-
PDF
- Split View
-
Views
-
Cite
Cite
Guanglu Wang, Mengyuan Wang, Jinchu Yang, Qian Li, Nianqing Zhu, Lanxi Liu, Xianmei Hu, Xuepeng Yang, De novo Synthesis of 2-phenylethanol from Glucose by Metabolically Engineered Escherichia coli, Journal of Industrial Microbiology and Biotechnology, Volume 49, Issue 6, November 2022, kuac026, https://doi.org/10.1093/jimb/kuac026
- Share Icon Share
Abstract
2-Phenylethanol (2- PE) is an aromatic alcohol with wide applications, but there is still no efficient microbial cell factory for 2-PE based on Escherichia coli. In this study, we constructed a metabolically engineered E. coli capable of de novo synthesis of 2-PE from glucose. Firstly, the heterologous styrene-derived and Ehrlich pathways were individually constructed in an L-Phe producer. The results showed that the Ehrlich pathway was better suited to the host than the styrene-derived pathway, resulting in a higher 2-PE titer of ∼0.76 ± 0.02 g/L after 72 h of shake flask fermentation. Furthermore, the phenylacetic acid synthase encoded by feaB was deleted to decrease the consumption of 2-phenylacetaldehyde, and the 2-PE titer increased to 1.75 ± 0.08 g/L. As phosphoenolpyruvate (PEP) is an important precursor for L-Phe synthesis, both the crr and pykF genes were knocked out, leading to ∼35% increase of the 2-PE titer, which reached 2.36 ± 0.06 g/L. Finally, a plasmid-free engineered strain was constructed based on the Ehrlich pathway by integrating multiple ARO10 cassettes (encoding phenylpyruvate decarboxylases) and overexpressing the yjgB gene. The engineered strain produced 2.28 ± 0.20 g/L of 2-PE with a yield of 0.076 g/g glucose and productivity of 0.048 g/L/h. To our best knowledge, this is the highest titer and productivity ever reported for the de novo synthesis of 2-PE in E. coli. In a 5-L fermenter, the 2-PE titer reached 2.15 g/L after 32 h of fermentation, suggesting that the strain has the potential to efficiently produce higher 2-PE titers following further fermentation optimization.
Introduction
Due to its pleasant rose-like odor and antibacterial properties, 2-phenylethanol (2-PE) is a high-value compound widely used in the cosmetic, food, and flavor industries (Zhan et al., 2022). Various wild-type yeasts, including Saccharomyces cerevisiae, Kluyveromyces marxianus, and Yarrowia lipolytica have the capacity of de novo synthesis of 2-PE (Etschmann et al., 2002; Wang et al., 2019; Zhang et al., 2014). Three biosynthesis pathways for 2-PE production have been reported to date, including the Ehrlich pathway (Gu et al., 2020), styrene-derived pathway (Machas et al., 2017) and phenylethylamine pathway (Masuo et al., 2015). Since these pathways start from L-phenylalanine (L-Phe) or phenylpyruvate (PPA) from the aromatic amino acid biosynthesis pathway, which is subject to strict feedback regulation, the carbon flux from the central metabolic pool toward 2-PE is quite weak and supports only comparatively low 2-PE titers. Therefore, high 2-PE titers are usually achieved by the biotransformation of externally added L-Phe, which is a more effective approach but with a high cost. As L-Phe is a relatively costly feedstock, direct 2-PE production from cheaper, renewable biomass sugars represents an attractive alternative to L-Phe conversion.
With recent advances in metabolic engineering, 2-PE produced by microbial systems is a potentially sustainable alternative to fossil fuel-based production and isolation from native plants, which has attracted considerable interest (Kong et al., 2020; Li et al., 2021; Zhan et al., 2022; Zhan et al., 2020). In consideration of its rapid growth, clear genetic background and highly efficient genome editing, E. coli has been used as a versatile platform strain to produce various biochemicals by applying genetic engineering methods (Guo et al., 2020; Guo et al., 2022; Lai et al., 2022; Sheng et al., 2021; Zhang et al., 2022), including 2-PE (Guo et al., 2017; Kang et al., 2014; Wang et al., 2019). Although various efforts have been made to improve the synthetic ability of E. coli, the titer, yield and productivity were still less than satisfactory. In this study, we engineered E. coli W3110 for increased flux along the Ehrlich pathway and achieved the highest titer of 2-PE from glucose reported to date.
Materials and Methods
Strains and plasmids
Plasmid construction and cloning were performed in E. coli DH5α, using 100 mg/L ampicillin and/or 30 mg/L kanamycin for selection, where appropriate. The plasmids pREDCas9 and pGRB were obtained from the lab of Prof. Chen (Tianjin University). The L-Phe overproducer strain E. coli M4 was a gift from Prof. Xixian Xie (Tianjin University of Science and Technology). Table 1 lists the specific plasmids and strains used in this study.
Name . | Relevant genotype . | Source . |
---|---|---|
Plasmids | ||
pREDCas9 | SpeR, Cas9 and λ Red recombinase expression vector | (Li et al., 2015) |
pGRB | AmpR, gRNA expression vector | (Li et al., 2015) |
pGRB-yeeP-gRNA | yeeP-gRNA expression vector; Ampr | This study |
pGRB-gapC-gRNA | gapC-gRNA expression vector; Ampr | This study |
pGRB-yeeP-styABC | pGRB-yeeP-gRNA with styABC sequence; Ampr | This study |
pGRB-gapC-PAL2-FDC1 | pGRB-gapC-gRNA with PAL2 and FDC1 sequence; Ampr | This study |
pGRB-feaB-gRNA | gapC-gRNA expression vector; Ampr | This study |
pGRB-crr-gRNA | crr-gRNA expression vector; Ampr | This study |
pTrc99a | AmpR, trc promoter, cloning vector | Lab Stock |
pSTV28 | CmR, lac promoter, cloning vector | Lab Stock |
pWSK29 | CmR, lac promoter, cloning vector | Lab Stock |
pTrc99a-ARO10 | AmpR, trc promoter, ARO10 expression plasmid | This study |
pSTV28- ARO10 | CmR, lac promoter, ARO10 expression plasmid | This study |
pWSK29- ARO10 | CmR, lac promoter, ARO10 expression plasmid | This study |
pTrc99a- ARO10* | AmpR, trc promoter, codon optimized ARO10 expression plasmid | This study |
Strains | ||
E. coli DH5α | Standard cloning strain | Invitrogen |
E. coli W3110 | Wild type | Lab Stock |
Saccharomyces cerevisiae S288c | MATα SUC2 gal2 mal mel flo1 flo8-1 hap1 ho bio1 bio6 | Lab Stock |
E. coli M4 | E. coli W3110, ∆pheA, tyrR::PT7-pheA-tyrB, yghX::Ptrc-aroG, lacI::PxylA-T7 RNA polymerase | Lab Stock |
E. coli PE1 | E. coli M4; gapC::PT7-PAL2-FDC1 | This study |
E. coli PE2 | E. coli M4; gapC::PT7-PAL2-FDC1; yeeP::PT7-styAB-styC | This study |
E. coli PE3 | E. coli M4; pTrc99a-ARO10; | This study |
E. coli PE4 | E. coli M4; pSTV28-ARO10; | This study |
E. coli PE5 | E. coli M4; pWSK29-ARO10; | This study |
E. coli PE6 | E. coli M4; pTrc99a-ARO10*; | This study |
E. coli PE7 | E. coli M4; pTrc99a-ARO10; ΔfeaB; | This study |
E. coli PE8 | E. coli M4; pTrc99a-ARO10; ΔfeaB; Δcrr; | This study |
E. coli PE9 | E. coli M4; pTrc99a-ARO10; ΔfeaB; Δcrr; ΔpykF; | This study |
E. coli PE10 | E. coli M4; ΔfeaB; Δcrr; ΔpykF; yeeP::Ptrc-ARO10; | This study |
E. coli PE11 | E. coli M4; ΔfeaB; Δcrr; ΔpykF; yeeP::Ptrc-ARO10; ykgH::Ptrc-ARO10 | This study |
E. coli PE12 | E. coli M4; ΔfeaB; Δcrr; ΔpykF; yeeP::Ptrc-ARO10; ykgH::Ptrc-ARO10; ykgA::Ptrc-yjgB | This study |
Name . | Relevant genotype . | Source . |
---|---|---|
Plasmids | ||
pREDCas9 | SpeR, Cas9 and λ Red recombinase expression vector | (Li et al., 2015) |
pGRB | AmpR, gRNA expression vector | (Li et al., 2015) |
pGRB-yeeP-gRNA | yeeP-gRNA expression vector; Ampr | This study |
pGRB-gapC-gRNA | gapC-gRNA expression vector; Ampr | This study |
pGRB-yeeP-styABC | pGRB-yeeP-gRNA with styABC sequence; Ampr | This study |
pGRB-gapC-PAL2-FDC1 | pGRB-gapC-gRNA with PAL2 and FDC1 sequence; Ampr | This study |
pGRB-feaB-gRNA | gapC-gRNA expression vector; Ampr | This study |
pGRB-crr-gRNA | crr-gRNA expression vector; Ampr | This study |
pTrc99a | AmpR, trc promoter, cloning vector | Lab Stock |
pSTV28 | CmR, lac promoter, cloning vector | Lab Stock |
pWSK29 | CmR, lac promoter, cloning vector | Lab Stock |
pTrc99a-ARO10 | AmpR, trc promoter, ARO10 expression plasmid | This study |
pSTV28- ARO10 | CmR, lac promoter, ARO10 expression plasmid | This study |
pWSK29- ARO10 | CmR, lac promoter, ARO10 expression plasmid | This study |
pTrc99a- ARO10* | AmpR, trc promoter, codon optimized ARO10 expression plasmid | This study |
Strains | ||
E. coli DH5α | Standard cloning strain | Invitrogen |
E. coli W3110 | Wild type | Lab Stock |
Saccharomyces cerevisiae S288c | MATα SUC2 gal2 mal mel flo1 flo8-1 hap1 ho bio1 bio6 | Lab Stock |
E. coli M4 | E. coli W3110, ∆pheA, tyrR::PT7-pheA-tyrB, yghX::Ptrc-aroG, lacI::PxylA-T7 RNA polymerase | Lab Stock |
E. coli PE1 | E. coli M4; gapC::PT7-PAL2-FDC1 | This study |
E. coli PE2 | E. coli M4; gapC::PT7-PAL2-FDC1; yeeP::PT7-styAB-styC | This study |
E. coli PE3 | E. coli M4; pTrc99a-ARO10; | This study |
E. coli PE4 | E. coli M4; pSTV28-ARO10; | This study |
E. coli PE5 | E. coli M4; pWSK29-ARO10; | This study |
E. coli PE6 | E. coli M4; pTrc99a-ARO10*; | This study |
E. coli PE7 | E. coli M4; pTrc99a-ARO10; ΔfeaB; | This study |
E. coli PE8 | E. coli M4; pTrc99a-ARO10; ΔfeaB; Δcrr; | This study |
E. coli PE9 | E. coli M4; pTrc99a-ARO10; ΔfeaB; Δcrr; ΔpykF; | This study |
E. coli PE10 | E. coli M4; ΔfeaB; Δcrr; ΔpykF; yeeP::Ptrc-ARO10; | This study |
E. coli PE11 | E. coli M4; ΔfeaB; Δcrr; ΔpykF; yeeP::Ptrc-ARO10; ykgH::Ptrc-ARO10 | This study |
E. coli PE12 | E. coli M4; ΔfeaB; Δcrr; ΔpykF; yeeP::Ptrc-ARO10; ykgH::Ptrc-ARO10; ykgA::Ptrc-yjgB | This study |
Name . | Relevant genotype . | Source . |
---|---|---|
Plasmids | ||
pREDCas9 | SpeR, Cas9 and λ Red recombinase expression vector | (Li et al., 2015) |
pGRB | AmpR, gRNA expression vector | (Li et al., 2015) |
pGRB-yeeP-gRNA | yeeP-gRNA expression vector; Ampr | This study |
pGRB-gapC-gRNA | gapC-gRNA expression vector; Ampr | This study |
pGRB-yeeP-styABC | pGRB-yeeP-gRNA with styABC sequence; Ampr | This study |
pGRB-gapC-PAL2-FDC1 | pGRB-gapC-gRNA with PAL2 and FDC1 sequence; Ampr | This study |
pGRB-feaB-gRNA | gapC-gRNA expression vector; Ampr | This study |
pGRB-crr-gRNA | crr-gRNA expression vector; Ampr | This study |
pTrc99a | AmpR, trc promoter, cloning vector | Lab Stock |
pSTV28 | CmR, lac promoter, cloning vector | Lab Stock |
pWSK29 | CmR, lac promoter, cloning vector | Lab Stock |
pTrc99a-ARO10 | AmpR, trc promoter, ARO10 expression plasmid | This study |
pSTV28- ARO10 | CmR, lac promoter, ARO10 expression plasmid | This study |
pWSK29- ARO10 | CmR, lac promoter, ARO10 expression plasmid | This study |
pTrc99a- ARO10* | AmpR, trc promoter, codon optimized ARO10 expression plasmid | This study |
Strains | ||
E. coli DH5α | Standard cloning strain | Invitrogen |
E. coli W3110 | Wild type | Lab Stock |
Saccharomyces cerevisiae S288c | MATα SUC2 gal2 mal mel flo1 flo8-1 hap1 ho bio1 bio6 | Lab Stock |
E. coli M4 | E. coli W3110, ∆pheA, tyrR::PT7-pheA-tyrB, yghX::Ptrc-aroG, lacI::PxylA-T7 RNA polymerase | Lab Stock |
E. coli PE1 | E. coli M4; gapC::PT7-PAL2-FDC1 | This study |
E. coli PE2 | E. coli M4; gapC::PT7-PAL2-FDC1; yeeP::PT7-styAB-styC | This study |
E. coli PE3 | E. coli M4; pTrc99a-ARO10; | This study |
E. coli PE4 | E. coli M4; pSTV28-ARO10; | This study |
E. coli PE5 | E. coli M4; pWSK29-ARO10; | This study |
E. coli PE6 | E. coli M4; pTrc99a-ARO10*; | This study |
E. coli PE7 | E. coli M4; pTrc99a-ARO10; ΔfeaB; | This study |
E. coli PE8 | E. coli M4; pTrc99a-ARO10; ΔfeaB; Δcrr; | This study |
E. coli PE9 | E. coli M4; pTrc99a-ARO10; ΔfeaB; Δcrr; ΔpykF; | This study |
E. coli PE10 | E. coli M4; ΔfeaB; Δcrr; ΔpykF; yeeP::Ptrc-ARO10; | This study |
E. coli PE11 | E. coli M4; ΔfeaB; Δcrr; ΔpykF; yeeP::Ptrc-ARO10; ykgH::Ptrc-ARO10 | This study |
E. coli PE12 | E. coli M4; ΔfeaB; Δcrr; ΔpykF; yeeP::Ptrc-ARO10; ykgH::Ptrc-ARO10; ykgA::Ptrc-yjgB | This study |
Name . | Relevant genotype . | Source . |
---|---|---|
Plasmids | ||
pREDCas9 | SpeR, Cas9 and λ Red recombinase expression vector | (Li et al., 2015) |
pGRB | AmpR, gRNA expression vector | (Li et al., 2015) |
pGRB-yeeP-gRNA | yeeP-gRNA expression vector; Ampr | This study |
pGRB-gapC-gRNA | gapC-gRNA expression vector; Ampr | This study |
pGRB-yeeP-styABC | pGRB-yeeP-gRNA with styABC sequence; Ampr | This study |
pGRB-gapC-PAL2-FDC1 | pGRB-gapC-gRNA with PAL2 and FDC1 sequence; Ampr | This study |
pGRB-feaB-gRNA | gapC-gRNA expression vector; Ampr | This study |
pGRB-crr-gRNA | crr-gRNA expression vector; Ampr | This study |
pTrc99a | AmpR, trc promoter, cloning vector | Lab Stock |
pSTV28 | CmR, lac promoter, cloning vector | Lab Stock |
pWSK29 | CmR, lac promoter, cloning vector | Lab Stock |
pTrc99a-ARO10 | AmpR, trc promoter, ARO10 expression plasmid | This study |
pSTV28- ARO10 | CmR, lac promoter, ARO10 expression plasmid | This study |
pWSK29- ARO10 | CmR, lac promoter, ARO10 expression plasmid | This study |
pTrc99a- ARO10* | AmpR, trc promoter, codon optimized ARO10 expression plasmid | This study |
Strains | ||
E. coli DH5α | Standard cloning strain | Invitrogen |
E. coli W3110 | Wild type | Lab Stock |
Saccharomyces cerevisiae S288c | MATα SUC2 gal2 mal mel flo1 flo8-1 hap1 ho bio1 bio6 | Lab Stock |
E. coli M4 | E. coli W3110, ∆pheA, tyrR::PT7-pheA-tyrB, yghX::Ptrc-aroG, lacI::PxylA-T7 RNA polymerase | Lab Stock |
E. coli PE1 | E. coli M4; gapC::PT7-PAL2-FDC1 | This study |
E. coli PE2 | E. coli M4; gapC::PT7-PAL2-FDC1; yeeP::PT7-styAB-styC | This study |
E. coli PE3 | E. coli M4; pTrc99a-ARO10; | This study |
E. coli PE4 | E. coli M4; pSTV28-ARO10; | This study |
E. coli PE5 | E. coli M4; pWSK29-ARO10; | This study |
E. coli PE6 | E. coli M4; pTrc99a-ARO10*; | This study |
E. coli PE7 | E. coli M4; pTrc99a-ARO10; ΔfeaB; | This study |
E. coli PE8 | E. coli M4; pTrc99a-ARO10; ΔfeaB; Δcrr; | This study |
E. coli PE9 | E. coli M4; pTrc99a-ARO10; ΔfeaB; Δcrr; ΔpykF; | This study |
E. coli PE10 | E. coli M4; ΔfeaB; Δcrr; ΔpykF; yeeP::Ptrc-ARO10; | This study |
E. coli PE11 | E. coli M4; ΔfeaB; Δcrr; ΔpykF; yeeP::Ptrc-ARO10; ykgH::Ptrc-ARO10 | This study |
E. coli PE12 | E. coli M4; ΔfeaB; Δcrr; ΔpykF; yeeP::Ptrc-ARO10; ykgH::Ptrc-ARO10; ykgA::Ptrc-yjgB | This study |
Plasmid construction and chromosomal manipulation
The recombinant DNA manipulation was performed as previously described (Sambrook & Russell, 2001). The primers used in this study are shown in Table S1. The sequence of the target gene, which was codon optimized for E. coli using GeneOptimizer software, was synthesized by GENEWIZ Bio Inc. (Suzhou, China). For plasmid construction, the complete gene sequence was cloned into the vector using PrimeSTAR Max DNA Polymerase (2×) (TaKaRa, Japan) by POE-PCR (You & Zhang, 2014). The PCR products were then electroporated into E. coli DH5α competent cells after being digested with DpnI (TaKaRa, Japan). Positive colonies were identified by PCR and DNA sequencing (Genewiz, Suzhou, China).
According to the reported chromosomal deletion and recombination strategy based on CRISPR/Cas9 technology (Wu et al., 2018; Xiong et al., 2021), we describe our experimental procedures using the deletion of the feaB gene as an example. We first obtained the 20-bp spacer sequences using the CRISPR RGEN Tool (http://www.rgenome.net/), conducted the PCR reaction using plasmid pGRB, primers pGRB-feaB-F, and pGRB-gRNA-R, and PrimeSTAR Max DNA Polymerase (2×). We then electroporated the DpnI-digested PCR fragments into E. coli DH5α competent cells. Finally, we identified the positive pGRB-feaB colonies by conducting a PCR test and DNA sequencing. For gene deletion, overlap-extension PCR was used to ligate the up- and down-stream homologous arms of feaB, amplified with feaB-F-F/R and feaB-B-F/R primer pairs, to generate DNA-feaB as the donor. For integration, the plasmids pREDCas9 were chemically transformed into E. coli W3110 competent cells, and then the transformants were cultured in an LB medium containing 50 μg/mL spectinomycin at 37°C. 0.1 mM IPTG was added when OD600 = 0.1–0.2 to induce the expression of λ red recombinase, and the cells were harvested when OD600 = 0.6–0.7. Subsequently, 200 ng DNA-feaB and 100 ng pGRB-feaB plasmids were both introduced into the electrocompetent cells of E. coli W3110 cells containing pREDCas9 using an Eppendorf Eporator (Germany) at 1.85 kV. After recovering in 1.0 mL SOC medium for 2 h at 32°C, the transformants were spread on LB agar plates containing 50 μg/mL ampicillin and 50 μg/mL spectinomycin for overnight culture at 32°C. Colony PCR detected positive clones were further verified by Sanger sequencing, and then cultured in LB medium with 0.2% L-arabinose (32°C) to eliminate pGRB. Finally, pREDCas9 was eliminated by culturing at 42°C.
Fermentation
For shake-flask fermentation, the transformed strains were cultured in 30 mL of seed-culture medium (Xiong et al., 2021) for 10 h in a shake flask (37°C, 220 rpm shaking). Then, 3 mL seed culture was diluted into 27 mL fermentation medium (Xiong et al., 2021) and cultivated for 24 h at 37°C with 220 rpm shaking. The fermentation process was maintained by adding 1.0 mL of 60% glucose solution when the concentration of glucose fell below 2.0 g/L. For fed-batch fermentation, the cells were pre-cultivated on agar slants for 12 h at 37°C and then transferred into a 5-L bioreactor to finish the fermentation process (pH 7.0, 37°C, and > 20% dissolved oxygen).
qRT-PCR
Total RNA was extracted from fresh cells during the exponential growth phase using a RNAprep Pure Cell/Bacteria Kit (Tiangen, China). The RNA was reverse-transcribed into cDNA using a FastQuant RT Kit (Tiangen, China) with gDNase and random primers. The total reaction volume of qRT-PCR was 20 μL, including 100 ng of cDNA and 0.25 mmol/L of each primer (See Supplementary Table S1), and was performed with Real Master Mix (SYBR Green) on a Light Cycler® 480 II (Roche, Switzerland) according to the manufacturer's instructions. The fold change of each transcript was measured by comparing it to the control, normalized to the rrsA gene as the internal control, and calculated using the comparative CT method (Livak & Schmittgen, 2001; Wang et al., 2018). The data represent the means from three biological replicates.
Analytical methods
Cell growth was examined at OD600 via a UV spectrophotometer. The concentrations of 2-PE, L-Phe, and 2-phenylacetic acid were determined by HPLC (Agilent 1260) at 260 nm with a column temperature of 30°C (Lu et al., 2016). A Silgreen-C18 AQ column (4.6 mm × 250 mm, 5 μm; Beijing Green Grass Technology Development Co., LTD) and an Agilent DAD detector were used. The methanol/water mixture (50:50 v/v) was used as the mobile phase which flow rate is 0.5 mL/min (Machas et al., 2017). And an SBA-40E biosensor (Shandong Province Academy of Sciences, China) was used to examine the glucose concentration.
Results
Initial assessment of a previously constructed strain for L-Phe biosynthesis
Genetic modifications of several key genes involved in L-Phe biosynthesis pathway in E. coli W3110 were introduced using metabolic engineering strategies, including elimination of the feedback repressor TyrR and improving the expression of genes encoding key enzymes (such as aroG, pheA, etc.) in the L-Phe biosynthesis pathway (Fig. 1). The T7 RNA polymerase gene was amplified from E. coli BL21(DE3) by PCR, placed under the control of the PxylA promoter, and integrated at the lacI locus in the genome. To remove feedback inhibition of key rate-limiting enzymes, the pheA gene (encoding chorismite mutase/prephenate dehydratase) in the native locus was deleted and another pheA gene and tyrB gene (encoding tyrosine aminotransferase) were combined into a single artificial operon controlled by the T7 promoter and integrated into the tyrR locus. In addition, a mutant aroGS180F (encoding a 3-deoxy-D-arabino-heptulosonate-7-phosphate (DAHP) synthase variant resistant to feedback inhibition by L-Phe) controlled by the trc promoter was integrated into the yghX pseudogene locus (Goormans et al., 2020), resulting in an engineering strain designated as E. coli M4 (E. coli W3110; ∆pheA; tyrR:: PT7-pheA-tyrB; yghX:: Ptrc-aroG; lacI:: PxylA-T7 RNA polymerase). Consequently, the L-Phe titer of E. coli M4 reached about 3.12 ± 0.18 g/L in shake-flask fermentation and up to 11.71 g/L in a 5-L bioreactor fermentation with high dissolved oxygen. This suggested that E. coli M4 could be a good platform for 2-PE production.
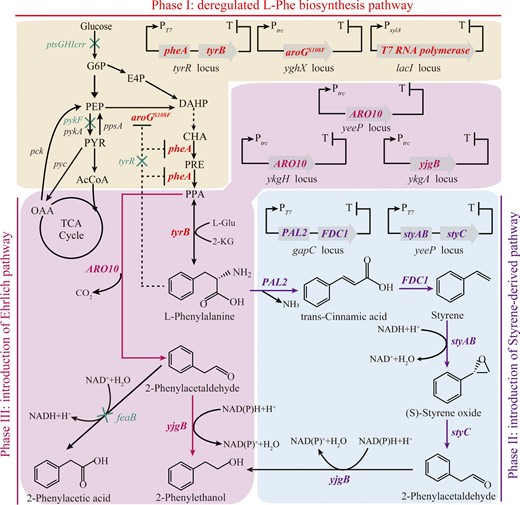
Rational metabolic engineering strategy for de novo synthesis of 2-PE in E. coli. Native enzymatic pathways are indicated with black arrows. The deregulated L-Phe biosynthesis pathway is indicated in red. The heterologous Ehrlich pathway is indicated in magenta and the styrene-derived pathway is indicated in purple. Overexpressed genes are indicated in bold and highlighted with colors. Downregulated or deleted genes are indicated with green crosses. G6P, glucose 6-p; E4P, erythrose 4-p; PEP, phosphoenolpyruvate; PYR, pyruvate; AcCoA, acetyl coenzyme A; OAA, oxaloacetate; DAHP, 3-deoxy-D-arabinoheptulosonate 7-p; CHA, chorismate; PRE, prephenate; PPA, phenylpyruvate;
Introduction of a heterologous styrene-derived pathway for 2-PE biosynthesis
Wu et al. reported a multienzyme cascade biocatalysis strategy for converting styrene into 2-PE with a particularly high conversion (>99%) by using styrene monooxygenase (to convert styrene to S-styrene oxide), styrene oxide isomerase (to convert styrene oxide to phenylacetaldehyde) and phenylacetaldehyde reductase (to convert phenylacetaldehyde to 2-PE) (Wu et al., 2017). A 2-PE biosynthesis pathway, derived from styrene, was constructed based on the extension of the styrene biosynthesis pathway. This process includes five steps starting from endogenous L-Phe (Machas et al., 2017). The thermodynamic driving force of the styrene-derived pathway was approximately 10 times greater than that of the Ehrlich pathway, and it was considered a better 2-PE biosynthesis route with high stability and efficiency in E. coli (Machas et al., 2017; Wu et al., 2017). This pathway is encoded by four heterologous genes, including PAL2 (from Arabidopsis thaliana, encoding phenylalanine ammonia-lyase), FDC1 (from Saccharomyces cerevisiae, encoding phenylacrylic acid decarboxylase), and styAB (from Pseudomonas putida S12, encoding styrene monooxygenase). It converts L-Phe to (S)-styrene oxide, after which styC-encoded styrene oxide isomerase (from Pseudomonas putida S12) converts (S)-phenylene oxide to phenylacetaldehyde. Finally, native NADPH-dependent alcohol dehydrogenases reduce phenylacetaldehyde into 2-PE (Fig. 1).
Therefore, we attempted to assess the styrene-derived pathway in E. coli M4. Four heterologous genes PAL2 (GenBank: AAM12956.1), FDC1(GenBank: DAA12368.1), styAB (GenBank: AJA17113.1 and AJA17114.1) and styC (GenBank: AJA17115.1) were codon-optimized for E. coli and synthesized. The corresponding coding sequences were placed under the control of the strong PT7 promoter and integrated into the chromosome of E. coli M4, resulting in the strains E. coli PE1 (E. coli M4, gapC:: PT7-PAL2-FDC1) and E. coli PE2 (E. coli M4, gapC:: PT7-PAL2-FDC1, yeeP:: PT7-styAB-styC). Compared with the parent strain, the L-Phe titer of E. coli PE2 decreased in shake flask after 72 h of fermentation, from 3.12 ± 0.18 g/L to 2.18 ± 0.22 g/L (Supplementary information, Figure S1). Surprisingly, only trace amounts of 2-PE were detected in PE2. RT-PCR results confirmed that all four genes were successfully overexpressed in the cells (Supplementary information, Figure S2). Taken together, the results indicate that the enzyme activity obtained by placing the gene under the control of a strong promoter was unsatisfactory, probably due to abnormal folding caused by excessively fast transcription.
Introduction of a heterologous ehrlich pathway for 2-PE biosynthesis
We attempted to introduction the Ehrlich pathway, which was relatively simple. The Ehrlich pathway consists of three reactions. A transaminase first converts L-Phe into phenylpyruvate, which is subsequently converted into phenylethylaldehyde and 2-PE by phenylpyruvate decarboxylase and alcohol dehydrogenase, respectively (Qian et al., 2019). The decarboxylation reactions can be catalyzed by many enzymes, such as those encoded by pcd, kind, ipdC and ARO10 (Wang et al., 2019). We employed ARO10 from S. cerevisiae S288c, which encodes a phenylpyruvate decarboxylase with relatively high activity (Yin et al., 2015). In the beginning, three plasmids with different copy number were selected as backbone (high copy number pTrc99a, medium copy number pSTV28 and low copy number pWSK29), while the original ARO10 and optimized ARO10* sequences were cloned by POE-PCR (Supplementary information, Figure S3). The plasmids were then introduced into E. coli M4 resulting in E. coli PE3 (E. coli M4; pTrc99a-ARO10), PE4 (E. coli M4; pSTV28-ARO10), PE5 (E. coli M4; pWSK29-ARO10) and PE6 (E. coli M4; pTrc99a-ARO10*).
As shown in Fig. 2, E. coli PE3 with the high copy number plasmid produced the highest 2-PE titer of 0.76 ± 0.02 g/L after 72 h of fermentation. This suggested that strong overexpression of phenylpyruvate decarboxylase plays a crucial role in 2-PE production. By contrast, the 2-PE titer decreased significantly in E. coli PE6 (much lower than PE3), we speculated that the optimized gene might lead to mRNA instability or abnormal folding, which finally resulted in low enzymatic activity of phenylpyruvate decarboxylate. Consequently, the engineered strain E. coli PE3 was chosen as the starting strain for further optimization.
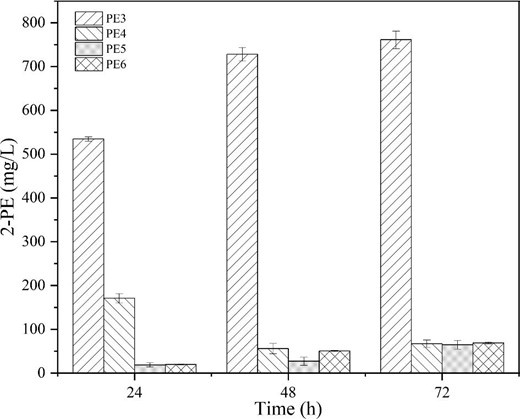
Initial assessment of using the Ehrlich pathway to produce 2-PE from glucose.
Improving 2-PE production by eliminating the by-product phenylacetic acid
In addition to 2-PE, the strains generated the byproduct 2-phenylacetic acid, with a final titer reaching 0.84 ± 0.02 g/L in E. coli PE3. In fact, the native NAD+-dependent phenylacetic acid synthase encoded by feaB converts 2-phenylacetaldehyde into 2-phenylacetic acid. Therefore, deletion of feaB could eliminate the undesirable accumulation of 2-phenylacetic acid and further increase 2-PE production. We successfully knocked out feaB in E. coli PE3 and obtained E. coli PE7 (E. coli M4; ΔfeaB; pTrc99a-ARO10). As previously reported (Machas et al., 2017), 2-phenylacetic acid production was no longer detected after this deletion, and the 2-PE titer also exhibited a great improvement. As shown in Fig. 3A, the 2-PE titer of E. coli PE7 reached 1.04 ± 0.34 g/L after 72 h of fermentation, representing a 35% increase over E. coli PE3. To further improve the 2-PE production, E. coli PE7 was induced with different concentrations IPTG ranging from 0.25 to 1.5 mmol/L. As shown in Fig. 3B, the highest production of 2-PE was observed in the fermentation induced with 0.5 mmol/L IPTG. The production of 2-PE reached 1.75 ± 0.08 g/L after 72 h.
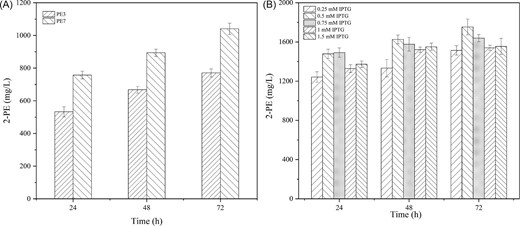
2-PE titers of engineered strains E. coli PE3 and E. coli PE7 under different IPTG induction concentrations. (A) 0.1 mmol/L IPTG; (B) 0.25 to 1.5 mmol/L IPTG.
Increasing the precursor supply to improve 2-PE biosynthesis
Robust 2-PE production depends on ample production of the SA (shikimic acid) pathway precursors (Fig. 1), which in turn is known to benefit from increased availability of phosphoenolpyruvate (PEP). Consequently, improving PEP production or blocking its consumption could further increase 2-PE production (Guo et al., 2022). Indeed, pykF (encoding pyruvate dehydrogenase), which converts PEP into pyruvate, is helpful for improving PEP availability and reducing the acetate yield (Noda et al., 2016). In addition, it was further confirmed that partial inactivation of the glucose-specific phosphotransferase system (PTS) can increase the PEP availability, which facilitates glucose uptake via its phosphorylation at the expense of PEP, as can be achieved by deleting crr (encoding IIAGlc, part of the PTSGHIcrr operon) (Gosset, 2005). Accordingly, the engineered strains E. coli PE8 (E. coli M4; ΔfeaB; Δcrr; pTrc99a-ARO10) and E. coli PE9 (E. coli M4; ΔfeaB; Δcrr; ΔpykF; pTrc99a-ARO10) were constructed by deleting crr or pykF. As shown in Fig. 4, the results indicated that deletion of the two genes had a significant effect on 2-PE production. The 2-PE titer of E. coli PE8 reached 2.12 ± 0.02 g/L and that of PE9 reached 2.36 ± 0.06 g/L under induction with 0.5 mmol/L IPTG, which represent improvements by about 21 and 35%, respectively. Notably, this is the highest 2-PE titer reported to date for de novo synthesis by expressing heterologous Ehrlich pathway in E. coli.
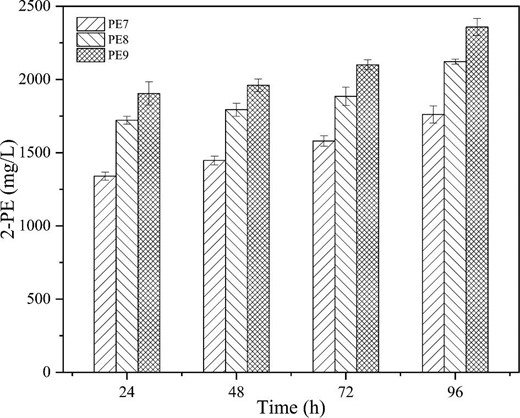
2-PE titers of engineered strains E. coli PE7, PE8, and PE9 under 0.5 mmol/L IPTG induction.
Improving 2-PE tolerance by introducing the LexA_E45I mutation and adaptive laboratory evolution
Considering that the 2-PE titer of E. coli PE8 and E. coli PE9 reached over 2.0 g/L, we were highly aware of the problem of 2-PE toxicity to E. coli, which greatly limited the 2-PE titer. Liang et al. applied an iCREATE strategy to design, construct, and test a library of transcriptional regulators targeting 54 genes with 85 420 mutations for increased styrene resistance and production in E. coli. The best mutant, ST05 LexA_E45I, not only exhibited improved styrene tolerance but also produced a 3.45-fold higher styrene concentration than the parental strain (Liang et al., 2020). Therefore, we attempted to introduce the LexA_E45I mutation into E. coli PE3 to improve its 2-PE tolerance. The experimental results indicated that the LexA_E45I mutation improved the 2-PE tolerance to a certain extent, but the strain nearly completely lost its ability to produce 2-PE (data not shown). This finding suggests that the 2-PE tolerance mechanism is different from that of styrene tolerance. In addition, we used the classical tool of adaptive laboratory evolution to improve the 2-PE tolerance. Using E. coli PE8 as the starting strain, 90 days of continuous culture were carried out with increasing 2-PE concentrations (0.5, 1.0, 1.5 to 2.0 g/L, and each concentration was applied for 30 days). It is important to note that the evolved strain grew well in the presence of 2.0 g/L of 2-PE, but still almost completely loses its capacity for 2-PE synthesis. These results indicate that 2-PE tolerance and 2-PE biosynthesis are conflicting evolutionary objectives. As a consequence of our failed attempts, improving the 2-PE tolerance of E. coli by adaptive laboratory evolution still demands more elaborate directed evolution strategies, such as adding both 2-PE and p-fluorophenylalanine (structural analog of L-Phe) to the medium, which might improve its 2-PE biosynthesis ability while obtaining higher 2-PE tolerance. However, this hypothetical strategy needs to be further tested in future studies.
Engineering of a plasmid-free strain for de novo synthesis of 2-PE based on the ehrlich pathway
After confirming its feasibility using plasmids, we attempted to construct an engineered strain that avoids the use of plasmids and antibiotics, resulting in the chromosomally engineered strain E. coli PE10 (E. coli M4, ΔfeaB; Δcrr; ΔpykF; yeeP:: Ptrc-ARO10). As shown in Fig. 5, the available glucose was almost completely consumed after 36 h of fermentation, at which point the titer of 2-PE reached 1.61 ± 0.13 g/L, corresponding to a yield of 0.054 g/g glucose and productivity of 0.048 g/L/h. Given that the over-expression of ARO10 is critical for 2-PE production, it is likely that the integration of ARO10 at a single locus is insufficient for 2-PE biosynthesis. Accordingly, we integrated an additional copy of ARO10 into the genome of E. coli PE10 at the ykgH locus (Goormans et al., 2020) to obtain E. coli PE11 (E. coli M4, ΔfeaB; Δcrr; ΔpykF; yeeP:: Ptrc-ARO10; ykgH:: Ptrc-ARO10). YjgB is a known aldehyde reductase of E. coli that can accept a broad range of various aldehydes as substrates to produce the corresponding alcohols, and its overexpression was reported to improve the biosynthesis of 2-PE (Guo et al., 2017). In addition, an additional copy of yjgB was integrated into the genome, resulting in E. coli PE12 (E. coli M4, ΔfeaB, Δcrr, ΔpykF; yeeP:: Ptrc-ARO10; ykgH:: Ptrc-ARO10; ykgA:: Ptrc-yjgB). The engineered strain grew well, with the maximum OD600 reaching about 25 during shake flasks fermentation, and produced the highest de novo 2-PE titer from glucose in E. coli reported to date. Under the optimal conditions, the engineered strain PE12 produced 2.28 ± 0.20 g/L of 2-PE with a yield and productivity of 0.076 g/g glucose and 0.048 g/L/h, respectively. E. coli PE12 produced slightly less 2-PE than E. coli PE9, which we speculate may be caused by lower levels of ARO10 expression.

L-Phe, 2-PE titers and growth curve of engineered strains E. coli PE10, E. coli PE11, and E. coli PE12.
Fed-batch production of 2-PE in a 5-L bioreactor
Based on previous reports that an adequately high dissolved oxygen level is critical for L-Phe biosynthesis, we conducted a fed-batch L-Phe fermentation with an adequate dissolved oxygen level and continuous sugar supplementation. The OD600 of E. coli PE12 reached approximately 54, which was 2.13 times higher than in shake-flask fermentation. L-Phe was continuously accumulated and the final titer reached 11.71 g/L. However, the titer of 2-PE only reached 0.11 g/L, which was much lower than in shake-flask fermentation. We speculated that continuous glucose supplementation and the high dissolved oxygen level significantly increased the biomass and L-Phe titer, but it was clearly not conducive to the de novo synthesis of 2-PE. To balance the cell growth, L-Phe supply and 2-PE biosynthesis, we investigated constant stirring speeds of 200, 400, 600, 800 r/min, while the glucose concentration was maintained below 5.0 g/L. The highest 2-PE titer was obtained at 400 r/min, as shown in Fig. 6. The OD600 reached ∼20 and the L-Phe titer reached 1.0 g/L after 60 h. The synthesis of 2-PE began already at 4 h, and the titer continuously increased in the first half of the fermentation process, reaching a maximum of 2.15 g/L at 32 h. Although the time to reach the maximal titer was cut in half, the 2-PE titer we obtained in the bioreactor was still not satisfactory (notably lower than previous studies using S. cerevisiae or Bacillus licheniformis), and requires additional research efforts in the future.
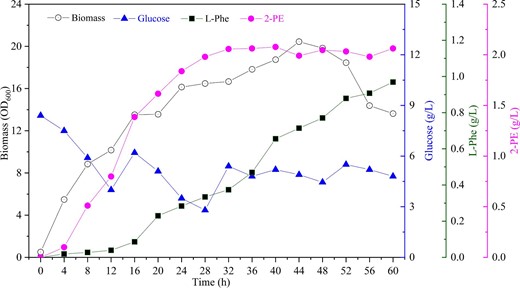
Fed-batch fermentation of recombinant E. coli PE12 in a 5-L bioreactor. OD600 (black circle), glucose concentration (blue triangle), L-Phe titer (black square), and 2-PE titer (magenta circle), respectively.
Discussion
The increasing demand for 2-PE has inspired great interest in its biotechnological production. Many studies have successfully implemented the synthesis of 2-PE in microbial strains expressing a heterologous Erich pathway, phenylacetaldehyde synthesis pathway, or styrene derivative pathway (Table 2). In this study, an Ehrlich pathway was successfully introduced into E. coli to de novo synthesize 2-PE from glucose and the titer was further improved by metabolic engineering. The plasmid-free chromosomally engineered strain E. coli PE12 was able to produce 2-PE from glucose and the culture process no longer requires antibiotics. In addition, a 5-L laboratory-scale fermentation of the best strain was preliminarily explored. Under 5-L fermentation conditions, 2-PE biosynthesis started already at 4 h, and the titer continuously increased in the first half of the fermentation process, reaching a maximum of 2.15 g/L at 32 h. Notably, this is the highest titer reported for the de novo synthesis of 2-PE by E. coli to date.
2-PE production in rationally engineered strains using glucose as substrate
Microorganism . | Characteristics . | Carbon source (g/L) . | Titer (g/L) . | Cultivation mode . | Reference . |
---|---|---|---|---|---|
Bacillus licheniformis PE23 | DWc9n-xkdG::yugJ, Δpyk; pHY-kivD-aroD* | glucose (20) | 6.24 | Shake flask | (Zhan et al., 2022) |
Pichia pastoris SK004 | ARO10; ADH6; ARO8; aroGfbr; pheAfbr | glucose (20) | 1.17 | Shake flask | (Kong et al., 2020) |
Kluyveromyces marxianus CBS 6556 | glucose (20) | 1.94 | Shake flask | (Li et al., 2021) | |
K. marxianus BY25569 | aro10; adh2; aroGfbr | glucose (20) | 1.3 | Shake flask | (Kim et al., 2014) |
Yarrowia lipolytica po1fk7P | po1fk4 − 2 ΔylDGA2 ΔylDG-A1::loxP harboring pYLXP′-citB-ylIDP2-ylODC | glucose (40) | 2.67 | Shake flask | (Gu et al., 2020) |
Saccharomyces cerevisiae BY4741 | aro10; ∆aro8 | glucose (20) | 0.10 | Shake flask | (Shen et al., 2016) |
S. cerevisiae BY4741 | aro4fbr; aro7fbr; ∆aro8; ∆tyr1; ∆aro3 | glucose (20) | 0.41 | Shake flask | (Romagnoli et al., 2015) |
Escherichia coli NST74 | PAL2; FDC1; styAB; styC; ∆feaB; ∆pykA; ∆pykF; ∆crr | glucose (50) | 1.94 | Shake flask | (Machas et al., 2017) |
E. coli DG02 | aroGfbr; pheAfbr; kdc; yjgB; aro8 | glucose (20) | 1.02 | Shake flask | (Guo et al., 2017) |
E. coli MG1655 | aro8; kdc; yjgB | glucose (20) | 0.18 | Shake flask | (Guo et al., 2017) |
Enterobacter sp. CGMCC 5087 | pheAfbr; DAHP | glucose (20) | 0.34 | Shake flask | (Zhang et al., 2014) |
E. coli BW25113(DE3) | ipdC; yahK; aroFfbr; pheAfbr; ΔfeaB | glucose (10) | 0.94 | Shake flask | (Koma et al., 2012) |
Microorganism . | Characteristics . | Carbon source (g/L) . | Titer (g/L) . | Cultivation mode . | Reference . |
---|---|---|---|---|---|
Bacillus licheniformis PE23 | DWc9n-xkdG::yugJ, Δpyk; pHY-kivD-aroD* | glucose (20) | 6.24 | Shake flask | (Zhan et al., 2022) |
Pichia pastoris SK004 | ARO10; ADH6; ARO8; aroGfbr; pheAfbr | glucose (20) | 1.17 | Shake flask | (Kong et al., 2020) |
Kluyveromyces marxianus CBS 6556 | glucose (20) | 1.94 | Shake flask | (Li et al., 2021) | |
K. marxianus BY25569 | aro10; adh2; aroGfbr | glucose (20) | 1.3 | Shake flask | (Kim et al., 2014) |
Yarrowia lipolytica po1fk7P | po1fk4 − 2 ΔylDGA2 ΔylDG-A1::loxP harboring pYLXP′-citB-ylIDP2-ylODC | glucose (40) | 2.67 | Shake flask | (Gu et al., 2020) |
Saccharomyces cerevisiae BY4741 | aro10; ∆aro8 | glucose (20) | 0.10 | Shake flask | (Shen et al., 2016) |
S. cerevisiae BY4741 | aro4fbr; aro7fbr; ∆aro8; ∆tyr1; ∆aro3 | glucose (20) | 0.41 | Shake flask | (Romagnoli et al., 2015) |
Escherichia coli NST74 | PAL2; FDC1; styAB; styC; ∆feaB; ∆pykA; ∆pykF; ∆crr | glucose (50) | 1.94 | Shake flask | (Machas et al., 2017) |
E. coli DG02 | aroGfbr; pheAfbr; kdc; yjgB; aro8 | glucose (20) | 1.02 | Shake flask | (Guo et al., 2017) |
E. coli MG1655 | aro8; kdc; yjgB | glucose (20) | 0.18 | Shake flask | (Guo et al., 2017) |
Enterobacter sp. CGMCC 5087 | pheAfbr; DAHP | glucose (20) | 0.34 | Shake flask | (Zhang et al., 2014) |
E. coli BW25113(DE3) | ipdC; yahK; aroFfbr; pheAfbr; ΔfeaB | glucose (10) | 0.94 | Shake flask | (Koma et al., 2012) |
2-PE production in rationally engineered strains using glucose as substrate
Microorganism . | Characteristics . | Carbon source (g/L) . | Titer (g/L) . | Cultivation mode . | Reference . |
---|---|---|---|---|---|
Bacillus licheniformis PE23 | DWc9n-xkdG::yugJ, Δpyk; pHY-kivD-aroD* | glucose (20) | 6.24 | Shake flask | (Zhan et al., 2022) |
Pichia pastoris SK004 | ARO10; ADH6; ARO8; aroGfbr; pheAfbr | glucose (20) | 1.17 | Shake flask | (Kong et al., 2020) |
Kluyveromyces marxianus CBS 6556 | glucose (20) | 1.94 | Shake flask | (Li et al., 2021) | |
K. marxianus BY25569 | aro10; adh2; aroGfbr | glucose (20) | 1.3 | Shake flask | (Kim et al., 2014) |
Yarrowia lipolytica po1fk7P | po1fk4 − 2 ΔylDGA2 ΔylDG-A1::loxP harboring pYLXP′-citB-ylIDP2-ylODC | glucose (40) | 2.67 | Shake flask | (Gu et al., 2020) |
Saccharomyces cerevisiae BY4741 | aro10; ∆aro8 | glucose (20) | 0.10 | Shake flask | (Shen et al., 2016) |
S. cerevisiae BY4741 | aro4fbr; aro7fbr; ∆aro8; ∆tyr1; ∆aro3 | glucose (20) | 0.41 | Shake flask | (Romagnoli et al., 2015) |
Escherichia coli NST74 | PAL2; FDC1; styAB; styC; ∆feaB; ∆pykA; ∆pykF; ∆crr | glucose (50) | 1.94 | Shake flask | (Machas et al., 2017) |
E. coli DG02 | aroGfbr; pheAfbr; kdc; yjgB; aro8 | glucose (20) | 1.02 | Shake flask | (Guo et al., 2017) |
E. coli MG1655 | aro8; kdc; yjgB | glucose (20) | 0.18 | Shake flask | (Guo et al., 2017) |
Enterobacter sp. CGMCC 5087 | pheAfbr; DAHP | glucose (20) | 0.34 | Shake flask | (Zhang et al., 2014) |
E. coli BW25113(DE3) | ipdC; yahK; aroFfbr; pheAfbr; ΔfeaB | glucose (10) | 0.94 | Shake flask | (Koma et al., 2012) |
Microorganism . | Characteristics . | Carbon source (g/L) . | Titer (g/L) . | Cultivation mode . | Reference . |
---|---|---|---|---|---|
Bacillus licheniformis PE23 | DWc9n-xkdG::yugJ, Δpyk; pHY-kivD-aroD* | glucose (20) | 6.24 | Shake flask | (Zhan et al., 2022) |
Pichia pastoris SK004 | ARO10; ADH6; ARO8; aroGfbr; pheAfbr | glucose (20) | 1.17 | Shake flask | (Kong et al., 2020) |
Kluyveromyces marxianus CBS 6556 | glucose (20) | 1.94 | Shake flask | (Li et al., 2021) | |
K. marxianus BY25569 | aro10; adh2; aroGfbr | glucose (20) | 1.3 | Shake flask | (Kim et al., 2014) |
Yarrowia lipolytica po1fk7P | po1fk4 − 2 ΔylDGA2 ΔylDG-A1::loxP harboring pYLXP′-citB-ylIDP2-ylODC | glucose (40) | 2.67 | Shake flask | (Gu et al., 2020) |
Saccharomyces cerevisiae BY4741 | aro10; ∆aro8 | glucose (20) | 0.10 | Shake flask | (Shen et al., 2016) |
S. cerevisiae BY4741 | aro4fbr; aro7fbr; ∆aro8; ∆tyr1; ∆aro3 | glucose (20) | 0.41 | Shake flask | (Romagnoli et al., 2015) |
Escherichia coli NST74 | PAL2; FDC1; styAB; styC; ∆feaB; ∆pykA; ∆pykF; ∆crr | glucose (50) | 1.94 | Shake flask | (Machas et al., 2017) |
E. coli DG02 | aroGfbr; pheAfbr; kdc; yjgB; aro8 | glucose (20) | 1.02 | Shake flask | (Guo et al., 2017) |
E. coli MG1655 | aro8; kdc; yjgB | glucose (20) | 0.18 | Shake flask | (Guo et al., 2017) |
Enterobacter sp. CGMCC 5087 | pheAfbr; DAHP | glucose (20) | 0.34 | Shake flask | (Zhang et al., 2014) |
E. coli BW25113(DE3) | ipdC; yahK; aroFfbr; pheAfbr; ΔfeaB | glucose (10) | 0.94 | Shake flask | (Koma et al., 2012) |
As we summarized in Table 2, many studies have successfully implemented the synthesis of 2-PE in microbial strains. However, microbial production of heterologous organic compounds is challenging, as the biosynthetic pathways are often complex and produce metabolites that are toxic to the host. Although 2-PE production has been significantly improved through diverse engineering strategies, industrial production of 2-PE is still a challenge. In fact, compared with highly tolerant hosts (for example, Y. lipolytica or B. licheniformis), a serious disadvantage for 2-PE bioproduction in E. coli is its low tolerance to 2-PE, which greatly limited the 2-PE titer so far. New strategies to overcome the toxicity of 2-PE are expected to significantly advance the 2-PE titer and would further develop E. coli into a suitable platform for 2-PE biosynthesis. However, additional research in this direction is still needed in the future. This limitation has been partially addressed by strategies such as in situ product recovery (ISPR) (Hua & Xu, 2011; Wang et al., 2019). In terms of process engineering, in-situ separation technology is an effective means to improve the biosynthesis of 2-PE. Studies have applied this technology to continuously separate 2-PE from the fermentation broth, so that cells can grow normally and thereby increase the yield of 2-PE. Other approaches, such as pervaporation and solid-phase extraction (i.e. using hydrophobic resins) have resulted in up to 10-fold improvements of 2-PE production (Achmon et al., 2011). When S. cerevisiae Giv2009 was used to biosynthesize 2-PE, oleic acid was added to the fermentation broth, and the final yield of 2-PE reached 12.6 g/L in fed-batch fermentation (Stark et al., 2002). Hua et al. used PPG1500 as extractant to conduct supplementary fermentation with S. cerevisiae. The concentration of 2-PE in PPG1500 reached 22.0 g/L, and the total output was 7.5 g/L (Hua et al., 2013). Chreptowicz et al. reported that the yield of 2-PE extracted from the fermentation broth of S. cerevisiae with rapeseed oil was 9.79 g/L (Chreptowicz & Mierzejewska, 2018). While these methods considerably increased the titer of 2-PE, they result in higher production costs. Accordingly, future research studies should focus on improving the 2-PE titer by increasing its tolerance of E. coli to 2-PE.
Author contribution
Q. L. and G. W. designed the experiments and drafted the manuscript. M. W., N. Z., L. L., and X. H. carried out the experiments. J. Y. and X. Y. oversaw the project. Al l authors read and approved the final manuscript.
Funding
This work was supported by the Joint Funds of the National Natural Science Foundation of China (Grant No. U1904101) and the Key Research Projects of the Science and Technology Department of Henan Province (Nos. 202 102 310 021 and 182 102 310 607).
Conflicts of interest
The authors declare that they have no conflicts of interest related to the publication of this study.
Data availability
All experimental data and strains constructed in this study will be made available from the corresponding author upon reasonable request from readers.
Ethics approval
Not applicable
Consent to participate
Not applicable
Consent for publication
Not applicable
References
Author notes
These authors contributed equally to this work.