-
PDF
- Split View
-
Views
-
Cite
Cite
Hervé Cochard, Sébastien Forestier, Thierry Améglio, A new validation of the Scholander pressure chamber technique based on stem diameter variations, Journal of Experimental Botany, Volume 52, Issue 359, 1 June 2001, Pages 1361–1365, https://doi.org/10.1093/jxb/52.359.1361
- Share Icon Share
Abstract
The Scholander pressure chamber is one of the most widely used techniques for measuring plant water status. However, the technique has been the subject of recent controversies, and its validity awaits new experimental evidence. This paper presents a new test based on the analysis of the dependence on water potential difference (ΔΨ) of stem diameter variation (Δ D ) in walnut ( Juglans regia L.). The correlation between ΔΨ and Δ D was established (1) on transpiring potted trees, (2) on dehydrating cut branches, (3) by perfusing the xylem of branch segments with mannitol and sucrose solutions, and (4) by pressurizing segments in a pressure sleeve. The ΔΨ was respectively assessed with a pressure chamber (1, 2), a freezing point osmometer (3) and an air pressure transducer (4). A single relationship was established between ΔΨ (ranging from 0 to −2 MPa) and Δ D for all the experiments. This shows that the measured changes of water potential were correlated to similar modifications of water content in the stems, irrespective of the technique used to induce these changes, and therefore validates the pressure chamber technique and confirms the occurrence of large negative pressures in the xylem of walnut branches.
Introduction
Ever since the early stages or modern plant physiology in the 19th century, the mechanism of water ascent has been the subject of much controversy. The current understanding of the mechanism comes from very early experiments ( Böhm, 1893 ; Dixon and Joly, 1894 ) and according to their ‘cohesion‐tension’ theory, the transpiration pull induces negative pressures in the leaves which are transmitted to the roots via the cohesion of water in the xylem conduits. The theory predicts the occurrence of large negative pressures in the xylem ( Px ). Dixon and Joly were aware that a measure of Px would validate their theory but their early efforts were stopped by technical problems ( Dixon and Joly, 1894 ). The development of thermocouple psychrometers ( Spanner, 1951 ) and of the pressure chamber technique ( Scholander et al ., 1964 ) permitted indirect estimates of the xylem pressure on a large number of species, which gave considerable credit to the cohesion‐tension theory. Because of its feasibility and its simplicity, the pressure chamber technique is widely used by plant physiologists, but also by farmers to measure plant water stress and schedule irrigation. For many species, Px , as determined with these techniques, typically ranges between −1 and −2 MPa.
During the past decade, new techniques have been introduced to assess Px directly. First, tiny glass capillaries (‘xylem pressure probe’, Zimmermann et al ., 1994 ) have been inserted in situ into vessel lumens, and direct readings of Px have been attempted. The pressures that were recorded with this technique were much less negative (in the range of 0 to −0.5 MPa) than the values produced by the pressure chamber ( Zimmermann et al ., 1994 ). However, new experiments have recently been conducted with the pressure probe ( Wei et al ., 1999 ) and were found to agree with the pressure chamber. Second, the vessel content during plant transpiration has been observed with a cryo‐scanning electron microscope ( Canny, 1997 ). These observations have revealed the presence of many air bubbles in the vessel lumens or of water droplets lying against the vessel wall. These observations have been used as arguments against the occurrence of large negative pressures in the vessels because the capillary pressure calculated with the curvature radius of these bubbles is only of a few kPa. However, in a recent study, Cochard et al ., 2000 , 2001 ) it was suggested that these bubbles were artefacts of the freezing procedure.

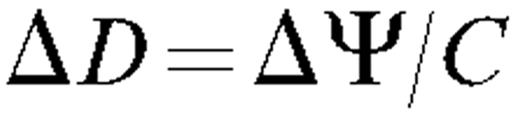
The principle of this test is to correlate changes in stem diameter with changes in ΔΨ, ΔΨ being measured with the pressure chamber and independent techniques. The dependence of Δ D with Px (assessed with the pressure chamber) was first extablished by measuring their diurnal variations on both potted trees and on cut branches dehydrated on a bench top. Branch segments were then perfused with solutions having different osmotic pressures in order to provoke changes in Π x . Finally, a pneumatic pressure was applied to the bark of branch segments to alter Pb . If the dependence of Δ D to ΔΨ is the same in these experiments, the conclusion would be that the pressure change measured with the pressure chamber yielded a correct estimation of Px .
Materials and methods
Plant material
Experiments were conducted during the 1999 growing season on branches of 18‐year‐old Juglans regia L. cv. Lara trees growing in an orchard at the INRA site at Crouelle (Clermont‐Ferrand, France). All the diameter measurements were performed on previous year (1998) shoot segments with 10–15 mm diameter. For the branch dehydration experiments, 1 m long leafy branches were collected and brought to the laboratory in a black plastic bag to minimize leaf dehydration. For the other experiments, only the 1998 shoot segment was collected in the field.
Branch dehydration
The cut end of the branches collected on the trees were recut under tap water in the laboratory and allowed to rehydrate for 2 h while still enclosed in a plastic bag. During that period, two LVDT linear displacement transducers (model DF2.5, Solartron Metrology, Massy, France) were mounted on 1998 shoot internodes with Invar‐made holders and connected to a data logger. The transducers recorded the changes in stem diameter (accuracy<1 μm). Once stem diameters had stabilized, the leaf water potential (Ψ l ) was measured on two or three terminal leaflets with a pressure chamber. Preliminary experiments have shown that Ψ l measured on leaflets enclosed in a small air‐tight plastic bag several hours before excision were similar to the Ψ l value of unbagged leaflets. This confirmed that the branches placed in the large plastic bags were not transpiring and that, under these conditions, Ψ l was a measure of the branch xylem water potential (Ψ x ) ( Turner, 1981 ). The branch cut end was then removed from the water supply and the bag was opened for several hours to allow leaf transpiration. The bag was closed anew and, once the diameters stabilized, Ψ l was measured as above. The whole procedure was repeated until Ψ l reached c . −2 MPa. The experiment was repeated on seven different branches. In order to detect any temperature‐ or time‐dependent change in stem diameter, three branches were treated as before but left entirely enclosed in a plastic bag during the whole experiment. Overall, the changes in stem diameter remained very small for these samples (less than 10 μm).
Stem perfusion
The objective of this experiment was to perfuse the xylem of short leafless shoot segments with solutions at different osmotic potentials and follow the changes in diameters. Eleven centimetre long shoot segments were obtained from internodes of previous year shoots, placed vertically and fitted to plastic tubing. On the end where the tubing was connected, the bark was removed over a 1 cm length, in order to expose only the xylem to the perfused solution. The tubing was filled with the solution and connected to a reservoir containing the same solution. The pressure head was about 30 cm. One LVDT transducer (model DF 2.5) was mounted on each segment as above. The different solutions were obtained by diluting the appropriate quantity of d ‐sucrose and d ‐mannitol with distilled water in order to obtain osmotic potentials (Π x ) of 0.0, −0.5, −1.0, −1.5, and −2.0 MPa. The Π value of each solution was verified at the beginning of each experiment with a freezing‐point osmometer (Model 13DR, Roebling, Berlin, Germany). The samples were first perfused with distilled water for 2 h. When the diameter readings stabilized, the tubing was emptied and the samples were perfused with the −0.5 MPa solutions, until the diameters stabilized. The same procedure was successively repeated with the –1, –1.5 and –2 MPa solutions. The diameters stabilized after 10–20 h. The Π value of the solution dripping out of the segments was measured periodically with the osmometer. A few minutes after the reservoir solution was changed, the dripping solution was at the same Π value as the solution in the reservoir. Twelve and three segments were perfused with the different mannitol and sucrose solutions, respectively. As controls, two segments were continuously perfused with distilled water while the other segments were perfused with mannitol or sucrose. The diameter variations were barely detectable on these samples.
Stem pressurization
The aim of this experiment was to expose a shoot segment to a pneumatic pressure and provoke a stem shrinkage. Fifty centimetre long shoot segments were obtained from previous year shoots and inserted in a pressure sleeve. The steel sleeve had a ‘T’ form, the shoot being placed, with the two ends protruding about 15 cm in the horizontal axis, and the vertical axis containing a pressure‐proof LVDT sensor (model SDF 15, Solartron, Massy, France). The LVDT sensor was fixed on the chamber with only the sensor axial rod touching the sample. Hence, the sensor was recording the change in stem radius, so the values were multiplied by two for consistency with the other experiments. A plastic tube was attached to one end of the stem in order to supply the sample with distilled water continuously during the experiment. This was necessary to make sure that the xylem water potential remained close to 0 MPa throughout the experiment ( Cochard et al ., 1992 ). The chamber was connected to a tank of nitrogen and pressure in the chamber was recorded and automatically controlled. The pressure was increased from 0 to 2 MPa in steps of 0.25 MPa and maintained for 5 h at each selected pressure. A data logger (model 21X, Campbell Scientific LTD, Logan, Utah, USA) regulated the pressure and collected the data. The experiment was repeated on five different branches.
Field measurements
The diurnal variations of Px and stem diameter were replotted from Améglio and Archer ( Améglio and Archer, 1996 ). LVDT sensors (model DF 2.5) were mounted on the previous year shoot segment of 3‐year‐old walnut trees grown in 200 l containers at the INRA site at Crouelle. The diurnal changes in Px were estimated as above by measuring the balancing pressure of leaves enclosed for at least 2 h in a plastic bag.
Water retention curves
In order to assess how stem shrinkage relates to an outflow of water from the bark to the xylem, the relationship between diameter and weight variations was analysed on 10 cm long internodes. Each segment was first rehydrated in tap water. The sample was then briefly dried with absorbing paper, its size and fresh weight measured, and an LVDT sensor (model DF 2.5) was installed. The segment was placed on the scale of a digital balance (resolution 0.01 g, model PM6100, Mettler) and allowed to dehydrate under laboratory conditions. Diameter variations were converted to volume changes assuming the samples were cylindrical. The experiment was repeated on nine different samples.
Results and discussion
For walnut shoot segments, changes in diameter reflected changes in water content (Fig. 1 ). A near 1:1 relationship was found between the mass and volume variations during the first stage of the dehydration, suggesting that most of the water lost by dehydration was originating from the elastic tissues located in the bark. When the diameter change was higher than 400 μm, the mass change was higher than the volume change, suggesting that water was lost by non‐elastic tissues. Water was probably released by cavitations in the xylem conduits because the xylem water potential was then close to the threshold potential for cavitation ( Tyree et al ., 1993 ; and see below).
In the following discussion, the results of the different experiments will first be analysed according to equation (1) . When samples were perfused with sucrose and mannitol solutions, sudden changes were provoked in Π x . Because the xylem conduits were exposed to a low and constant hydrostatic pressure, Px always remained close to zero in this experiment. The time‐course of D following a perfusion with mannitol was negatively exponential (Fig. 2 ). By contrast, the time‐course of D following a perfusion with sucrose was negatively exponential only during the first hours, D tending to increase later on (Fig. 2 ). The difference between both solutes was probably due to differences in the rate of active uptake into the bark tissue. The diameter increase noticed with sucrose suggests an accumulation of this solute in the bark tissue causing a flow of water back into the bark. The lack of diameter increase with mannitol suggests a much lower membrane permeability for this solute ( Steudle and Tyerman, 1983 ; Steudle et al ., 1987 ). This is also consistent with the fact that plasma membrane symports have been identified for sucrose ( Sauter, 1981 ) in contrast to mannitol.
When samples were pressurized in a pressure sleeve, Px remained close to zero, for the same reason as above, and Pb was rapidly increased. The mechanical force resulting from the pneumatic pressure applied to the bark could have, in theory, compressed non‐living tissues. The good agreement between the different experiments suggests that these tissue were not compressed for two non‐exclusive reasons: (1) their coefficient of elasticity was very high or (2) they were highly permeable to the gas and therefore the pneumatic gradient was small within the tissue. A non‐woody species may have behaved differently.
In the perfusion and pressurization experiments, the water potential differences were induced by long established physico‐chemical processes and quantified by unquestionable techniques. In the dehydration experiments, Px was altered as a result of branch dehydration and assessed indirectly by the pressure chamber. The measure is indirect because it is performed on non‐transpiring leaves assuming that the balancing pressure obtained on these leaves equals Px . For branches dehydrated in the laboratory Π x was close to 0 MPa because the stems were first rehydrated with distilled water. However, under field conditions, Π x values were probably slightly negative, but the effect on ΔΨ will depend on the σ values for the solutes in the sap.
In Fig. 3 the relationships between Δ D and ΔΨ is shown for all the experiments. The variability between samples was high, probably because of difference in capacitance (see equation 2 ). However, the variance was comparable when Δ D was normalized by D . On average, the response curves were all very similar and the linear regressions through the data were not statistically different. This shows that the measured changes of ΔΨ were correlated to similar modifications of water content in the stems, irrespective of the technique used to induce these changes. The effective water potential difference imposed by a solution is dependent on the solute reflection coefficient σ (see equation 1 ). In Fig. 3 , σ was hypothetically set to 1. The close agreement between the results of the different experiments in this study suggests that the hypothesis was realistic, which is in agreement with previously published values of σ for sucrose and mannitol ( Steudle and Tyerman, 1983 ; Steudle et al ., 1987 ).
According to equations (1) and (2) , the same causes have the same effects, it can be concluded that the estimate of ΔΨ with the pressure chamber is in perfect agreement with the other independent techniques. These experiments provide a new validation of Scholander's technique and also confirm that large xylem tensions develop in the xylem conduits in field‐grown trees (as low as –1.6 MPa in this study), in agreement with the prediction of the cohesion‐tension theory.
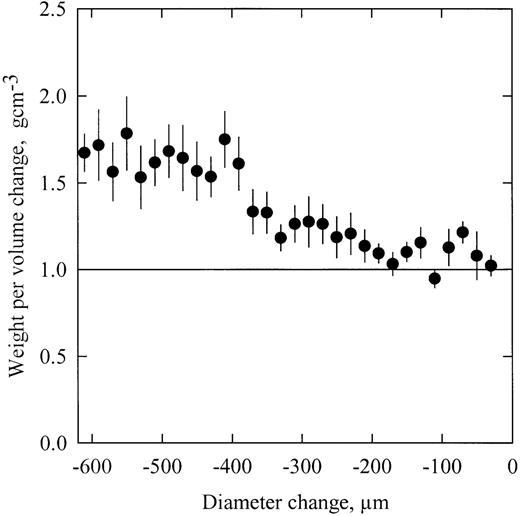
Weight loss per volume change of dehydrating shoot segments. Shoot dehydration provoked a stem diameter variation ( x ‐axis) caused by a shrinkage of the elastic tissues located in the bark. The weight per volume change ( y ‐axis) is an indicator of the outflow of water from the bark. When the ratio is close to unity, mostly bark tissues are losing water. When the ratio is higher than unity, water is also lost from the non‐elastic xylem tissue. The error bars indicate ±1 SE.
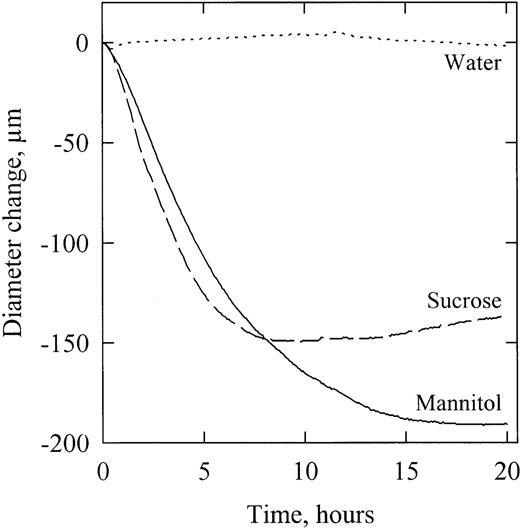
Time‐course of diameter variation of branch segments perfused with mannitol (plain line), sucrose (dashed line) and water (dotted line). For this representative example, solutions having a Π value of −1.5 MPa were perfused (at time=0) through different segments previously equilibrated with a solution at −1 MPa.
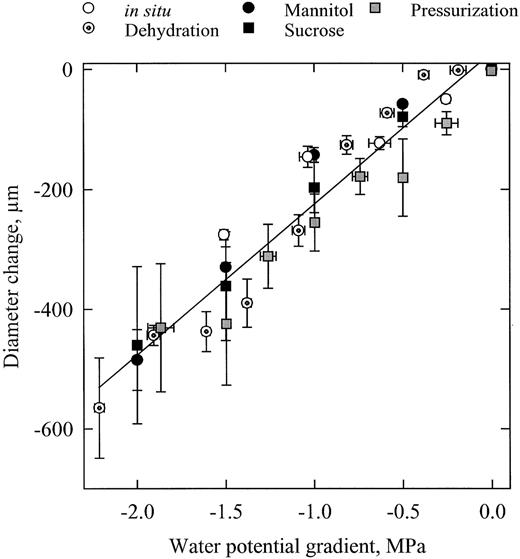
Dependence of stem diameter variation ( y ‐axis) on water potential difference ( x ‐axis) between xylem and bark tissues. Diameter variations were measured on transpiring potted trees (open circles), on dehydrating cut branches (crossed circles), by perfusing branch segments with concentrated solutions of mannitol (closed circles) and sucrose (closed squares) and by pressurizing segments in a pressure sleeve (gray squares). The error bars indicate ±1 SE and the line is a linear regression through all the data.
To whom correspondence should be addressed. Fax: +33 4 73 72 44 54. E‐mail: [email protected]
The authors thank C Bodet, P Chaleil, M Martignac, and S Ploquin for technical assistance during this work. This work was supported by the European union under Project FAIR1‐CT95‐0030 ‘Biosensors for tree irrigation’.
References
Améglio T, Archer P.
Böhm J.
Canny MJ.
Cochard H, Bodet C, Améglio T, Cruiziat P.
Cochard H, Améglio T, Cruiziat P.
Cochard H, Cruiziat P, Tyree MT.
Holbrook NM, Burns MJ, Field CB.
Passioura JB, Munns R.
Sauter JJ.
Scholander PF, Hammel HT, Hemmingsen EA, Bradstreet ED.
Simonneau T, Habib R, Goutouly GP, Huget JG.
Spanner DC.
Steudle E, Tyerman SD.
Steudle E, Oren R, Schulze ED.
Tyree MT, Cochard H, Cruiziat P, Sinclair B, Ameglio T.
Turner NC.
Wei C, Tyree MT, Steudle E.
Comments