-
PDF
- Split View
-
Views
-
Cite
Cite
Caitlin W Elgarten, Elisa B Margolis, Matthew S Kelly, The Microbiome and Pediatric Transplantation, Journal of the Pediatric Infectious Diseases Society, Volume 13, Issue Supplement_1, February 2024, Pages S80–S89, https://doi.org/10.1093/jpids/piad062
- Share Icon Share
Abstract
The microbial communities that inhabit our bodies have been increasingly linked to host physiology and pathophysiology. This microbiome, through its role in colonization resistance, influences the risk of infections after transplantation, including those caused by multidrug-resistant organisms. In addition, through both direct interactions with the host immune system and via the production of metabolites that impact local and systemic immunity, the microbiome plays an important role in the establishment of immune tolerance after transplantation, and conversely, in the development of graft-versus-host disease and graft rejection. This review offers a comprehensive overview of the evidence for the role of the microbiome in hematopoietic cell and solid organ transplant complications, drivers of microbiome shift during transplantation, and the potential of microbiome-based therapies to improve pediatric transplantation outcomes.
INTRODUCTION
Our bodies are colonized by trillions of microorganisms that play vital roles in maintaining our health. These microbes mainly reside in the gastrointestinal tract (the “gut microbiome”), but they also colonize our aerodigestive tract, skin, and genitourinary tract [1–3]. At no point during the lifespan do these microbial communities change as dramatically as during early childhood. During birth, infants acquire an enormous number of microbes, mostly from their mother’s vaginal, fecal, or skin microbiota [4]. Over the ensuing days and months, the composition of these microbial communities undergoes substantial shifts due to changes in the local microenvironment and microbial competition. For instance, the newborn gut is an aerobic environment comprised primarily of facultative aerobes such as Escherichia coli and Enterococcus species; however, this gut microbiome transitions to anaerobic bacteria (eg, Clostridium species) as oxygen levels decrease over the first several days of life [5, 6]. Thereafter, Bifidobacterium species predominate, particularly in exclusively breastfed infants. As solid foods are introduced, there is a shift toward Bacteroides, Clostridium, and Ruminococcus species such that, by 2–3 years of age, the gut microbiota resembles that of adults [6]. Notably, these shifts in microbiome composition during early childhood occur alongside maturation of the immune system and other crucial developmental processes.
Next-generation sequencing methods enable the identification of microbes in a culture-independent fashion, thus permitting description of the complete genomic content of microbiomes, and have the capacity to sequence many samples in parallel, overcoming a major limitation of prior methods. The development of these technologies vastly improved our ability to study microbial communities, with several concepts emerging that are important to understanding the impact of this microbiome on the outcomes of pediatric transplant recipients. First, commensal microbes that inhabit our bodies protect against potential pathogens. This concept, referred to as “colonization resistance,” occurs through a variety of mechanisms including competition for attachment sites, space, and nutrients; production of antimicrobial substances; modulation of host immune responses; and destruction of competitor niches [7–11]. The ability of microbial communities to provide colonization resistance exists on a spectrum (Figure 1). Microbiomes with high diversity (more species) and that are resilient to external perturbations generally provide more effective colonization resistance, while microbiomes of low diversity and stability offer less colonization resistance and tend to be associated with an increased risk of infection. Second, the microbiota has bidirectional, dynamic, and context–dependent interactions with the host immune system. The microbiota interacts with T cells through antigen-specific receptors and various other immune cell populations through Toll-like and Nod-like receptors [12–15], and these interactions serve to promote tolerance to commensal microbes and the mounting of effective immune responses to pathogens. Such microbiome–host crosstalk is particularly important for pediatric hematopoietic cell transplant (HCT) and solid organ transplant (SOT) recipients in whom immune tolerance is vital for graft survival and the avoidance of graft-versus-host disease (GVHD) and graft rejection. Several factors contribute to disruptions of the microbiome among transplant recipients, including exposure to broad-spectrum antibiotics [16–18], dietary changes, chemotherapy-induced damage to the gut epithelium [19], and changes in the number and function of immune cells as a result of treatment with immunosuppressive medications (Figure 2).
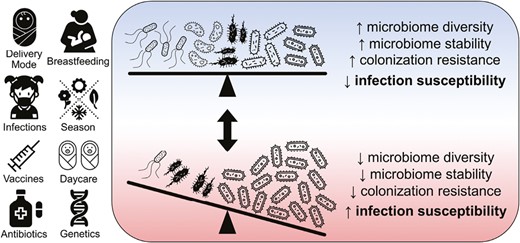
Host factors and exposures that influence microbiome colonization resistance and host infection susceptibility. Two microbial communities are shown representing opposite ends of the spectrum of microbiome diversity, stability, colonization resistance, and host susceptibility to infection. Compared with the microbiome shown at the bottom of the figure, the microbiome at the top of the figure has higher diversity in that it contains more species and these species have similar abundances; such a state is also generally associated with higher stability (more resiliency to external perturbations), the ability to more effectively resist colonization by potential pathogens, and lower susceptibility to infections.
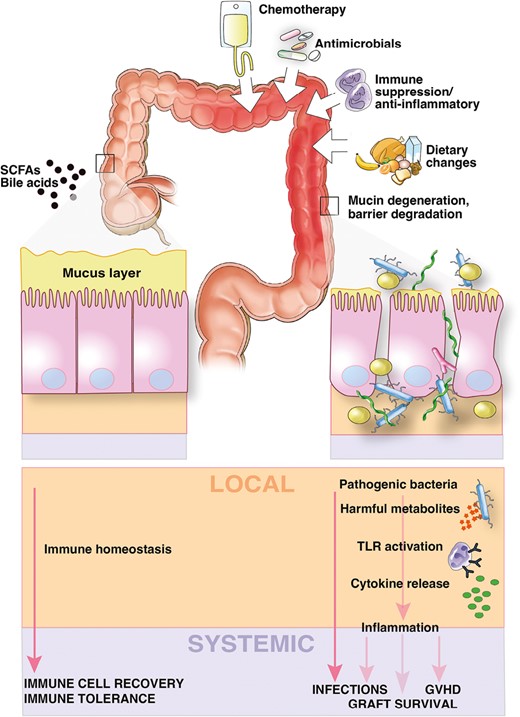
Key drivers of microbiome injury and microbiome–host interactions that influence outcomes after transplantation. In health (shown on the left), diverse gut microbes and microbial metabolites maintain epithelial barrier integrity, resist pathogen expansion, and promote host immune recovery and tolerance. During transplantation (shown on the right), dietary changes, antibiotics, and immunosuppressive medications alter the microbiome, leading to the loss of putatively beneficial bacteria and the expansion of potential pathogens that produce harmful metabolites that promote inflammation and alter the intestinal microenvironment. These changes leave the host susceptible to bacterial translocation and initiate an inflammatory cascade that can result in adverse outcomes of transplantation such as GVHD and graft failure. GVHD, graft-versus-host disease; SCFA, short-chain fatty acid; TLR, Toll-like receptor.
ANTIBIOTIC RESISTANCE IN PEDIATRIC TRANSPLANT RECIPIENTS
Transplant recipients have a heightened risk of colonization and infection by multidrug-resistant organisms (MDROs) due to exposure to healthcare settings, compromised immunity, and frequent receipt of broad-spectrum antibiotics. Studies indicate that over 50% of adult transplant recipients are colonized by MDROs with associated increased risks of infection and all-cause mortality [20, 21]. While the prevalence of MDRO colonization may be lower in pediatric transplant recipients, the risk of progression to infection remains high [22–24] and is associated with substantial morbidity and mortality [25]. Recent research highlights the role of antibiotics as a risk factor for MDRO infections among transplant recipients. For instance, antibiotic exposures preceded the onset of most mucosal barrier-injury bloodstream infections caused by MDROs in pediatric HCT recipients and were associated with an increase in the abundance of the infection-causing strain in the gut microbiota [26]. Consequently, there is growing interest in strategies to eradicate MDRO colonization prior to transplantation. Fecal microbiota transplantation (FMT) has shown promising results, with a meta-analysis suggesting approximately 70% efficacy [27], but experience in children being evaluated for transplantation is limited [28, 29].
Traditionally, studies of transplant recipients have utilized culture or PCR to identify colonization by specific MDROs or antibiotic resistance genes. However, metagenomic sequencing is increasingly being used to detect the complete set of resistance genes, known as the “resistome.” These studies reveal that the gut resistome of HCT and SOT recipients is more diverse and abundant than that of healthy individuals [30, 31]. Moreover, the resistomes of transplant recipients are highly dynamic, with antibiotics playing a major role in shaping the resistome after transplantation. Notably, antibiotics promote the acquisition and expansion of resistance genes to that class of antibiotic and to other antibiotic classes [30, 31]. Recent research in pediatric HCT recipients also suggests that different antibiotics have varying effects on the gut resistome, with antibiotics with an anaerobic spectrum of activity promoting the acquisition of new resistance genes and increasing the overall abundance of resistance genes within the gut microbiome [31]. An improved understanding of the impact of antibiotics and other interventions (eg, immunosuppressive medications, radiation therapy) on the gut resistome could guide clinical management and antimicrobial stewardship efforts in pediatric transplant recipients.
THE MICROBIOME IN HEMATOPOIETIC CELL TRANSPLANTATION
Over the past several decades, a growing body of literature has implicated the gut microbiome as a modifier of outcomes after HCT. Patients undergoing evaluation for HCT typically have gut microbiomes that differ from healthy individuals before transplantation due to prior healthcare exposures [32–34]. Further changes in the gut microbiome occur during HCT including a reduction in microbial diversity, loss of putatively beneficial anaerobes from the bacterial orders Clostridiales and Bacteriodales, and increases in the abundances of Enterobacteriaceae (eg, E. coli, Klebsiella), Enterococcus, and Streptococcus [32, 35]. After HCT, recovery of the gut microbiome typically follows a protracted course; for instance, the diversity and composition of the gut microbiota differed from the pre-HCT microbiota for 3 months after transplantation in a large adult cohort [36].
Mortality
The gut microbiome has also been shown to predict mortality after HCT. Studies of adult HCT recipients demonstrated that lower gut microbiome diversity at the time of neutrophil engraftment is associated with reduced overall survival and higher treatment-related mortality [32, 37]. Additionally, the composition of the gut microbiome has been associated with treatment-related mortality in this patient population, with higher risk observed with higher relative abundances of potential pathogens (eg, Gammaproteobacteria [37], Enterococcus [34]), a lower abundance of Blautia [38] (a commensal genus of Clostridiales), and lower urinary concentrations of 3-indole sulfate, a tryptophan metabolite produced by colonic bacteria [39]. Notably, the generalizability of these findings to pediatric HCT recipients is unknown because of the small sample sizes of pediatric cohorts studied to date. An additional challenge to interpreting these results is distinguishing the extent to which these associations reflect microbiome features serving as biomarkers of disease processes rather than true causal relationships.
Infections
The gut microbiome has also been shown to be a useful predictor of the risk of certain infections after HCT. For instance, in adult HCT recipients, a single taxon representing greater than 30% of the gut microbial population has been associated with an increased risk of bloodstream infection [35], with declining abundances of putatively beneficial bacteria frequently preceding bloodstream infection onset in this patient population [40]. The gut microbiome has also been linked to viral enterocolitis in pediatric HCT recipients [41], likely through alterations of gut epithelial architecture and nutrients [42]. Finally, the gut microbiome has been associated with the risk of infections at distant sites, including respiratory infections occurring after HCT in adults [43, 44], presumably through interactions with the host immune system.
GVHD
The gut microbiota is also linked to morbidity and mortality after HCT through its influence on T-cell alloreactivity and the development of GVHD. Lower diversity of the gut microbiome has been associated with an increased risk of acute GVHD in multiple adult [32, 45–48] and pediatric studies [17, 49]. Moreover, specific disruptions of gut microbiome composition have been associated with increased acute GVHD risk, including lower abundances of Clostridiales and Bacteroidales [49] and higher abundances of Enterobacteriaceae, Enterococcus [47], Prevotella, and Staphylococcus [41]. Short-chain fatty acids and other microbial metabolites may also influence the development of both acute and chronic GVHD in HCT recipients (Table 1). For example, lower abundances of butyrate-producing bacteria [45], depletion of genes for butyrate metabolism [17], and lower fecal levels of propionate and butyrate [49, 50] have all been associated with an increased risk of acute GVHD. Notably, many of the associations with specific microbes or microbial metabolites have not been consistently demonstrated in cohorts at different centers or in studies of both children and adults, hindering the development of reliable biomarkers or therapeutic targets for GVHD. Nonetheless, findings from these studies provide valuable insights into potential mechanisms by which the gut microbiota contributes to GVHD pathogenesis, including through immune activation by translocated bacteria [51], decreased production of anti-inflammatory metabolites [52, 53], overgrowth of mucin-degrading bacteria [16], and impaired bile acid metabolism [54].
Metabolite . | Proposed Mechanism of Action . | Host Target . | Microbial Association . | Pediatric Considerations . | HCT Associations . | SOT Associations . |
---|---|---|---|---|---|---|
Arginine | Arginine and polyamine derivatives inhibit pro-inflammatory (M1) macrophage activation | Macrophages | Bifidobacterium, Ruminococcus, Streptococcus mutans | Increased arginine metabolites in adult HCT recipients who did not develop GVHD [54]; higher arginine biosynthesis gene abundance predicted lower risk of acute GVHD in pediatric HCT recipients [41] | ||
Aryl hydrocarbon receptor ligands | Contextual, as T-cell ligands can result in the induction of FOXP3+ regulatory T cells; antigen-presenting cell ligands can increase IL-22 production | T and B cells, dendritic cells, macrophages, and mucosal cells | Widespread production | Diet influences ligand formation (eg, broccoli, parsley); potential for age-related variation | Metabolomic analysis of adult HCT recipients with acute GVHD showed decreased production of aryl hydrocarbon receptor ligands [54] | |
Inosine (or degradation products xanthine and hypoxanthine) | Interacts with adenosine α-2A receptor to stimulate Th1 differentiation (when co-stimulation is present); may require reduced gut integrity to translocate systemically [104] | T cells | Bifidobacterium pseudolongum; Akkermansia muciniphila; Lactobacillus johnsonii | Inosine-producing bacteria may vary across age groups (more prevalent in infants) | Theoretical role in acute rejection among SOT recipients [65] | |
Phenylacetylglutamine | Microbial phenylpyruvic acid production converted by host to phenylacetylglutamine which signals via α-2A, α-2B, and β2 adrenergic receptors and increases platelet adhesion and thrombus formation | Platelets | Widespread production by Bacteroides species and Clostridium asparagiforme | Diet plays a key role as is derived from phenylalanine-rich foods; potential for age-related variation | Theoretical role in vasculopathy during chronic organ rejection or transplant-associated thrombotic microangiopathy [65] | |
Secondary bile acids | Promote regulatory T-cell induction by direct binding to RORγT to block Th17 differentiation, increasing mitochondrial reactive oxygen species production, or binding to farnesoid X receptor in dendritic cells to decrease immunostimulation | T cells or dendritic cells | Clostridium; Parabacteroides; Bacteroides dorei; Alistipes; Lachnospiraceae; Hungatella hathewayi; Odoribacter laneus | Low levels of secondary bile acids were observed during early infancy but stable high levels by 3 years of age [105] | Metabolomic analysis of adult HCT recipients with acute GVHD showed alterations in bile acids [54] | |
Short-chain fatty acids | Promote peripheral regulatory T-cell generation, suppress Th17 generation, modulate macrophage function; butyrate may promote gut integrity | T cells, macrophages, intestinal epithelial cells | Widespread production | Children generally have higher abundances of bacteria that produce short-chain fatty acids in their microbiomes [41] | Mixed response: may protect against acute GVHD among adults [106], but also associated with risk of steroid-refractory acute GVHD [107]; linked to respiratory viral infections in adult HCT recipients [44] but not in pediatrics cohorts [41] | Loss of short-chain fatty acid producers associated with mycophenolate-induced enteropathy [108] |
Sulfobactin B | Interacts with transcription factors (eg, NFκβ) and DNA polymerase inhibitor to decrease response of macrophages to damage-associated molecular pattern (DAMP) signaling | Macrophages | Alistipes, Odoribacter, Chryseobacterium | Potential role in graft survival in murine models [64] |
Metabolite . | Proposed Mechanism of Action . | Host Target . | Microbial Association . | Pediatric Considerations . | HCT Associations . | SOT Associations . |
---|---|---|---|---|---|---|
Arginine | Arginine and polyamine derivatives inhibit pro-inflammatory (M1) macrophage activation | Macrophages | Bifidobacterium, Ruminococcus, Streptococcus mutans | Increased arginine metabolites in adult HCT recipients who did not develop GVHD [54]; higher arginine biosynthesis gene abundance predicted lower risk of acute GVHD in pediatric HCT recipients [41] | ||
Aryl hydrocarbon receptor ligands | Contextual, as T-cell ligands can result in the induction of FOXP3+ regulatory T cells; antigen-presenting cell ligands can increase IL-22 production | T and B cells, dendritic cells, macrophages, and mucosal cells | Widespread production | Diet influences ligand formation (eg, broccoli, parsley); potential for age-related variation | Metabolomic analysis of adult HCT recipients with acute GVHD showed decreased production of aryl hydrocarbon receptor ligands [54] | |
Inosine (or degradation products xanthine and hypoxanthine) | Interacts with adenosine α-2A receptor to stimulate Th1 differentiation (when co-stimulation is present); may require reduced gut integrity to translocate systemically [104] | T cells | Bifidobacterium pseudolongum; Akkermansia muciniphila; Lactobacillus johnsonii | Inosine-producing bacteria may vary across age groups (more prevalent in infants) | Theoretical role in acute rejection among SOT recipients [65] | |
Phenylacetylglutamine | Microbial phenylpyruvic acid production converted by host to phenylacetylglutamine which signals via α-2A, α-2B, and β2 adrenergic receptors and increases platelet adhesion and thrombus formation | Platelets | Widespread production by Bacteroides species and Clostridium asparagiforme | Diet plays a key role as is derived from phenylalanine-rich foods; potential for age-related variation | Theoretical role in vasculopathy during chronic organ rejection or transplant-associated thrombotic microangiopathy [65] | |
Secondary bile acids | Promote regulatory T-cell induction by direct binding to RORγT to block Th17 differentiation, increasing mitochondrial reactive oxygen species production, or binding to farnesoid X receptor in dendritic cells to decrease immunostimulation | T cells or dendritic cells | Clostridium; Parabacteroides; Bacteroides dorei; Alistipes; Lachnospiraceae; Hungatella hathewayi; Odoribacter laneus | Low levels of secondary bile acids were observed during early infancy but stable high levels by 3 years of age [105] | Metabolomic analysis of adult HCT recipients with acute GVHD showed alterations in bile acids [54] | |
Short-chain fatty acids | Promote peripheral regulatory T-cell generation, suppress Th17 generation, modulate macrophage function; butyrate may promote gut integrity | T cells, macrophages, intestinal epithelial cells | Widespread production | Children generally have higher abundances of bacteria that produce short-chain fatty acids in their microbiomes [41] | Mixed response: may protect against acute GVHD among adults [106], but also associated with risk of steroid-refractory acute GVHD [107]; linked to respiratory viral infections in adult HCT recipients [44] but not in pediatrics cohorts [41] | Loss of short-chain fatty acid producers associated with mycophenolate-induced enteropathy [108] |
Sulfobactin B | Interacts with transcription factors (eg, NFκβ) and DNA polymerase inhibitor to decrease response of macrophages to damage-associated molecular pattern (DAMP) signaling | Macrophages | Alistipes, Odoribacter, Chryseobacterium | Potential role in graft survival in murine models [64] |
GVHD, graft-versus-host disease; HCT, hematopoietic cell transplantation; SOT, solid organ transplantation.
Metabolite . | Proposed Mechanism of Action . | Host Target . | Microbial Association . | Pediatric Considerations . | HCT Associations . | SOT Associations . |
---|---|---|---|---|---|---|
Arginine | Arginine and polyamine derivatives inhibit pro-inflammatory (M1) macrophage activation | Macrophages | Bifidobacterium, Ruminococcus, Streptococcus mutans | Increased arginine metabolites in adult HCT recipients who did not develop GVHD [54]; higher arginine biosynthesis gene abundance predicted lower risk of acute GVHD in pediatric HCT recipients [41] | ||
Aryl hydrocarbon receptor ligands | Contextual, as T-cell ligands can result in the induction of FOXP3+ regulatory T cells; antigen-presenting cell ligands can increase IL-22 production | T and B cells, dendritic cells, macrophages, and mucosal cells | Widespread production | Diet influences ligand formation (eg, broccoli, parsley); potential for age-related variation | Metabolomic analysis of adult HCT recipients with acute GVHD showed decreased production of aryl hydrocarbon receptor ligands [54] | |
Inosine (or degradation products xanthine and hypoxanthine) | Interacts with adenosine α-2A receptor to stimulate Th1 differentiation (when co-stimulation is present); may require reduced gut integrity to translocate systemically [104] | T cells | Bifidobacterium pseudolongum; Akkermansia muciniphila; Lactobacillus johnsonii | Inosine-producing bacteria may vary across age groups (more prevalent in infants) | Theoretical role in acute rejection among SOT recipients [65] | |
Phenylacetylglutamine | Microbial phenylpyruvic acid production converted by host to phenylacetylglutamine which signals via α-2A, α-2B, and β2 adrenergic receptors and increases platelet adhesion and thrombus formation | Platelets | Widespread production by Bacteroides species and Clostridium asparagiforme | Diet plays a key role as is derived from phenylalanine-rich foods; potential for age-related variation | Theoretical role in vasculopathy during chronic organ rejection or transplant-associated thrombotic microangiopathy [65] | |
Secondary bile acids | Promote regulatory T-cell induction by direct binding to RORγT to block Th17 differentiation, increasing mitochondrial reactive oxygen species production, or binding to farnesoid X receptor in dendritic cells to decrease immunostimulation | T cells or dendritic cells | Clostridium; Parabacteroides; Bacteroides dorei; Alistipes; Lachnospiraceae; Hungatella hathewayi; Odoribacter laneus | Low levels of secondary bile acids were observed during early infancy but stable high levels by 3 years of age [105] | Metabolomic analysis of adult HCT recipients with acute GVHD showed alterations in bile acids [54] | |
Short-chain fatty acids | Promote peripheral regulatory T-cell generation, suppress Th17 generation, modulate macrophage function; butyrate may promote gut integrity | T cells, macrophages, intestinal epithelial cells | Widespread production | Children generally have higher abundances of bacteria that produce short-chain fatty acids in their microbiomes [41] | Mixed response: may protect against acute GVHD among adults [106], but also associated with risk of steroid-refractory acute GVHD [107]; linked to respiratory viral infections in adult HCT recipients [44] but not in pediatrics cohorts [41] | Loss of short-chain fatty acid producers associated with mycophenolate-induced enteropathy [108] |
Sulfobactin B | Interacts with transcription factors (eg, NFκβ) and DNA polymerase inhibitor to decrease response of macrophages to damage-associated molecular pattern (DAMP) signaling | Macrophages | Alistipes, Odoribacter, Chryseobacterium | Potential role in graft survival in murine models [64] |
Metabolite . | Proposed Mechanism of Action . | Host Target . | Microbial Association . | Pediatric Considerations . | HCT Associations . | SOT Associations . |
---|---|---|---|---|---|---|
Arginine | Arginine and polyamine derivatives inhibit pro-inflammatory (M1) macrophage activation | Macrophages | Bifidobacterium, Ruminococcus, Streptococcus mutans | Increased arginine metabolites in adult HCT recipients who did not develop GVHD [54]; higher arginine biosynthesis gene abundance predicted lower risk of acute GVHD in pediatric HCT recipients [41] | ||
Aryl hydrocarbon receptor ligands | Contextual, as T-cell ligands can result in the induction of FOXP3+ regulatory T cells; antigen-presenting cell ligands can increase IL-22 production | T and B cells, dendritic cells, macrophages, and mucosal cells | Widespread production | Diet influences ligand formation (eg, broccoli, parsley); potential for age-related variation | Metabolomic analysis of adult HCT recipients with acute GVHD showed decreased production of aryl hydrocarbon receptor ligands [54] | |
Inosine (or degradation products xanthine and hypoxanthine) | Interacts with adenosine α-2A receptor to stimulate Th1 differentiation (when co-stimulation is present); may require reduced gut integrity to translocate systemically [104] | T cells | Bifidobacterium pseudolongum; Akkermansia muciniphila; Lactobacillus johnsonii | Inosine-producing bacteria may vary across age groups (more prevalent in infants) | Theoretical role in acute rejection among SOT recipients [65] | |
Phenylacetylglutamine | Microbial phenylpyruvic acid production converted by host to phenylacetylglutamine which signals via α-2A, α-2B, and β2 adrenergic receptors and increases platelet adhesion and thrombus formation | Platelets | Widespread production by Bacteroides species and Clostridium asparagiforme | Diet plays a key role as is derived from phenylalanine-rich foods; potential for age-related variation | Theoretical role in vasculopathy during chronic organ rejection or transplant-associated thrombotic microangiopathy [65] | |
Secondary bile acids | Promote regulatory T-cell induction by direct binding to RORγT to block Th17 differentiation, increasing mitochondrial reactive oxygen species production, or binding to farnesoid X receptor in dendritic cells to decrease immunostimulation | T cells or dendritic cells | Clostridium; Parabacteroides; Bacteroides dorei; Alistipes; Lachnospiraceae; Hungatella hathewayi; Odoribacter laneus | Low levels of secondary bile acids were observed during early infancy but stable high levels by 3 years of age [105] | Metabolomic analysis of adult HCT recipients with acute GVHD showed alterations in bile acids [54] | |
Short-chain fatty acids | Promote peripheral regulatory T-cell generation, suppress Th17 generation, modulate macrophage function; butyrate may promote gut integrity | T cells, macrophages, intestinal epithelial cells | Widespread production | Children generally have higher abundances of bacteria that produce short-chain fatty acids in their microbiomes [41] | Mixed response: may protect against acute GVHD among adults [106], but also associated with risk of steroid-refractory acute GVHD [107]; linked to respiratory viral infections in adult HCT recipients [44] but not in pediatrics cohorts [41] | Loss of short-chain fatty acid producers associated with mycophenolate-induced enteropathy [108] |
Sulfobactin B | Interacts with transcription factors (eg, NFκβ) and DNA polymerase inhibitor to decrease response of macrophages to damage-associated molecular pattern (DAMP) signaling | Macrophages | Alistipes, Odoribacter, Chryseobacterium | Potential role in graft survival in murine models [64] |
GVHD, graft-versus-host disease; HCT, hematopoietic cell transplantation; SOT, solid organ transplantation.
Immune Recovery
Several recent studies suggest that the microbiome plays a role in immune reconstitution after HCT. In a small study of pediatric HCT recipients, specific microbiome profiles were associated with patterns of reconstitution of several immune cell populations, including inflammatory T, natural killer, and B cells [55]. Similarly, the abundances of specific bacterial genera within the gut microbiome were associated with the dynamics of neutrophil, lymphocyte, and monocyte recovery in adult HCT recipients [56]; in this same study, autologous FMT was shown to boost numbers of these immune cell populations during engraftment [56].
THE MICROBIOME IN SOLID ORGAN TRANSPLANTATION
Comparatively few studies have examined the microbiome in SOT recipients; however, the limited data available suggest that the microbiome may play a role in the development of infections after SOT. Specifically, among adult liver and renal transplant recipients, gut microbiome composition has been associated with the risks of respiratory viral infections [57], MDRO colonization [21], and other infections [58] after transplantation. Most studies on the microbiome in SOT recipients have focused on its potential role in organ rejection and graft function, while others have highlighted how immunosuppressive medications can impact the microbiome and, in turn, how gut microbes can affect the metabolism of these medications.
Graft Rejection and Function
The risk and intensity of alloreactive immune responses following SOT is heavily influenced by the degree of genetic disparity between the donor and recipient, but various other patient factors (eg, diet, infections) also influence the development of graft rejection. The gut microbiome can influence local immune responses at the site of the transplant or systemic responses at sites distant from the transplant. For instance, a higher risk of acute cellular rejection has been correlated with the abundances of specific bacterial populations in the gut microbiomes of pediatric intestinal (eg, lower abundance of putatively beneficial Lactobacillales, higher abundance of Enterobacteriaceae) [59] and adult liver (eg, higher abundance of Enterobacteriaceae) [60] transplant recipients. Additionally, in adult renal transplant recipients, the urinary microbiome has been linked to spontaneous transplantation tolerance, which enables the withdrawal of immunosuppressive medications [61]. Animal models of liver, lung, and skin transplantation have further demonstrated the influential role of the gut microbiota in organ graft rejection [62–64].
As in HCT recipients, associations between the microbiome and alloreactive immune responses are mediated in part through the production of microbial metabolites (Table 1) [65]. For example, sulfobacin B, a lipid compound produced by commensal bacteria from the genus Alistipes, has been associated with prolonged graft survival and reduced tumor necrosis factor production by macrophages in a murine skin transplantation model [64]. Additionally, a high-fiber diet or dietary supplementation with acetate was associated with the prolongation of graft survival through induction of regulatory T-cell responses in a murine model of renal transplantation [66]. Other microbial metabolites, such as trimethyl-amine N-oxide and phenylacetylglutamine, have been proposed to play a role in vasculopathy, a common feature of chronic organ rejection [67, 68].
Both local and distant microbial populations influence graft survival and function after SOT. For instance, the lung microbiome before transplantation modifies the risk of bronchiolitis obliterans syndrome among adult lung transplant recipients by affecting the microbes that colonize the transplanted organs [69]. Moreover, modulation of the gut microbiome through the administration of Bifidobacterium pseudolongum prolonged the duration of graft survival in mice undergoing cardiac transplantation [70], while administration of Bifidobacterium longum, Lactobacillus acidophilus, and Enterococcus faecalis was associated with reduced liver injury in a mouse model of liver transplantation [71]. Additionally, in adult renal transplant recipients, higher levels of the potentially beneficial Lachnospiraceae and Veillonellaceae after transplantation were associated with improved graft function [72].
Immunosuppressive Medications
The medications routinely used to suppress immune responses and prevent organ rejection in SOT recipients may also impact the microbiome. For example, tacrolimus has been shown to result in alterations in gut microbiome composition when administered to mice [73]. Conversely, microbial populations can metabolize immunosuppressive medications and thereby influence their effectiveness and toxicity. For instance, investigators recently observed that adults who had a high abundance of Faecalibacterium prausnitzii in their gut microbiome required higher doses of tacrolimus in the first month after renal transplantation [74]. Subsequent screening of strains of F. prausnitzii and other bacterial species demonstrated that many Clostridiales metabolize tacrolimus to less active compounds, reducing enteral absorption and contributing to the variable tacrolimus exposures observed with enteral dosing [75]. The microbiome has also been shown to modulate adverse effects associated with immunosuppressive medications. Gastrointestinal toxicity from mycophenolate mofetil was not observed in germ-free mice, suggesting that the development of this adverse effect requires an intact microbiota [76]. Additionally, in mice, the development of sirolimus and tacrolimus-induced hyperglycemia can be prevented by administration of Lactobacillus plantarum [77] or the short-chain fatty acid butyrate [78].
INTERVENTIONS TO MODIFY THE MICROBIOME
In contrast to most other patient factors that influence transplantation outcomes, the gut microbiome is amenable to modification. Thus, interventions that modify the microbiome have enormous promise to improve the outcomes of pediatric transplantation. Use of such microbiome-based therapies in transplant recipients has recently gained interest. Strategies currently being investigated include new therapies and modifications of supportive care practices that affect the microbiome (Figure 3).
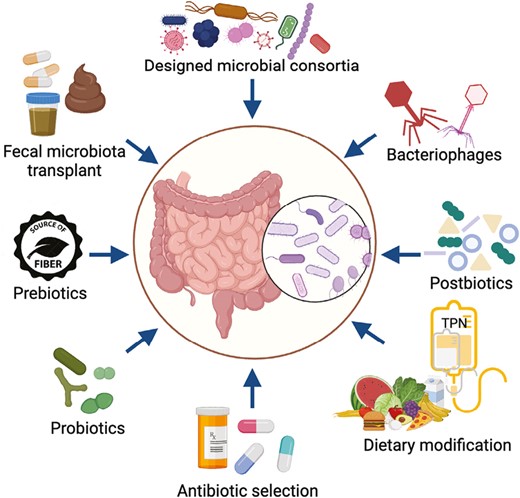
Microbiome-based interventions in development or under investigation for use in transplant recipients. Strategies that seek to directly alter the microbiome include prebiotics (nonviable substances that promote the growth of beneficial microbes), probiotics (live microorganisms that confer a potential health benefit), bacteriophages that enable targeted elimination of harmful bacteria, transfer of entire gut microbial communities through fecal microbiota transplantation, and administration of microbial consortia containing multiple live bacterial strains. Other strategies such as dietary modifications and selection of specific antibiotics for prophylaxis or treatment indirectly modify the microbiome with the potential to influence transplantation outcomes. Created with Biorender.com.
Dietary Modification
The gut microbiome metabolizes dietary products that the host cannot, and diet alters the composition of microbial communities, with the potential to influence clinical outcomes [79]. For example, enteral nutrition has been linked to higher microbiome diversity, improved survival, and lower risks of infection and GVHD in pediatric HCT recipients [30, 80, 81]. In contrast, parenteral nutrition has been associated with negative effects on the gut microbiome, including loss of Blautia, altered production of short-chain fatty acids, and bacterial translocation [38, 82].
Perioperative Antibiotics
Administration of antibiotics to SOT recipients alters the microbiome and may have beneficial or detrimental effects that vary based on the organ transplanted. Perioperative antibiotics have been associated with a reduction in the immune infiltrate responding to ischemia-reperfusion injury in adult liver transplant recipients [83] and with improved graft outcomes in mice undergoing cardiac or skin transplantation [70, 84]. In contrast, perioperative antibiotics have been associated with worse outcomes following transplantation of other organs, possibly due to effects on immunosuppressive drug metabolism or through infection risk [57, 65, 73].
Antibiotic Selection
Antibiotics are often prescribed for infection prophylaxis or treatment in transplant recipients with little consideration of the effects on potentially beneficial microbes. However, several recent studies indicate that antibiotic selection can have a substantial impact on outcomes after HCT [85]. Early investigation of antibiotics in this patient population evaluated their utility for GVHD prevention and involved administration of high doses of nonabsorbable oral antibiotics; although this approach was not consistently shown to prevent GVHD and was largely abandoned for this purpose, data from a recent pilot clinical trial suggest that it may reduce the risk of bloodstream infection after HCT [86]. More recent efforts to mitigate the risks of GVHD and infection after HCT have largely focused on the selection of antibiotics to minimize off-target effects on putatively beneficial microbes. In particular, studies of adult and pediatric HCT recipients have consistently reported associations between exposure to antibiotics with anaerobic spectra of activity and both gut microbiome alterations (eg, lower microbial diversity [39], loss of Bacteroidetes [16] or Clostridiales [17, 18]) and an increased risk of acute GVHD of the gut or liver [16, 28]. Experiments conducted in mice suggest that these associations may be causal; for instance, mice treated with imipenem–cilastatin or piperacillin–tazobactam had higher GVHD mortality after allogeneic HCT than mice treated with aztreonam [16, 18]. An ongoing clinical trial that is randomizing adult allogeneic HCT recipients with febrile neutropenia to receipt of cefepime or piperacillin–tazobactam will evaluate for such a causal relationship in humans (ClinicalTrials.gov: NCT03078010). In an analogous manner, preservation of certain gut bacteria was associated with protection from chronic rejection in a mouse model of lung transplantation [63], suggesting that judicious use of antibiotics could also promote immune tolerance in SOT recipients.
Prebiotics
Prebiotics are nonviable substances that promote the growth of beneficial microorganisms. Several of these agents, including human milk oligosaccharides [87], fructo-oligosacchrides [88], and a combination of glutamine, fiber, and oligosaccharides [89] have been evaluated in transplant recipients. These products have generally been shown to be safe and to have modest effects on gut microbiome composition and markers of inflammation, though with limited data for clinical benefits [87–89]. Importantly, the benefits of prebiotics in transplant recipients may be limited by the substantial heterogeneity of the gut microbiome in these patient populations and the detrimental effects of antibiotics on the beneficial microbes targeted by these products.
Probiotics
Probiotics are live microbes that when consumed confer potential health benefits to the host. Products differ by species, dose, and preparation, all of which influence the ability of these microbes to colonize or impact the resident gut microbiota. Despite promising preclinical experiments in mice [90], Bifidobacterium and Lactobacillus-based probiotics have lacked clinical efficacy in small studies of SOT [91] and HCT [92] recipients. For instance, a recent study evaluating L. plantarum for the prevention of acute GVHD in pediatric HCT recipients closed prematurely because the interim analysis did not support efficacy (ClinicalTrials.gov: NCT03057054). Other related interventions, including synbiotics (mixtures of prebiotics and probiotics) and postbiotics (microbe-derived metabolites), have thus far not been studied in transplant populations.
FMT
FMT has proven effective for treating Clostridiodes difficile infection in immunocompetent patients [93] and, although its efficacy is somewhat lower in SOT recipients [94], it was effective in small trials involving HCT recipients [95, 96]. FMT is also being investigated for the eradication of gut colonization by MDROs prior to transplantation [29] and the prevention or treatment of acute gut or liver GVHD, with favorable responses reported in a growing number of cases of steroid-refractory GVHD [97–99]. Serious infections resulting from FMT have been reported [100] and further research is needed to optimize processes for donor selection and sample processing to ensure safe use of this therapy in pediatric transplant recipients.
FUTURE DIRECTIONS
Microbiome diversity and composition differ among children and adults. During childhood, there are rapid shifts in microbial community structure that coincide with the maturation of the immune system. Therefore, findings from studies of the microbiome in adult transplant recipients may not be generalizable to pediatric populations. In addition, the indications for transplantation, diet, immune cell populations, and risks of infections and noninfectious complications of transplantation differ among children and adults. Despite recent advances in our understanding of the microbiome’s importance to the outcomes of transplantation, there are several major gaps in our knowledge. Most studies have focused only on bacteria, leaving fungi, viruses, and archaea largely unexplored. There is increasing interest in studying these microbial populations in transplant recipients, with the few studies conducted to date suggesting potential roles in the pathogenesis of GVHD [101, 102] and solid organ graft dysfunction [103]. Additionally, further research is needed both to identify the causal pathways underlying associations between the microbiome and transplantation outcomes and to expeditiously translate therapies likely to benefit transplant recipients. As our understanding of the microbiome improves and new tools to modify the microbiome emerge, there is the potential for the development of individualized microbiome-targeted therapies that prevent infections and other complications among pediatric transplant recipients.
Notes
Financial support: C.W.E. (K23-HL161309) and M.S.K. (K23-AI135090) were supported by Career Development Awards from the National Institutes of Health.
Supplement sponsorship. This article appears as part of the supplement “Advances in Pediatric Transplant Infectious Diseases,” sponsored by Eurofins Viracor.
Potential conflicts of interest: All authors: No reported conflicts.
REFERENCES
Author notes
Caitlin W Elgarten and Elisa B Margolis contributed equally to this work.