-
PDF
- Split View
-
Views
-
Cite
Cite
Heidi E. Brown, Jonathan Cox, Andrew C. Comrie, Roberto Barrera, Habitat and Density of Oviposition Opportunity Influences Aedes aegypti (Diptera: Culicidae) Flight Distance, Journal of Medical Entomology, Volume 54, Issue 5, September 2017, Pages 1385–1389, https://doi.org/10.1093/jme/tjx083
- Share Icon Share
Abstract
Understanding the dispersal of Aedes (aegypti (L.) Diptera: Culicidae) after consuming a potentially infectious bloodmeal is an important part of controlling the spread of the arboviruses it transmits. Because of the impact on abundance, removal of oviposition sites is a key component of vector control. However, source reduction around a case may encourage dispersal of potentially infected vectors. We compare the effect of oviposition site availability on Ae. aegypti dispersal behavior within 30-m linear cages in three model ecosystems at the University of Arizona’s Biosphere 2 research facility. We found a significant interaction effect in which, when oviposition site density was sparse, dispersal was greater in the highly vegetated humid rainforest and limited in the low vegetation, arid desert model ecosystem. When oviposition site density was dense, no significant effect on dispersal was observed. These analyses support the idea that source reduction has an important influence on the distance that gravid, potentially infected, females will travel.
Dengue, chikungunya, and Zika are important mosquito-borne diseases, occurring primarily in the tropics and transmitted principally by the yellow fever mosquito, Aedes aegypti (L.) (Diptera: Culicidae). Aedes aegypti is not considered a far dispersing mosquito (Pan American Health Organization 1994). Understanding the factors influencing flight range after the vector consumes a potentially infectious bloodmeal is an important step toward improving disease prevention. If the vector does not fly far after infection, prevention should focus on the immediate vicinity of infected homes. If, however, the vector disperses over greater distances, then alternative strategies become more important in the midst of an epidemic. Since at least the 1960s, the implications of flight range on for successful use of modified-mosquito releases for vector control have been discussed, specifically that more frequent releases may be necessary when mosquitoes disperse only short distances (Schoof 1967).
Rubidium-labeled Ae. aegypti released into an open area with oviposition sites calculated the mean distance traveled within 40–67 h after feeding to be 181 m (range 9–432 m; Reiter et al. 1995). Distances up to 320 m were recorded for rubidium-labeled Aedes albopictus (Skuse) and Ae. aegypti across an urban area (Liew and Curtis 2004). Mean travel distances of 288 m (maximum 690 m) were reported from a mark–release–recapture study using indoor traps only, though only 6.3% of released mosquitoes were recaptured (Maciel-de-Freitas and Lourenco-de-Oliveira 2009). A clustering effect was found among adult Ae. aegypti collections for 10 m around homes (with weak clustering up to 30 m) in Iquitos, Peru (Getis et al. 2003). A mean travel distance of 77.8 m was reported using outdoor sticky traps, though 23.1% of the recaptured Ae. aegypti were collected further than 100 m and one female was captured at the 200 m study boundary (Russell et al. 2005).
Aedes aegypti marked and released within the home were often recaptured within the home or the neighboring home, with rare recaptures of up to 512 m (Harrington et al. 2005). Despite low recapture rates (38%, which the authors attributed to mortality), 48% of recaptures were within the same home in which they were released, and only 4% dispersed beyond 40 m (Mcdonald 1977). These findings indicate that although occasional long distance dispersal is possible, most Ae. aegypti activity remains close to the home (within about 30 m).
Removal of oviposition sites is a key component of vector control in response to Ae. aegypti disease prevention under the premise that, by removing oviposition sources, vector abundance and the associated disease risk is reduced (Barrera et al. 2002). The counter argument has been made, namely, that source reduction around a case may encourage greater dispersal of potentially infected vectors (Reiter et al. 1995, Reiter 2007). In fact, Edman et al. (1998) manipulated oviposition sites around homes where Ae. aegypti were released and found that of the 7% that dispersed outside the home in which they were released, significantly more were recaptured around homes with a greater number of oviposition sites.
We sought to determine how environmental conditions and distance between oviposition sites interact to influence Ae. aegypti oviposition behavior. Using locally collected Ae. aegypti that were reared in the laboratory for no more than two generations, we compared behavior among three model ecosystems at Biosphere 2: tropical rainforest, savanna, and fog desert, and under two oviposition conditions: dense (an oviposition container every 1.5 m) or limited (an oviposition container every 11 m). The results are discussed within the context of source reduction control strategies.
Materials and Methods
Mosquito Maintenance
Although the viruses it transmits do not currently circulate in southern Arizona, Ae. aegypti is a dominant mosquito species throughout urban areas of the region (Fink et al. 1998). Aedes aegypti larvae and pupae were collected from a flowerpot saucer in the Palo Verde neighborhood (centroid: 32.243361, −110.918267) of Tucson, AZ. A subset of the adults was confirmed to be Ae. aegypti and a colony established and maintained in 0.02-cubic meter tabletop incubators (Quincy Lab Inc., Chicago, IL) at a median temperature of 26.5 °C (Interquartile range [IQR]=3) and median 97.5% relative humidity (IQR = 15%). Adults were provided access to a 10% sucrose solution via cotton balls ad libitum. Colony mosquitos were provided a bloodmeal twice, weekly delivered via hog casing (Dewied International Inc., San Antonio, TX) covered glass bells (U Arizona, Chemistry Glassblowing Facility, Tucson, AZ) warmed to 38 °C using a Isotemp Digital-Control Water Bath: Model 205 (Thermo Fisher Scientific, Waltham, MA). Whole blood collected in citrate phosphate dextrose anticoagulant (∼7 ml whole blood: 1 ml anticoagulant) from volunteer allogeneic donors meeting all FDA mandated criteria was supplied by the American Red Cross Biomedical Services (ARC IRB number 2016-005).
Study Location
This study was conducted in the University of Arizona’s Biosphere 2 research facility (Osmond et al. 2004). Three of the Biosphere 2’s seven model ecosystems were used, within the range of climate and vegetation conditions: the mature tropical rainforest, tropical savanna grassland, and coastal fog desert. An EL-USB-2 weather data logger (Lascar Electronics, Whiteparish, United Kingdom) was placed in each model ecosystem, measuring hourly temperature and relative humidity. The median daily maximum temperature was highest in the fog desert (median = 42.0 °C, IQR = 4.75), whereas the median daily maximum temperature was similar between the savanna (median = 30.5 °C, IQR = 3.0) and rainforest (median = 30.5 °C, IQR = 2.5). None of the median daily minimum temperatures neared freezing (median 24.5 °C, 23 °C, and 22.5 °C, in fog desert, savanna, and rainforest, respectively). Humidity was highest (mean = 93.5%, standard deviation [SD] = 9.1%) in the rainforest, followed by the savanna (mean = 75%, SD = 11.7%), and lowest in the fog desert (mean 49.7%, SD = 14.6%).
In each of the three model ecosystems, we constructed a 0.3- by 0.3- by 21-m-long flight cage constructed of a 2.5-cm PVC frame wrapped in gray fiberglass insect screen (WSG Industries, Inc., Laguna Beach, CA; Fig. 1). The cage dimensions were selected to maximize distance while remaining uniform across the three model ecosystems. Oviposition opportunities were provided by 6-ounce plastic containers with water and seed germination paper. No other oviposition sites were available within the experimental cages.
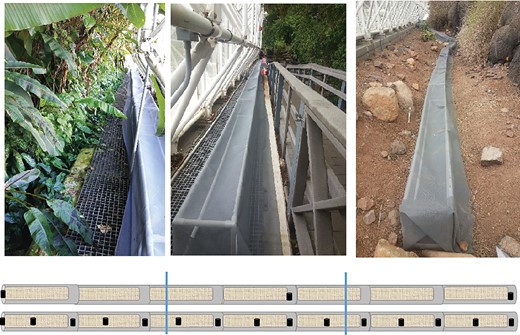
Images of the flight cages in each of the model ecosystems, from left to right: tropical rainforest, savanna, coastal fog desert. Below the photographs are schematics of the experimental cages, with the sparse oviposition condition represented above and the dense condition below. The black squares represent oviposition containers and the vertical lines indicate how the cages were divided into proximal, medial, and distal sections for analysis.
Experiment
Cohorts of 4–8-d-old females were offered a 2 ml bloodmeal after being starved (only water) for 2 d. Visibly blood-fed females were aspirated into 16-ounce plastic containers with mesh lids, provided sucrose soaked cotton, and returned to the incubator until transport to the experiment site.
Within 24 h of feeding, they were transported to Biosphere 2 and released into the flight cages. On alternating experimental replicates, we tested two scenarios spanning the length (0–21.3 m) of the flight cage. For the sparse condition (Fig. 1 top schematic), oviposition containers were placed every 11 m. For the dense condition (Fig. 1 bottom schematic), oviposition containers were placed every 1.5 m. Blood-fed female mosquitoes were released at alternating ends of the flight cage to account for microhabitat differences. After 1 wk, egg papers were removed, transported to the laboratory, and counted using a stereo microscope (VWR International, Randor, PA).
Analysis
Replicates in which at least 10 eggs were recovered were used for the analysis (n = 35/49 experiments conducted) and the proportion of total eggs in each oviposition container calculated to make the runs comparable, irrespective of total number of eggs recovered (Table 1). The data at 1.5-m intervals were aggregated into those eggs that were laid in “proximal” (all eggs deposited <7.6 m), “medial” (all eggs deposited 7.6–13.7 m), and “distal” (all eggs deposited >13.7 m) containers to assess general trends.
Summary of the experiments in which enough eggs were recovered to use the data (N > 10)
. | . | % Eggsa per distance . | ||||
---|---|---|---|---|---|---|
. | No. of replicates . | Mean no. of mosquitoes per replicate . | Mean no. of eggs recoveredb . | Proximal . | Medial . | Distal . |
Sparse (11 m) | ||||||
Rainforest | 3 | 11.7 | 56.3 | 3.1 | 17.8 | 59.5 |
Savanna | 9 | 9.9 | 156.7 | 36.7 | 19.0 | 26.1 |
Fog desert | 4 | 9.5 | 111.0 | 72.0 | 12.4 | 6.2 |
Dense (1.5 m) | ||||||
Rainforest | 5 | 9.6 | 76.4 | 10.0 | 7.5 | 55.5 |
Savanna | 7 | 10 | 174.7 | 37.8 | 7.6 | 28.3 |
Fog desert | 7 | 10 | 186.3 | 35.2 | 23.7 | 29.9 |
. | . | % Eggsa per distance . | ||||
---|---|---|---|---|---|---|
. | No. of replicates . | Mean no. of mosquitoes per replicate . | Mean no. of eggs recoveredb . | Proximal . | Medial . | Distal . |
Sparse (11 m) | ||||||
Rainforest | 3 | 11.7 | 56.3 | 3.1 | 17.8 | 59.5 |
Savanna | 9 | 9.9 | 156.7 | 36.7 | 19.0 | 26.1 |
Fog desert | 4 | 9.5 | 111.0 | 72.0 | 12.4 | 6.2 |
Dense (1.5 m) | ||||||
Rainforest | 5 | 9.6 | 76.4 | 10.0 | 7.5 | 55.5 |
Savanna | 7 | 10 | 174.7 | 37.8 | 7.6 | 28.3 |
Fog desert | 7 | 10 | 186.3 | 35.2 | 23.7 | 29.9 |
Averaged across the square-root transformed data and then transformed back to percent.
We suspect that the reduced number of eggs in the rainforest is owing to ants removing the eggs.
Summary of the experiments in which enough eggs were recovered to use the data (N > 10)
. | . | % Eggsa per distance . | ||||
---|---|---|---|---|---|---|
. | No. of replicates . | Mean no. of mosquitoes per replicate . | Mean no. of eggs recoveredb . | Proximal . | Medial . | Distal . |
Sparse (11 m) | ||||||
Rainforest | 3 | 11.7 | 56.3 | 3.1 | 17.8 | 59.5 |
Savanna | 9 | 9.9 | 156.7 | 36.7 | 19.0 | 26.1 |
Fog desert | 4 | 9.5 | 111.0 | 72.0 | 12.4 | 6.2 |
Dense (1.5 m) | ||||||
Rainforest | 5 | 9.6 | 76.4 | 10.0 | 7.5 | 55.5 |
Savanna | 7 | 10 | 174.7 | 37.8 | 7.6 | 28.3 |
Fog desert | 7 | 10 | 186.3 | 35.2 | 23.7 | 29.9 |
. | . | % Eggsa per distance . | ||||
---|---|---|---|---|---|---|
. | No. of replicates . | Mean no. of mosquitoes per replicate . | Mean no. of eggs recoveredb . | Proximal . | Medial . | Distal . |
Sparse (11 m) | ||||||
Rainforest | 3 | 11.7 | 56.3 | 3.1 | 17.8 | 59.5 |
Savanna | 9 | 9.9 | 156.7 | 36.7 | 19.0 | 26.1 |
Fog desert | 4 | 9.5 | 111.0 | 72.0 | 12.4 | 6.2 |
Dense (1.5 m) | ||||||
Rainforest | 5 | 9.6 | 76.4 | 10.0 | 7.5 | 55.5 |
Savanna | 7 | 10 | 174.7 | 37.8 | 7.6 | 28.3 |
Fog desert | 7 | 10 | 186.3 | 35.2 | 23.7 | 29.9 |
Averaged across the square-root transformed data and then transformed back to percent.
We suspect that the reduced number of eggs in the rainforest is owing to ants removing the eggs.
We evaluated the effect of model ecosystem and container density on distance dispersal of eggs in containers using a factorial ANOVA using Stata v12 (StataCorp LP, College Station, TX). Data were square-root transformed to achieve normality.
Results
Data were transformed to normal, and normality was confirmed using the skewness and kurtosis test for normality (sparse: χ2 = 2.53, P = 0.282; dense: χ2 = 4.63, P = 0.099). A factorial ANOVA was used with square-root transformed percent of eggs in the container as the dependent variable and model ecosystem and distance as independent variables, as well as the interaction of the independent variables. The two experimental designs, sparse and dense containers, were analyzed individually.
The overall model for the sparse container condition was statistically significant (F = 2.84, df = 8, P = 0.014). The main effects of model ecosystem and distance were not statistically significant (F = 0.21, df = 2, P = 0.809 and F = 1.04, df = 2, P = 0.362, respectively). However, the interaction between model ecosystem and distance was significant (F = 4.67, df = 4, P = 0.004), indicating that the distance traveled to lay eggs differed depending upon the model ecosystem. This effect is graphically represented in Fig. 2, with the percent of eggs laid in proximal containers being greater for experiments conducted in the fog desert and the opposite effect observed in the tropical rainforest. The overall model for the dense container condition aggregated to proximal, medial, or distal containers was not statistically significant (F = 1.88, df = 8, P = 0.085). Overlapping error bars under the dense oviposition condition in Fig. 2 shows this same effect.
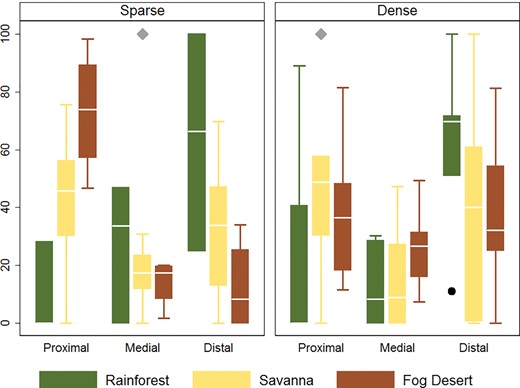
Box plots showing percent of eggs laid at each distance by model ecosystem. The left and right plots shows the sparse and dense (11 m and 1.5 m) intervals of oviposition containers.
Discussion
Using model ecosystems at Biosphere 2, we were able to simultaneously compare how habitat interacted with oviposition availability to influence dispersal of Ae. aegypti with limited rearing in the insectary (no more than two generations). When the availability of oviposition containers was sparse (11 m between containers), dispersal, measured as percent of total eggs laid, tended to be limited to proximal containers in the low vegetation, dryer fog desert but greater in distal containers in the highly vegetated, humid tropical rainforest. Our experimental design does not lend itself to understanding the biology behind what was observed; however, Rowley and Graham (1968) found that with extreme temperatures (>32 °C), low humidity had a limiting effect on Ae. aegypti flight. A possible explanation for the greater dispersal in the moderate temperatures and high humidity of the rainforest (Fig. 2) may similarly be owing to fewer limitations on oviposition seeking flight. Visual and olfactory cues may also have influenced dispersal (Day 2016). Alternating the end from which mosquitoes were released helped to control for these effects within each model ecosystem; however, such cues, in addition to humidity, may have influences on dispersal differences between model ecosystem and container density conditions.
When oviposition containers were dense (every 1.5 m), no effect of model ecosystem was observed. That is, the limited dispersal observed in the fog desert under the sparse container condition is lost when containers were more densely available. This indicates the importance of source reduction in arid places for disease control. The introduction of water storage in response to drought in Australia led to the creation of additional immature mosquito habitat, which was connected to the reintroduction of Ae. aegypti (Gibbons 2010). Furthermore, the additional habitat has implications for potential dengue outbreaks (Beebe et al. 2009). Though not significant, the trend in the rainforest, regardless of container density, tended toward distal dispersal.
The generalizability of our results is limited in that the constructed cages were restrictive to vertical flight. We used Ae. aegypti that were collected from, and likely adapted to, the arid urban Tucson environment and cannot assess whether our observations are influenced by the strain of Ae. aegypti used. Some of these issues might be explored with comparative mark–release–recapture field studies using wild captured Ae. aegypti in similar environments to what was available in Biosphere 2.
Although similar numbers of mosquitoes were released in each of the three biomes (Table 1), there were fewer eggs recovered in the rainforest. We suspect this was owing to predation by ants or other insects, based on our observations of ants capturing mosquitoes as well as literature citing predation (Russell et al. 2001) and specifically predation by ants on eggs of other mosquito species (Lee et al. 1994). Release of mosquitoes from alternating ends of the cage and analysis by percent of total eggs collected rather than counts were used to address possible bias introduced by ant predation or other microhabitat differences.
Nonetheless, these analyses support the idea that source reduction has important implications for the distance that gravid, potentially infected, females will travel. In the more arid environment of the Biosphere 2 fog desert, when oviposition containers were sparse, dispersal was limited. Water storage and the lack of waste removal have been shown to provide oviposition opportunities (Lloyd et al. 1994, Barrera et al. 1995, Hayes et al. 2003, Gibbons 2010). Mosquito abundance, in turn, is positively associated with dengue cases during outbreaks (Rodriguez-Figueroa et al. 1995).
That greater dispersal was observed when oviposition containers were sparse in the rainforest was not expected. This seems to support the hypothesis that source reduction around a case may facilitate dispersal (Reiter et al. 1995, Edman et al. 1998, Reiter 2007). Our results indicate that container density may not only have implications on vector abundance but also on influencing dispersal behavior. Assessing the implications of this might be best achieved through simulation modelling evaluating the differential impact of increased abundance versus dispersal.
Acknowledgments
Research reported in this publication was supported in part by the National Institute of Allergy and Infectious Diseases of the National Institutes of Health under Award number K01AI101224. The content is solely the responsibility of the authors and does not necessarily represent the official views of the National Institutes of Health. We appreciate student workers who contributed to the data collection.
References Cited
Author notes
Subject Editor: Maria Diuk-Wasser