wax (mg cm−2) | Wax (%) |
Populus canescens | −5.00 | 0.07 | – | – | – | 0.053 | 33.9 |
Pyrus pyrifolia | −5.34 | 0.17 | −3.29 | 0.05 | 112 | 0.055 | 25.5 |
P. communis cv. Conference | −5.43 | 0.11 | −3.74a | – | 49 | 0.061 | 23.7 |
Stephanotis floribunda | −5.68 | 0.25 | −3.73 | 0.02 | 89 | 0.026 | 10.2 |
M. domestica cv. Golden Delicious | −6.20 | 0.08 | −3.36 | 0.06 | 692 | 0.044 | 23.7 |
Ilex aquifolium | −6.64 | 0.18 | −4.14 | 0.12 | 316 | 0.162 | 24.6 |
Ginkgo biloba | −6.97 | 0.11 | −5.54 | 0.19 | 27 | 0.054 | 13.4 |
Melicoccus bijugatus | −7.04 | 0.11 | −4.81 | 0.06 | 170 | 0.049 | 8.8 |
Hedera helix | −7.16 | 0.14 | −3.75 | 0.05 | 2570 | 0.022 | 10.2 |
Strophanthus gratus | −7.18 | 0.16 | −5.34a | – | 69 | 0.060 | 19.5 |
Vanilla planifolia | −7.67 | 0.11 | −6.93 | 0.06 | 5 | 0.061 | 10.8 |
Ilex paraguariensis | −7.89 | 0.11 | −4.20 | 0.15 | 4897 | 0.114 | 22.3 |
Schefflera actinophylla | −8.09a | – | −4.57a | – | 3311 | 0.084 | 29.8 |
Plant species | CM log k* | 95% CI | MX log k* | 95% CI | Factor | Wax (mg cm−2) | Wax (%) |
Populus canescens | −5.00 | 0.07 | – | – | – | 0.053 | 33.9 |
Pyrus pyrifolia | −5.34 | 0.17 | −3.29 | 0.05 | 112 | 0.055 | 25.5 |
P. communis cv. Conference | −5.43 | 0.11 | −3.74a | – | 49 | 0.061 | 23.7 |
Stephanotis floribunda | −5.68 | 0.25 | −3.73 | 0.02 | 89 | 0.026 | 10.2 |
M. domestica cv. Golden Delicious | −6.20 | 0.08 | −3.36 | 0.06 | 692 | 0.044 | 23.7 |
Ilex aquifolium | −6.64 | 0.18 | −4.14 | 0.12 | 316 | 0.162 | 24.6 |
Ginkgo biloba | −6.97 | 0.11 | −5.54 | 0.19 | 27 | 0.054 | 13.4 |
Melicoccus bijugatus | −7.04 | 0.11 | −4.81 | 0.06 | 170 | 0.049 | 8.8 |
Hedera helix | −7.16 | 0.14 | −3.75 | 0.05 | 2570 | 0.022 | 10.2 |
Strophanthus gratus | −7.18 | 0.16 | −5.34a | – | 69 | 0.060 | 19.5 |
Vanilla planifolia | −7.67 | 0.11 | −6.93 | 0.06 | 5 | 0.061 | 10.8 |
Ilex paraguariensis | −7.89 | 0.11 | −4.20 | 0.15 | 4897 | 0.114 | 22.3 |
Schefflera actinophylla | −8.09a | – | −4.57a | – | 3311 | 0.084 | 29.8 |
Extractable wax fraction (mg cm−2; weight percentage) and differential factors of both solute mobilities are specified.
Solute mobilities at 25 °C were calculated from Arrhenius graphs (Buchholz, 1998).
Comparison of solute mobilities (k*; ±95% CI) for bifenox within CM and MX of 13 plant species at 25 °C
Plant species | CM log k* | 95% CI | MX log k* | 95% CI | Factor | Wax (mg cm−2) | Wax (%) |
Populus canescens | −5.00 | 0.07 | – | – | – | 0.053 | 33.9 |
Pyrus pyrifolia | −5.34 | 0.17 | −3.29 | 0.05 | 112 | 0.055 | 25.5 |
P. communis cv. Conference | −5.43 | 0.11 | −3.74a | – | 49 | 0.061 | 23.7 |
Stephanotis floribunda | −5.68 | 0.25 | −3.73 | 0.02 | 89 | 0.026 | 10.2 |
M. domestica cv. Golden Delicious | −6.20 | 0.08 | −3.36 | 0.06 | 692 | 0.044 | 23.7 |
Ilex aquifolium | −6.64 | 0.18 | −4.14 | 0.12 | 316 | 0.162 | 24.6 |
Ginkgo biloba | −6.97 | 0.11 | −5.54 | 0.19 | 27 | 0.054 | 13.4 |
Melicoccus bijugatus | −7.04 | 0.11 | −4.81 | 0.06 | 170 | 0.049 | 8.8 |
Hedera helix | −7.16 | 0.14 | −3.75 | 0.05 | 2570 | 0.022 | 10.2 |
Strophanthus gratus | −7.18 | 0.16 | −5.34a | – | 69 | 0.060 | 19.5 |
Vanilla planifolia | −7.67 | 0.11 | −6.93 | 0.06 | 5 | 0.061 | 10.8 |
Ilex paraguariensis | −7.89 | 0.11 | −4.20 | 0.15 | 4897 | 0.114 | 22.3 |
Schefflera actinophylla | −8.09a | – | −4.57a | – | 3311 | 0.084 | 29.8 |
Plant species | CM log k* | 95% CI | MX log k* | 95% CI | Factor | Wax (mg cm−2) | Wax (%) |
Populus canescens | −5.00 | 0.07 | – | – | – | 0.053 | 33.9 |
Pyrus pyrifolia | −5.34 | 0.17 | −3.29 | 0.05 | 112 | 0.055 | 25.5 |
P. communis cv. Conference | −5.43 | 0.11 | −3.74a | – | 49 | 0.061 | 23.7 |
Stephanotis floribunda | −5.68 | 0.25 | −3.73 | 0.02 | 89 | 0.026 | 10.2 |
M. domestica cv. Golden Delicious | −6.20 | 0.08 | −3.36 | 0.06 | 692 | 0.044 | 23.7 |
Ilex aquifolium | −6.64 | 0.18 | −4.14 | 0.12 | 316 | 0.162 | 24.6 |
Ginkgo biloba | −6.97 | 0.11 | −5.54 | 0.19 | 27 | 0.054 | 13.4 |
Melicoccus bijugatus | −7.04 | 0.11 | −4.81 | 0.06 | 170 | 0.049 | 8.8 |
Hedera helix | −7.16 | 0.14 | −3.75 | 0.05 | 2570 | 0.022 | 10.2 |
Strophanthus gratus | −7.18 | 0.16 | −5.34a | – | 69 | 0.060 | 19.5 |
Vanilla planifolia | −7.67 | 0.11 | −6.93 | 0.06 | 5 | 0.061 | 10.8 |
Ilex paraguariensis | −7.89 | 0.11 | −4.20 | 0.15 | 4897 | 0.114 | 22.3 |
Schefflera actinophylla | −8.09a | – | −4.57a | – | 3311 | 0.084 | 29.8 |
Extractable wax fraction (mg cm−2; weight percentage) and differential factors of both solute mobilities are specified.
Solute mobilities at 25 °C were calculated from Arrhenius graphs (Buchholz, 1998).
Cuticular waxes and cutin matrix
Cuticular wax composition is very complex and varies according to species, ontogenetic development, and environmental conditions (Bianchi, 1995; von Wettstein-Knowles, 1995). The commonest components are n-alkanes and primary alcohols, both of which range from C16 to C36 (Holloway, 1994). Long alkyl chain compounds exhibit a solid-state polymorphism (liquid→hexagonally packed solid phase→orthorhombically packed solid phase) across a temperature scan (Watanabe, 1961). The physical state of the cuticular wax may contain several phases and crystalline forms and is likely, therefore, to be the critical feature that determines the transport properties of the cuticle. Highly ordered crystalline regions are inaccessible to permeation. The physical state of a mixture is a function of its chemical composition and of physical variables like temperature, pressure, etc. and is best described using a phase diagram. Phase diagrams for such complex mixtures as cuticular waxes are difficult to obtain and would not be very informative due to their complexity. The examination of a simple binary mixture of two chemically homologous waxes (1-tetradecanol and 1-octadecanol) revealed very complex mixing characteristics, and suggested frequent phase immiscibility (Carreto et al., 2002). Therefore, a mosaic of phase domains had to be expected even at ambient temperatures.
This coexistence of crystalline and fluid or amorphous phases was determined for the cuticular waxes extracted from several different plant species (Reynhardt and Riederer, 1991, 1994; Viougeas et al., 1995; Schreiber et al., 1997), and was also demonstrated in situ (Merk et al., 1998). The measurement of the diffusion characteristics in reconstituted waxes is thought to be a reasonable approach since the composition, and the proportion of crystallites and amorphous phases is not altered during recrystallization (Schreiber and Schönherr, 1993). A good correlation between mobilities measured in reconstituted cuticular waxes and in CMs was found, which confirmed viscosity as an important property (Schreiber and Riederer, 1996; Kirsch et al., 1997). These investigations revealed that the degree of crystallinity and the spatial arrangement of crystals determined the permeating path through the amorphous phase, which in turn determined the effectiveness of the diffusion barrier (Schreiber et al., 1997). Extracted waxes might, however, crystallize in a different shape (spatial extension) compared with those confined in a polymer matrix. Due to tortuosity (the path taken around the crystalline obstacles) the length of the diffusion path can be much greater than the thickness of the barrier (Schreiber et al., 1996a; Baur et al., 1999).
The study of synthetic chemical polymer membranes can also help to elucidate the mechanism of diffusion across CMs, even though they are, in general, thicker and less complex than plant cuticles. This enables a more detailed view of the functional relationships to be obtained. For instance, clay platelets are incorporated as inert fillers into polymers to improve their mechanical and barrier properties. To make the clay platelets compatible with hydrophobic materials, such as polyolefins and waxes, their surfaces are treated with monolayers of specific amines. The mechanism by which these organoclays improve the barrier properties of the film relies on the high aspect ratio (relationship between the width and height) of the exfoliated clay platelets to impart a tortuous diffusion path. The tortuosity factor can be as high as several-hundred-fold for impermeable platelets with aspect ratios of 100–500 and at modest mineral loadings of 5–10 vol% (Cussler et al., 1988).
The tortuosity of nanocomposite materials, however, is rarely that high (typically 2- to 4-fold) and it has been shown that the interphase surrounding the clay platelets exerts a profound influence on gas permeation (Chaiko and Leyva, 2005): The polymer systems, the polyolefins together with the waxes, are semi-crystalline materials consisting of crystalline and amorphous phases. If the hydrocarbon chains are long enough, they will fold in on themselves to form crystallites (10–20 nm). The crystallites are held together by amorphous chain segments that contribute to the elastic strength of the material. If the hydrocarbon chain length is shortened sufficiently, the amorphous chain segments become less able to bridge the gap between the crystallites, and the material becomes brittle. Gas diffusion can then take place at the interfaces between the crystallites.
Similar interactions were also demonstrated with Hordeum wax. Longer chains bridged the amorphous zone between two adjacent layers of crystalline material, thus linking the two layers (Reynhardt and Riederer, 1994). The relevance of tortuosity in cuticular waxes as an effective way of reducing the permeabilities of plant cuticles is discussed by Schreiber (2006).
A significant amount of shrinkage might accompany wax recrystallization due to the high degree of crystallinity and the large density difference between the amorphous melt and the crystal phase. As the freezing points of the individual components in the wax differ significantly (Chaiko and Leyva, 2005), this shrinkage could lead to extensive cracking. Nanocomposite materials mixed with polyethylene, however, displayed reduced embrittlement at high clay loadings, and the films were quite flexible and free of gross structural defects. Within plant cuticles the cutin polymer can be viewed as the analogous elastomer ensuring flexibility and avoiding embrittlement.
The plant cuticle has to be flexible to follow deformations caused either by fluctuations in turgor pressure or by wind stress. Petracek and Bukovac (1995) investigated the rheological properties of tomato fruit cuticles and identified the cuticle as a viscoelastic polymer, comprising both elastic (reversible) and plastic (irreversible) components. Furthermore, water affected the mechanical properties, and waxes reduced elasticity and susceptibility to fracturing. The mechanical properties of cuticles are not related to thickness but are likely to be altered by chemical composition (Wiedemann and Neinhuis, 1998). Marga et al. (2001) specified that the chemical composition determined both the biochemical properties as well as the elastic or rigid behaviour.
Consequently, several structural principles described for synthetic polymers can be applied to the plant cuticle. The plant cuticle can be described as a matrix composed of highly ordered crystalline and disordered amorphous regions, within which the reduced dynamics in the crystalline regions of the wax barrier make them practically inaccessible to permeants (Reynhardt and Riederer, 1991).
The cutin polymer not only serves as a matrix but also contributes to the transport characteristics of the cuticle (Santier and Chamel, 1998). Cutin monomers are cross-linked by ester, peroxide, and ether bridges (Kolattukudy, 1984), and the presence of large amounts of di- and tri-hydroxyfatty acids may affect the degree of cross-linking. It was demonstrated that the maturation of cutin led to the addition of covalent bonds which resulted in less polarity and reduced permeability (Schmidt et al., 1981). The wax-free matrix membranes (MXs) of several plant species showed a relatively wide range of solute mobilities (Table 1). The applicability of the UDOS method also provided evidence for the asymmetry of the cutin matrix. Chloroform–methanol extraction of CM to provide MX not only removes the impermeable wax crystallites but also the amorphous waxes. Therefore, the elevated solute mobilities in MX are due to the reduced viscosity (absence of amorphous waxes) and the shortened diffusion path (lack of crystalline waxes causing tortuosity).
Solute size effects
Size dependence of mobility was quantified by plotting for each plant species logk* versus molar volume (Vx) for the respective sets of model compounds. Characteristic molar volumes were calculated according to McGowan and Mellors (1986). These molar volumes represent the volumes at absolute zero temperature and they are closely correlated to van der Waals volumes (McGowan, 1969).
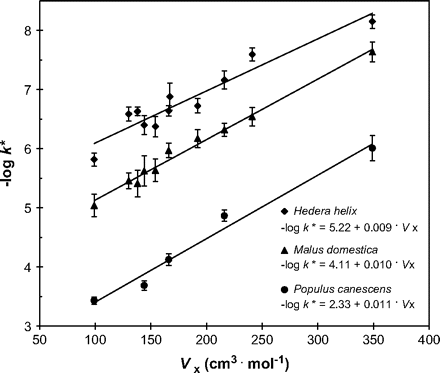
The effect of molar volumes (Vx) of model compounds on their mobility (k*) in cuticular membranes of three selected plant species. Averages of 16–20 cuticular membranes and 95% confidence intervals are shown.
No significant differences were observed in β′ among all the species tested, with the average value of β′ being 0.0095 mol cm−3 at 25 °C. This equates to a reduction in solute mobility of 700 times if Vx is increased from 100 to 400 cm3 mol−1. This size discrimination was found to be the same when cuticular waxes were extracted and comparable size selectivities were determined for polymer MXs (Baur et al., 1999). A similar correlation between diffusivity and solute size was also found for reconstituted waxes (Schreiber and Schönherr, 1993; Schreiber et al., 1996a; Schreiber, 2006).
Temperature
Size selectivity within plant cuticles is very temperature sensitive. For example, in ivy cuticles, the difference in solute mobilities between IAA (Vx=130 cm3 mol−1) and tebuconazole (Vx=241 cm3 mol−1) amounts to factors of 24.5, 10.5, and 2.2 at 25 °C, 35 °C, and 55 °C, respectively (Buchholz, 1998). Therefore, size selectivity diminishes at elevated temperatures and intensifies at low temperatures.
The benefit of this relationship for the estimation of the solute mobilities of new compounds at a given temperature and plant species has been impressively demonstrated (Buchholz et al., 1998). If the temperature sensitivity of a plant species is known for only two compounds (i.e. having two respective Arrhenius graphs) then a graph of β′ versus temperature can be made. With this information, the size selectivity (β′) for any desired temperature can be derived and consequently the solute mobility for any third compound (Vx) is predictable.
An explanation for this (temperature-dependent) size discrimination can be derived from the free volume theory (Coughlin et al., 1991; Duda and Zielinski, 1996). For a successful diffusion step within a polymer the penetrant needs a hole (a space between polymer chains) directly adjacent to its actual position. Enough energy to make the jump is ensured by the motion of the solute itself, via Brownian motion. Hence it follows that only the movement of the adjacent polymer chains limits the solute's spatial motion. At a given temperature, a polymer has a characteristic distribution of these temporary holes. Elevating the temperature causes an increase in the motion of the polymer chains which, in turn, produces a shift in the hole-size distribution towards bigger holes. (Increased energy enables the formation of larger gaps between adjacent polymer chains due to loosened bonds.) By contrast, the temporal abundance of small holes is relatively reduced at higher temperatures (Fig. 5).
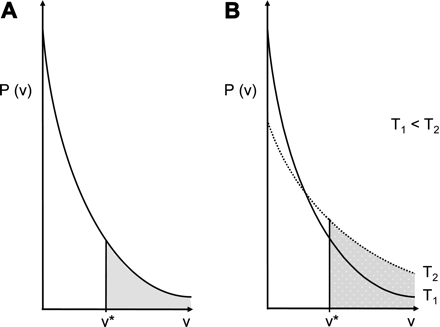
Exponential hole-size distribution of a polymer matrix as affected by temperature (modified according to Lieb and Stein, 1972). (A) The distribution function provides the probability (P) of finding a free-volume hole of a specific size (v*) at a given temperature (T). v* is the minimum volume hole size into which a molecule can jump. The diffusion coefficient is considered to be proportional to the probability of finding a hole of volume v* or larger. (B) Increasing temperature increases the overall free volume available for migration (shaded area).
The structural and functional integrity of cuticles can be examined by measuring mobility after temperature cycling. The comparison of subsequent experiments after annealing can identify hysteresis effects due to rearrangement of the wax constituents. Such experiments were conducted at temperatures up to 70 °C (Baur et al., 1997a). Results were plant-species specific and ranged from superimposed graphs (Prunus laurocerasus) to the straightening of previously curved graphs (Citrus aurantium), and in one case showed 10-fold increased mobilities at 25 °C (Hedera helix). These different results were related to differences in wax composition causing different melting behaviours and redistribution during annealing.
The sonication of CMs increased solute mobility at 25 °C by a factor of 4 and reduced the ED from123 kJ mol−1 to 92 kJ mol−1. Phase transitions or structural defects were not indicated by the Arrhenius graphs (Fig. 6). The interpretation of these results remains speculative (e.g. loosening of chemical bonds), although a further observation was that these effects diminished during storage (several months), and mobilities reverted to those of untreated CMs. This indicated a re-equilibration between the wax crystallites (‘healing of defects’) over time, as previously described for water transpiration (Geyer and Schönherr, 1990).
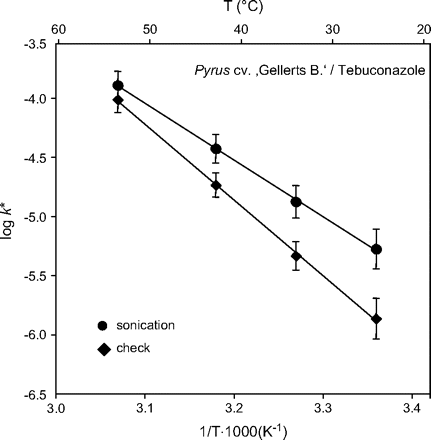
Arrhenius graphs obtained for tebuconazole in CM of Pyrus (cv. Gellerts Butterbirne). One sample of CMs (n=10) was treated with sonication for 5 min in a water bath 1 d before experimental use, whereas the other sample (n=11) remained untreated.
Based on the transition state theory (Glasstone et al., 1941) data can be plotted according to the thermodynamic relationship between the pre-exponential factor (k*0) of the Arrhenius equation (proportional to entropy) and activation energy (enthalpy) of diffusion (ED). A strict linear correlation was obtained for all of the CMs from 14 plant species and organic compounds having molar volumes ranging from 130 to 349 cm3 mol−1 and cuticle/water partition coefficients of 18–108 (Fig. 7). This provided evidence that all of these compounds diffused—irrespective of their size and lipophilicity—along the same lipophilic diffusion path. Each of the solutes must have travelled through a microenvironment with identical physicochemical properties (Buchholz and Schönherr, 2000).
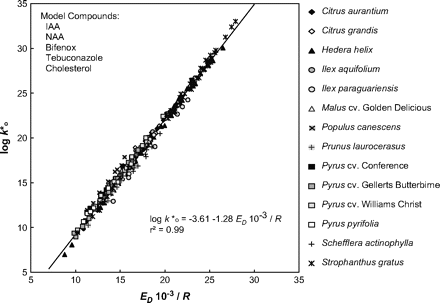
Correlation between the Arrhenius slopes (ED/R) and y-intercepts of Arrhenius graphs k*0. Data for individual CMs from 14 plant species were plotted. The solute mobilities were calculated for heating experiments with a temperature range of 25–50/55 °C for five different model compounds (modified from Buchholz and Schönherr, 2000, with kind permission of Springer Science and Business Media).
There are indications that water and lipophilic solutes can share the lipophilic path. This co-permeability was also confirmed by the simultaneous measurement of 3H-labelled water and 14C-labelled organic compounds across plant cuticles (Niederl et al., 1998). Water can, however, take two parallel paths of diffusion: the lipophilic path made of cutin and wax and the polar path composed of humidity-sensitive pores traversing the cuticles (Schreiber, 2002). Since wax extraction enhanced water permeability by several orders of magnitude (Schönherr, 1982), whereas the effect of humidity on cuticular water permeability was only 2–3-fold, it is reasonable to argue that the largest fraction of water diffused across the lipophilic paths in the cuticle (Schreiber et al., 2001; see also Table 2). The additional path predominated at temperatures higher than 30 °C and is likely to exhibit a threshold in size and/or lipophilicity (Schreiber, 2002).
Lipophilic pathway | Aqueous pathway | ||
Plant | Epicuticular waxes | (Retention on leaf surface) | |
No effect on permeability | Limit access to aqueous pores | ||
Intracuticular waxes | Amorphous waxes determine viscosity | Minor effect on penetration | |
Crystallites cause tortuosity and reduction in free volume | |||
Cutin matrix | Cross-linkage affects mobility | Quality and spatial arrangement of polar constituents determine abundance of aqueous pores | |
Substance (no plasticizer) | Molecular size | Pronounced size selectivity | Hydrate shells enforce weak size selectivity |
Lipophilicity | Affects driving force (sorption in waxes) | – | |
No effect on mobility | |||
Point of deliquescence | – | Low POD reduces risk of crystallized formulation residues | |
Concentration | Effect on driving force | Effect on driving force | |
Microclimate | Temperature | Effect on fluidity, viscosity of waxes and matrix | Minor effect on penetration |
Humidity | Effect on driving force (solubility) | Effect on driving force (solubility) and swelling of cuticle | |
Passive additives | Surfactants | (Retention; mass flow into open stomata) | |
Effect on driving force (solvation, partitioning) | Facilitation of access to aqueous pores | ||
Minor effect on penetration | |||
Humectants | Effect on driving force (partitioning, solvation, concentration dilution) | Effect on driving force (solubility, concentration dilution) | |
Prevention of crystallization extends time for penetration | Prevention of crystallization facilitates access to aqueous pores and extends time for penetration | ||
Active additives | Plasticizer | Penetrate into cuticle and decrease viscosity (accelerator) | Negligible effect on penetration |
Concentration dependent efficacy | |||
Plasticizer's and substance's mobility need a mutual fit |
Lipophilic pathway | Aqueous pathway | ||
Plant | Epicuticular waxes | (Retention on leaf surface) | |
No effect on permeability | Limit access to aqueous pores | ||
Intracuticular waxes | Amorphous waxes determine viscosity | Minor effect on penetration | |
Crystallites cause tortuosity and reduction in free volume | |||
Cutin matrix | Cross-linkage affects mobility | Quality and spatial arrangement of polar constituents determine abundance of aqueous pores | |
Substance (no plasticizer) | Molecular size | Pronounced size selectivity | Hydrate shells enforce weak size selectivity |
Lipophilicity | Affects driving force (sorption in waxes) | – | |
No effect on mobility | |||
Point of deliquescence | – | Low POD reduces risk of crystallized formulation residues | |
Concentration | Effect on driving force | Effect on driving force | |
Microclimate | Temperature | Effect on fluidity, viscosity of waxes and matrix | Minor effect on penetration |
Humidity | Effect on driving force (solubility) | Effect on driving force (solubility) and swelling of cuticle | |
Passive additives | Surfactants | (Retention; mass flow into open stomata) | |
Effect on driving force (solvation, partitioning) | Facilitation of access to aqueous pores | ||
Minor effect on penetration | |||
Humectants | Effect on driving force (partitioning, solvation, concentration dilution) | Effect on driving force (solubility, concentration dilution) | |
Prevention of crystallization extends time for penetration | Prevention of crystallization facilitates access to aqueous pores and extends time for penetration | ||
Active additives | Plasticizer | Penetrate into cuticle and decrease viscosity (accelerator) | Negligible effect on penetration |
Concentration dependent efficacy | |||
Plasticizer's and substance's mobility need a mutual fit |
Lipophilic pathway | Aqueous pathway | ||
Plant | Epicuticular waxes | (Retention on leaf surface) | |
No effect on permeability | Limit access to aqueous pores | ||
Intracuticular waxes | Amorphous waxes determine viscosity | Minor effect on penetration | |
Crystallites cause tortuosity and reduction in free volume | |||
Cutin matrix | Cross-linkage affects mobility | Quality and spatial arrangement of polar constituents determine abundance of aqueous pores | |
Substance (no plasticizer) | Molecular size | Pronounced size selectivity | Hydrate shells enforce weak size selectivity |
Lipophilicity | Affects driving force (sorption in waxes) | – | |
No effect on mobility | |||
Point of deliquescence | – | Low POD reduces risk of crystallized formulation residues | |
Concentration | Effect on driving force | Effect on driving force | |
Microclimate | Temperature | Effect on fluidity, viscosity of waxes and matrix | Minor effect on penetration |
Humidity | Effect on driving force (solubility) | Effect on driving force (solubility) and swelling of cuticle | |
Passive additives | Surfactants | (Retention; mass flow into open stomata) | |
Effect on driving force (solvation, partitioning) | Facilitation of access to aqueous pores | ||
Minor effect on penetration | |||
Humectants | Effect on driving force (partitioning, solvation, concentration dilution) | Effect on driving force (solubility, concentration dilution) | |
Prevention of crystallization extends time for penetration | Prevention of crystallization facilitates access to aqueous pores and extends time for penetration | ||
Active additives | Plasticizer | Penetrate into cuticle and decrease viscosity (accelerator) | Negligible effect on penetration |
Concentration dependent efficacy | |||
Plasticizer's and substance's mobility need a mutual fit |
Lipophilic pathway | Aqueous pathway | ||
Plant | Epicuticular waxes | (Retention on leaf surface) | |
No effect on permeability | Limit access to aqueous pores | ||
Intracuticular waxes | Amorphous waxes determine viscosity | Minor effect on penetration | |
Crystallites cause tortuosity and reduction in free volume | |||
Cutin matrix | Cross-linkage affects mobility | Quality and spatial arrangement of polar constituents determine abundance of aqueous pores | |
Substance (no plasticizer) | Molecular size | Pronounced size selectivity | Hydrate shells enforce weak size selectivity |
Lipophilicity | Affects driving force (sorption in waxes) | – | |
No effect on mobility | |||
Point of deliquescence | – | Low POD reduces risk of crystallized formulation residues | |
Concentration | Effect on driving force | Effect on driving force | |
Microclimate | Temperature | Effect on fluidity, viscosity of waxes and matrix | Minor effect on penetration |
Humidity | Effect on driving force (solubility) | Effect on driving force (solubility) and swelling of cuticle | |
Passive additives | Surfactants | (Retention; mass flow into open stomata) | |
Effect on driving force (solvation, partitioning) | Facilitation of access to aqueous pores | ||
Minor effect on penetration | |||
Humectants | Effect on driving force (partitioning, solvation, concentration dilution) | Effect on driving force (solubility, concentration dilution) | |
Prevention of crystallization extends time for penetration | Prevention of crystallization facilitates access to aqueous pores and extends time for penetration | ||
Active additives | Plasticizer | Penetrate into cuticle and decrease viscosity (accelerator) | Negligible effect on penetration |
Concentration dependent efficacy | |||
Plasticizer's and substance's mobility need a mutual fit |
Arrhenius graphs obtained by repeat measurements on the same CM showed that, although the data points remained on the general line for CMs, they were shifted towards lower entropy and enthalpy values (Fig. 8). A consecutive third run rarely caused any further change in this free energy relationship. Extraction of the cuticular waxes from the CMs greatly reduced the activation energies and increased the solute mobilities. In the thermodynamic plot the linear dependency was maintained, which means that the free energy was identical in both membrane types, CM and MX. Additionally, the graphs obtained for MX exhibited a parallel displacement towards higher entropy. This displacement was interpreted as a temperature-independent tortuosity factor directly related to entropy (Buchholz and Schönherr, 2000). Consecutive heating cycles caused a shift along the line of a given membrane type towards lower entropy and enthalpy. This means that the individual chemical components might have been redistributed during the annealing phase but this did not affect the physicochemical properties of the diffusion path.
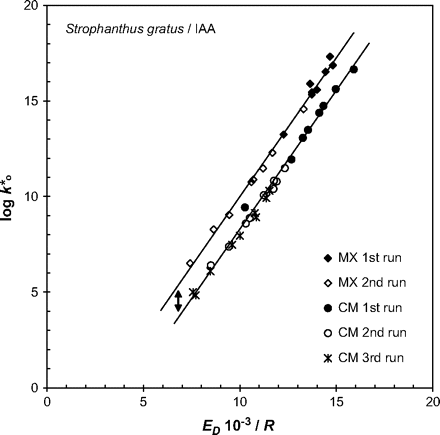
Correlation between Arrhenius slopes (ED/R) and y-intercepts of Arrhenius graphs k*0. Data for individual cuticular membranes (CM) and polymer matrix membranes (MX) from Strophanthus gratus leaves are plotted. The double arrow indicates the parallel displacement of both lines with identical slope. This displacement amounted to 1.7 (log scale). Solute mobilities were calculated for two or three successive heating experiments with identical membranes at temperature ranges of 25–55 °C (CM) and 15–30 °C (MX). Between two temperature scans the membranes were kept at ambient temperature for 24 h.
Plasticizer effects
Foliar uptake can be affected by formulation and adjuvants (Falk, 1994; Baur, 1998). Formulants are any added material in a pesticide formulation other than the biologically active ingredient(s), whereas adjuvants are those formulants designed to enhance the activity or other properties of an agrichemical product (Holland, 1996). Surfactants that enhance foliar uptake by increasing the mobility of a given compound are called accelerating adjuvants (Schönherr, 1993b). This accelerator effect has been demonstrated for various surfactants which were also plasticizers (Schönherr, 1993a; Schönherr and Baur, 1996; Baur et al., 1997b; Schönherr et al., 2001; Shi et al., 2005), and also for active ingredients (Baur et al., 1996a).
This increased diffusivity is dependent on concentration and is interpreted as an unspecific plasticizing effect of the surfactant molecule on the ultrastructure of the wax (Schreiber et al., 1996b). Rapid induction and complete reversibility of the effects indicate that the physical wax structure is not irreversibly altered (e.g. solubilization of wax or destruction of crystalline wax domains) by the surfactant-induced acceleration (Schreiber, 1995, 2006).
The plasticizing effect of accelerators on cuticular waxes in situ (CM) and on the cutin matrix (MX) was investigated by applying the entropy–enthalpy relationship (Buchholz and Schönherr, 2000). The plasticizer tributyl phosphate (TBP) increased solute mobilities and reduced ED significantly for both CM and MX. This indicated that sorption of TBP increased segmental mobility of polyethylene chains in both cutin and wax. This effect was almost completely reversible, and for both plasticized membrane types (CM, MX) the thermodynamic relationship existed whereby all data points lay on the same line. These data were used to characterize the lipophilic pathway across plant cuticles in terms of the free-volume theory, giving evidence that both high temperature and accelerators act similarly on the barrier properties of a CM.
It is known that the efficacy of an accelerator is dependent on both the type of plasticizer and on its concentration. Arrhenius diagrams indicate that lower activation energies are produced with higher loadings of plasticizers (Schönherr et al., 2001). The different intrinsic efficacies of two plasticizers have been elucidated using this method. This also demonstrated that the penetrating compound and the surfactant must be present simultaneously in the limiting skin in order to enhance the compound's mobility.
Perkins et al. (2005) demonstrated the plasticizing effect on both reconstituted waxes and on the native leaf surface. Localized thermal analysis measurements revealed considerable variation in the wax melting point (Tm) over the leaf surface, indicating lateral heterogeneity in wax composition. The authors proposed areas of amorphous wax formations of at least 1 μm2. After droplet application of Synperonic A7 (ethoxylated alcohol) they observed a depression in the wax melting point, mainly at the deposit annulus, whereas the surfactant Synperonic A20 (with a more homogenous deposit structure) had no observable effect on the Tm of the wax.
According to the model presented to describe the foliar uptake of non-electrolytes (Schönherr et al., 1999), these two surfactants could be classified as active (accelerator; plasticizing) or passive (donor; dissolving) adjuvants (Cronfeld et al., 2001). Passive adjuvants, which remain on the surface of the cuticles, can serve as solvents, and they affect driving forces via their effects on Kwxfr (partitioning of the active substance or adjuvants between the formulation residue and the cuticular waxes) and Cfr (concentration of the active substance in the formulation residue). If these passive adjuvants are hygroscopic, their effect on driving forces depends greatly on humidity. Active adjuvants are solvents as well, but in addition they penetrate into cuticles and increase solute mobility. These effects depend on the type of accelerator, temperature, size of solute, and plant species. Accelerators decrease the viscosity of amorphous waxes which results in reduced size selectivity. Accelerators also reduce differences in solute mobility among plant species (see above).
Foliar uptake
The sorption process is especially affected by numerous factors which change during droplet drying (or rewetting) and can rarely be analysed individually. Figure 9 attempts to illustrate the most relevant factors. Physicochemical properties of the active substance (group 1) affect the ‘external’ term of the driving force: KwxfrCfr. For example, low water solubility causes a low concentration of dissolved molecules (Cfr), and will result in minor sorption in cuticular wax (Kwxfr), although partitioning could be high. But a spray solution comprises, beside the active substance, adjuvants and other formulants, like emulsifiers, buffer, etc. (group 2) which affect, for example, solvation and partitioning (Baur et al., 1997c). Further, environmental factors like temperature and humidity govern the velocity of these dynamic processes. Consequently, it has to be realized that, in order to maximize rates of penetration, the product of Kwxfr and Cfr has to be increased, whereas both quantities are mutually dependent on each other.
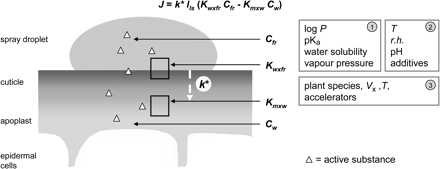
Schematic drawing of a spray droplet and the underlying plant epidermis. Factors affecting cuticular penetration via the ‘lipophilic pathway’ are grouped into (1) physicochemical properties of the active substance, (2) conditions determined by the environment and the spray solution, and (3) parameters affecting solute mobility, k*. J, Rate of cuticular penetration; lls, length of the diffusion path in the limiting skin (≠ thickness); Kwxfr, partition coefficient wax/formulation residue; Kmxw, partition coefficient polymer matrix/water; Cfr, concentration of active substance in formulation residue; Cw, concentration of active substance in water (apoplast) (according to the model described by Schönherr et al., 1999).
The individual parameters affecting solute mobility (group 3) were extensively described above. After travelling through the cuticle the ‘internal’ term of the driving force, KmxwCw becomes relevant. Here, the partitioning in the aqueous phase of the apoplast and potential access to the symplast (after traversing the biomembrane) determine the translocation into plant tissues. Rapid distribution will result in a low concentration underneath the spray droplet and enable large driving forces.
Finally, all those parameters describing the lipophilic pathway of non-electrolytes across plant cuticles—and demarcating it from the aqueous pathway (Schönherr, 2006)—are summarized in Table 2.
Conclusions
It is the big advantage of the UDOS method that, with this desorption measurement and the determination of the solute mobility k*, a pure descriptor of the diffusion process in CMs can be obtained. Only the feasibility of determining sorption-independent mobilities in CMs (or diffusion coefficients in extracted wax; Schreiber, 2006) allowed the quantification and interpretation of size selectivity and tortuosity, as well as entropy/enthalpy relationships. Therefore, the properties of the lipophilic pathway are now completely characterized. Only the interpretation of the plant species-specific y-intercept of a hypothetical molecule having zero molar volume, k*0, and its reference to tortuosity, remain to be explained in more detail.
This knowledge on factors affecting solute mobility (group 3 in Fig. 9) and the physicochemical properties of a given non-polar active substance (group 1 in Fig. 9) are the starting points for tailored crop protection products.
Comments