-
PDF
- Split View
-
Views
-
Cite
Cite
Sylwia Jezierska, Silke Claus, Rodrigo Ledesma-Amaro, Inge Van Bogaert, Redirecting the lipid metabolism of the yeast Starmerella bombicola from glycolipid to fatty acid production, Journal of Industrial Microbiology and Biotechnology, Volume 46, Issue 12, 1 December 2019, Pages 1697–1706, https://doi.org/10.1007/s10295-019-02234-x
- Share Icon Share
Abstract
Free fatty acids are basic oleochemicals implemented in a range of applications including surfactants, lubricants, paints, plastics, and cosmetics. Microbial fatty acid biosynthesis has gained much attention as it provides a sustainable alternative for petrol- and plant oil-derived chemicals. The yeast Starmerella bombicola is a microbial cell factory that naturally employs its powerful lipid metabolism for the production of the biodetergents sophorolipids (> 300 g/L). However, in this study we exploit the lipidic potential of S. bombicola and convert it from the glycolipid production platform into a free fatty acid cell factory. We used several metabolic engineering strategies to promote extracellular fatty acid accumulation which include blocking competing pathways (sophorolipid biosynthesis and β-oxidation) and preventing free fatty acid activation. The best producing mutant (Δcyp52m1Δfaa1Δmfe2) secreted 0.933 g/L (± 0.04) free fatty acids with a majority of C18:1 (43.8%) followed by C18:0 and C16:0 (40.0 and 13.2%, respectively). Interestingly, deletion of SbFaa1 in a strain still producing sophorolipids also resulted in 25% increased de novo sophorolipid synthesis (P = 0.0089) and when oil was supplemented to the same strain, a 50% increase in sophorolipid production was observed compared to the wild type (P = 0.03). We believe that our work is pivotal for the further development and exploration of S. bombicola as a platform for synthesis of environmentally friendly oleochemicals.
Electronic supplementary material
The online version of this article (10.1007/s10295-019-02234-x) contains supplementary material, which is available to authorized users.
Introduction
Fatty acids are essential building blocks of membrane lipids, energy and carbon storage and they participate in cell signaling [24]. In addition, free fatty acids are basic oleochemicals used in a wide range of applications including surfactants, lubricants, paints, surface coatings, plastics and cosmetics [18]; and thanks to the high energy potential, long chain fatty acids (LCFA) are ideal precursors for biofuels as well [1]. Due to the limited global supply of petroleum and energy security, microbial fatty acid biosynthesis has gained much attention as it provides a sustainable alternative for petrol- and plant oil- derived chemicals [15]. Numerous studies describe microbial cell factories for the production of fatty acid-derived oleochemicals including fatty alcohols, fatty acid methyl- and ethyl esters (FAMEs and FAEEs) and alkanes [9, 21, 34].
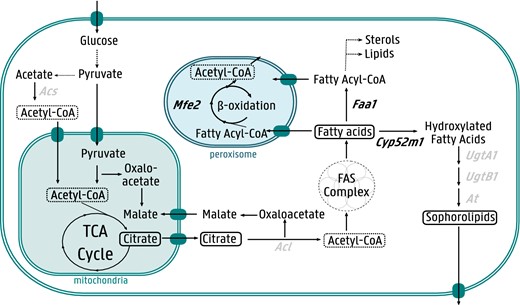
Overview of S. bombicola lipid metabolism and its link to sophorolipid biosynthesis. The enzymes targeted in this study are indicated in black bold. Mfe2 multifunction enzyme type 2, Faa1 fatty acyl-CoA synthetase, Acl ATPase citrate lyase, Cyp52m1 cytochrome P450 monooxygenase, UgtA1 UDP-glucosyltransferase, UgtB1 UDP-glucosyltransferase, At acetyltransferase, Acs acetyl-CoA synthase. Dashed arrows indicate more than one enzymatic reaction
Oleaginous microorganisms have a natural potential to accumulate high amounts of lipids ranging from 20 to 80% of the dry cell weight [19], mostly in a form of lipid droplets. However, because of their toxicity, free fatty acids constitute only a minor fraction of lipid droplets [5]. The first yeast that secretes significant amounts of free fatty acids (220 µM/L) was described by Scharnewski et al. [23] who engineered a S. cerevisiae strain deficient in Faa1 and Faa4. As already mentioned, fatty acids are degraded in the peroxisomes; hence, one straightforward and logical strategy to increase fatty acid production is to eliminate their catabolic pathways, including β-oxidation. In S. cerevisiae, deletion of Pox1 (acyl-CoA dehydrogenase responsible for the first reaction in the β-oxidation cycle) had a minimal effect on total fatty acid concentration [21]. However, the combination of β-oxidation inactivation (ΔPox1) with preventing cytosolic fatty acid activation by CoA (ΔFaa1, ΔFaa4) increased the total fatty acid production by 31% (106 mg/L) relative to the strain with only ΔFaa1 and ΔFaa4 deletions [17]. Inspired by these findings, Ledesma-Amaro et al. 2016, combined the natural lipogenic ability of an oleaginous organism with the secretion of free fatty acids. The obtained Y. lipolytica Δfaa1Δmfe2 strain secreted 1.1 g/L of free fatty acids and further strain engineering and process optimization with solvent co-extraction led to the production of 10.4 g/L of extracellular free fatty acids [16].
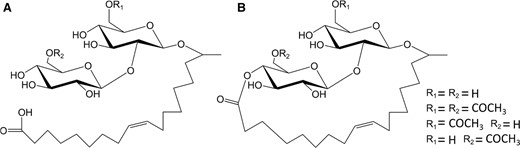
Structure of typical sophorolipids produced by S. bombicola a acidic b lactonic
In this work we aim to exploit the robustness and lipidic potential of the yeast S. bombicola to convert it from a glycolipid production platform into a free fatty acid cell factory. To do so, we engineered fatty acid catabolic pathways including preventing fatty acid activation by CoA, disruption the β-oxidation and blocking glycolipid synthesis. Additionally, these engineering strategies were applied to enhance production of sophorolipids. The engineered S. bombicola strains provide a proof of concept that this non-conventional yeast can be used for de novo production of industrially relevant compounds other than glycolipids, including fatty acids, and that the genetic engineering of the lipid metabolism proved to be a valuable approach to increase sophorolipid titers.
Materials and methods
Strains and culture conditions
S. bombicola strains used in this study are listed in Table 1. Uracil auxotrophic strain derived from S. bombicola wild‐type (ATCC22214) and S. bombicola Δcyp52m1 were used as the parental strains for genetic manipulations. All yeast strains were maintained on YPD (1% yeast extract, 2% peptone, 2% dextrose, and 2% agar) or 3C (10% glucose, 1% east extract 0.1% urea, 2% agar) and incubated at 30 °C. Antibiotic resistance of the yeast mutants was tested on YPD plates containing 600 µg/mL of nourseothricin or 500 µg/mL hygromycin B. Strains transformed with ura3 containing transformation cassette were selected on SD-ura plates (0.67% yeast nitrogen base without amino acids and uracil (DIFCO) and 2% glucose supplemented with complete supplement mixture CSM-ura (MP Biomedicals Europe)). For sophorolipid and fatty acid analysis during shake flask growth experiment, S. bombicola strains were cultivated on the Lang production medium (glucose: 132 g/L; yeast extract: 4 g/L; sodium citrate tribasic dihydrate: 5 g/L; NH4Cl: 1.5 g/L; KH2PO4: 1 g/L; K2HPO4: 0.16 g/L; MgSO4.7H2O: 0.7 g/L; NaCl: 0.5 g/L; CaCl2.2H2O: 0.27 g/L) described by [14]. Escherichia coli Top10 cells were used in all cloning experiments and were grown in Luria–Bertani (LB) medium (10 g/L tryptone, 5 g/L yeast extract, and 5 g/L sodium chloride) supplemented with 100 mg/L ampicillin. Liquid E. coli cultures were cultivated at 37 °C on a rotary shaker (200 rpm).
S. bombicola strains used in this research
Name . | Genotype . | References . |
---|---|---|
S. bombicola ATCC 22214 | Wild type | ATCC 22214 |
S. bombicola PT36 | Δura3 | [28] |
S. bombicola Δcyp52m1 | cyp52m1::hyg | [27] |
S. bombicola Δmfe2 | mfe2::ura3 | This study |
S. bombicola Δfaa1 | faa1::ura3 | This study |
S. bombicola Δcyp52m1Δfaa1 | cyp52m1::hyg, faa1::ura3 | This study |
S. bombicola Δcyp52m1Δmfe2 | cyp52m1::hyg, mfe2::ura3 | This study |
S. bombicola Δcyp52m1Δfaa1Δmfe2 | cyp52m1::hyg, faa1::ura3, mfe2::nat1 | This study |
Name . | Genotype . | References . |
---|---|---|
S. bombicola ATCC 22214 | Wild type | ATCC 22214 |
S. bombicola PT36 | Δura3 | [28] |
S. bombicola Δcyp52m1 | cyp52m1::hyg | [27] |
S. bombicola Δmfe2 | mfe2::ura3 | This study |
S. bombicola Δfaa1 | faa1::ura3 | This study |
S. bombicola Δcyp52m1Δfaa1 | cyp52m1::hyg, faa1::ura3 | This study |
S. bombicola Δcyp52m1Δmfe2 | cyp52m1::hyg, mfe2::ura3 | This study |
S. bombicola Δcyp52m1Δfaa1Δmfe2 | cyp52m1::hyg, faa1::ura3, mfe2::nat1 | This study |
S. bombicola strains used in this research
Name . | Genotype . | References . |
---|---|---|
S. bombicola ATCC 22214 | Wild type | ATCC 22214 |
S. bombicola PT36 | Δura3 | [28] |
S. bombicola Δcyp52m1 | cyp52m1::hyg | [27] |
S. bombicola Δmfe2 | mfe2::ura3 | This study |
S. bombicola Δfaa1 | faa1::ura3 | This study |
S. bombicola Δcyp52m1Δfaa1 | cyp52m1::hyg, faa1::ura3 | This study |
S. bombicola Δcyp52m1Δmfe2 | cyp52m1::hyg, mfe2::ura3 | This study |
S. bombicola Δcyp52m1Δfaa1Δmfe2 | cyp52m1::hyg, faa1::ura3, mfe2::nat1 | This study |
Name . | Genotype . | References . |
---|---|---|
S. bombicola ATCC 22214 | Wild type | ATCC 22214 |
S. bombicola PT36 | Δura3 | [28] |
S. bombicola Δcyp52m1 | cyp52m1::hyg | [27] |
S. bombicola Δmfe2 | mfe2::ura3 | This study |
S. bombicola Δfaa1 | faa1::ura3 | This study |
S. bombicola Δcyp52m1Δfaa1 | cyp52m1::hyg, faa1::ura3 | This study |
S. bombicola Δcyp52m1Δmfe2 | cyp52m1::hyg, mfe2::ura3 | This study |
S. bombicola Δcyp52m1Δfaa1Δmfe2 | cyp52m1::hyg, faa1::ura3, mfe2::nat1 | This study |
Shake flask scale fermentation
Strains grown on 3C plates were used to inoculate 5 mL Lang medium. After 48 h incubation on a rotary shaker (200 rpm) at 30 °C cells were transferred to fresh 50 mL Lang medium in 250 mL shake flasks. In experiments when sophorolipid production was enhanced by supplementing an external hydrophobic carbon source, 37.5 g/L of rapeseed oil (Coloruyt) was added to each culture after 48 h of incubation. The initial OD600 was adjusted to 0.5. All experiments were performed in triplicate and stopped after 8 days. Samples for fatty acid, sophorolipid, glucose, OD600 and pH analysis were collected every 48 h.
Cloning and strain engineering
S. bombicola fatty acyl-CoA synthase (SbFaa1, GenBank accession no. MK952999), multifunction enzyme type 2 (Mfe2, GenBank accession no. EU371724.1) single and double knockout strains were obtained by replacing the faa1or mfe2 coding sequences by the S. bombicola ura3 marker in the respective PT36 and Δcyp52m1 strains. S. bombicola Δcyp52m1Δfaa1Δmfe2 was created by replacing the mfe2 coding sequence by the nat1 selection marker cassette allowing selection based on nourseothricin resistance in strain already deficient in both Faa1 and Cyp52m1.
Plasmids harboring transformation cassettes were created by Circular Polymerase Extension Cloning (CPEC) assembly of PCR-amplified 1000 bp up- and downstream regions of gene of interest, selection marker and pJET (Thermo Fisher) vector backbone. S. bombicola was transformed with the linear cassettes (4 and 4.7 kb for the auxotrophic and dominant marker, respectively) by electroporation as described by [22]. Correct integration of the cassette was confirmed by colony PCR. All primers used in this study are listed in Table S1 in the Supplementary Material.
Sophorolipid and extracellular fatty acid analysis
Sophorolipids and fatty acids were extracted by adding 200 µl of ethyl acetate to 200 µl of culture broth and shaking for 5 min followed by 5 min centrifugation at 13,300 rpm. Final sophorolipid extraction (after 8 days of incubation) was performed by adding equal volume of ethyl acetate to the culture broth (50 mL), samples were shaken for 5 min and left for about 30 min to sediment. The top solvent layer was carefully collected and stored at − 20 °C or directly analyzed by UPLC (Waters Acquity H-Class UPLC) system coupled to an evaporative light scattering detector (ELSD; Waters Acquity). A Kinetex® 2.6 µm Polar C18 column (Phenomenex) was used for product separation. A combination of two eluents 0.5% acetic acid in miliQ and 100% acetonitrile was used at a flow rate of 0.6 mL/min. The initial 5% concentration of the acetic acid solution increased linearly until 95% during 6.8 min and then linearly decreased back to 5% during 1.8 min. Next, 5% acetonitrile was maintained until the run was completed (10 min/sample). For the ELSD detection, the nebulizer was cooled at 12 °C and the drift tube was kept at a temperature of 50 °C, the gain was set to 200. Prior to analysis, samples were filtered on 0.2 µm pore-size membranes. For sophorolipid quantification, a calibration curve based on dilution series of purified wild type sophorolipid mix was prepared as described in [20]. To quantify free fatty acids serial dilutions of oleic acid, palmitic acid and stearic acid (all from Sigma-Aldrich) were used as external standards.
Intracellular fatty acid analysis by GC-FID
Free fatty acids were analyzed in S. bombicola grown in Lang medium. After 72 h the cells were pelleted by centrifugation and washed twice with miliQ water. Lipids were extracted from freeze-dried yeast samples (250 mg) and methylated by a base-catalyzed step followed by an acid-catalyzed step and analyzed by Gas Chromatography coupled to Flame Ionization Detection (GC-FID) as described in [6].
Glucose quantification
Glucose was quantified by HPLC (Shimadzu) coupled to ELSD using Rezex ROA organic acid H+ column (50 × 7.8 mm, Phenomenex). Elution was performed with 0.08% acetic acid aqueous solution at 45 °C and a flow rate of 0.1 mL/min for 15 min. The drift tube of the ELSD was kept at a temperature of 55 °C and the gain was set to 5. Quantification was based on dilution series of external standards.
Fluorescence microscopy
Fluorescence microscopic images were acquired via confocal laser scanning microscopy (Nikon A1R, Nikon Instruments Inc., Paris, France), using a 60 × oil DIC N2 filled objective. Yeast was cultivated in 5 mL Lang medium and lipids were stained with BodiPy 493/503 dye (2.5 mg/mL in ethanol) during 10 min incubation at RT. Fluorescence emission was detected through a 525/50 nm band pass filter. The pinhole was set to 1 Airy unit and acquisition settings (laser power, gain, offset and scan speed) were kept constant throughout the experiment. Image processing was performed with Nikon Galvano scanner with a DU4 detector and later in ImageJ2 (Fiji).
Statistical analysis
All experiments were performed in triplicate from the respective independent starter culture. The results are expressed as mean values ± standard deviation (SD). The significance of differences among groups was analyzed using one-way analysis of variance (ANOVA) with Tukey’s multiple comparisons test with GraphPad Prism version 7.00 for Windows (GraphPad Software Inc.). A P value of less than 0.05 was considered statistically significant (P < 0.05).
Results and discussion
Free fatty acid synthesis by engineered S. bombicola strains
Deletion of Faa1 promotes free fatty acid secretion in S. bombicola
As explained in the introduction section, S. bombicola exhibits true potential for fatty acid synthesis judged by its ability to de novo synthesize high amounts of glycolipids, which rely on fatty acid intermediates. However, in normal physiological conditions, free fatty acids will not accumulate intracellularly to high concentrations due to their toxicity to microbial cells. This toxic effect can be circumvented by rerouting the free fatty acids to other pathways where they are either degraded (β-oxidation) or incorporated in more complex lipids like triglycerides or sphingolipids, which are subsequently stored in special organelles. Nevertheless, only the fatty acids activated with CoA can be used in afore mentioned processes. The tight regulation and the balance between free fatty acids and fatty acyl-CoA in the cytosol is controlled by Faa1. The S. bombicola fatty acyl-CoA synthetase candidate was selected based on a homology search against known Faa proteins. In contrast to baker’s yeast, in S. bombicola only one potential fatty acyl-CoA synthetase was identified (here referred to as SbFaa1). SbFaa1 shows the highest amino acid identity to Faa1 orthologues of both Y. lipolytica and S. cerevisiae: 46 and 45%, respectively.
In S. bombicola, the main distribution route for excess free fatty acids is sophorolipid biosynthesis (see Fig. 1) and one would assume that blocking this route would result in free fatty acid accumulation. However, S. bombicola Δcyp52m1, a strain unable to produce these glycolipids due to the knockout of the cytochrome P450 monooxygenase Cyp52m1 responsible for catalyzing the first step in sophorolipid biosynthesis, does not synthesize neither glycolipids nor excess fatty acids (even in stationary phase when all promoting factors like high C/N ratio are present). The unaffected growth and viability of the Δcyp52m1 mutant also suggest no toxic intracellular accumulation of free fatty acids, but rather supports the hypothesis that the extra fatty acids are redirected to other biochemical processes or degraded in the peroxisomes by means of β-oxidation [27]. To eliminate the possibility that potentially accumulated free fatty acids are degraded, we engineered a strain that in addition to Δcyp52m1 also has an inactive β-oxidation (Δmfe2). These and other mutated strains were cultivated on Lang medium that is developed to maximize sophorolipid biosynthesis. Moreover, due to its high carbon/nitrogen ratio (C/N = 30) it is also very suitable for fatty acid production. Nevertheless, the Δcyp52m1Δmfe2 strain did not secrete free fatty acids. The glucose consumption rates of all engineered strains can be consulted in Table S2 in the Supplementary Material. Consequently, to test if the activation of free fatty acids by Faa1 is a decisive element in unlocking the potential of this non-conventional yeast as a free fatty acid producer, we performed a deletion of faa1 in S. bombicola Δcyp52m1.
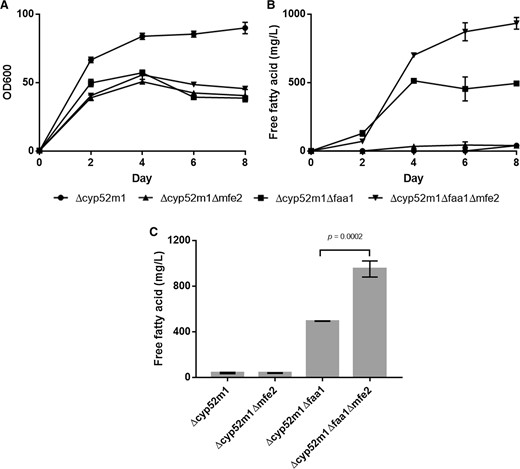
Shake flask cultivation of different S. bombicola Δcyp52m1 strains. a Growth, b Time course of the production of extracellular free fatty acids in Lang medium, c Total extracellular free fatty acid concentration after 8 days of cultivation. Results presented are mean values ± SD, n = 3. P < 0.05
The total intracellular fatty acid concentration in early stationary phase (day 3) in the Δcyp52m1Δfaa1 mutant and wild type was similar: 94.41 mg/gCDW and 99.50 mg/gCDW, respectively. However, their composition varied significantly (Table 2). The nearly double-fold higher concentration of C16:0 and C18:0 found in S. bombicola Δcyp52m1Δfaa1 could suggest the preferential activity of Faa1 synthetase towards saturated fatty acids. Unnecessary high fatty acid supply in the non-sophorolipid producing strain (Δcyp52m1) was also reflected in lower total intracellular fatty acid concentration. Interestingly, impaired β-oxidation did not result in extra accumulation of fatty acids in S. bombicola Δcyp52m1Δmfe2 but nearly 30% and 40% less fatty acids were detected in comparison to the single Δcyp52m1 knockout and wild type, respectively.
GC-FID analysis of total intracellular fatty acid composition of different S. bombicola strains at early stationary phase
mg/100 g . | C10:0 . | C12:0 . | C14:0 . | C15:0 . | C16:0 . | C17:0 . | C18:0 . | C20:0 . | C22:0 . | C24:0 . | C14:1 . | C16:1 . | C17:1 . | tC18:1 . | c9C18:1 . | c11C18:1 . | C20:1 . | C18:2n-6 . | C20:3n-6 . | C18:3n-3 . | C20:4n-3 . | n-3 PUFA . | Sum . |
---|---|---|---|---|---|---|---|---|---|---|---|---|---|---|---|---|---|---|---|---|---|---|---|
Δcyp52m1Δfaa1 | 1.2 | 4.2 | 52.9 | 0.8 | 1301.9 | 2.8 | 1141.5 | 13.5 | 1.1 | 0.8 | 1.4 | 783.7 | 1.3 | 12.3 | 6100.1 | 7.1 | 3.1 | 4.4 | 2.3 | 3.5 | 1.1 | 4.7 | 9441.1 |
Wild type | 0.5 | 1.3 | 17.3 | 4.2 | 692.3 | 14.6 | 643.5 | 6.3 | 0.0 | 0.0 | 3.1 | 744.5 | 57.8 | 13.4 | 7692.1 | 21.0 | 0.0 | 21.0 | 1.2 | 15.4 | 1.0 | 16.4 | 9950.2 |
Δcyp52m1Δmfe2 | 0.9 | 4.3 | 22.0 | 16.2 | 610.3 | 9.9 | 170.9 | 0.9 | 3.6 | 2.3 | 1.5 | 1066.3 | 47.6 | 45.4 | 4057.5 | 58.2 | 0.9 | 0.4 | 3.9 | 29.8 | 2.1 | 32.0 | 6155.0 |
Δcyp52m1 | 0.4 | 0.7 | 15.2 | 5.2 | 679.4 | 19.5 | 548.1 | 5.3 | 1.3 | 0.0 | 0.0 | 655.7 | 60.3 | 9.0 | 6436.8 | 23.8 | 0.0 | 0.0 | 2.6 | 8.4 | 0.0 | 8.4 | 8471.9 |
mg/100 g . | C10:0 . | C12:0 . | C14:0 . | C15:0 . | C16:0 . | C17:0 . | C18:0 . | C20:0 . | C22:0 . | C24:0 . | C14:1 . | C16:1 . | C17:1 . | tC18:1 . | c9C18:1 . | c11C18:1 . | C20:1 . | C18:2n-6 . | C20:3n-6 . | C18:3n-3 . | C20:4n-3 . | n-3 PUFA . | Sum . |
---|---|---|---|---|---|---|---|---|---|---|---|---|---|---|---|---|---|---|---|---|---|---|---|
Δcyp52m1Δfaa1 | 1.2 | 4.2 | 52.9 | 0.8 | 1301.9 | 2.8 | 1141.5 | 13.5 | 1.1 | 0.8 | 1.4 | 783.7 | 1.3 | 12.3 | 6100.1 | 7.1 | 3.1 | 4.4 | 2.3 | 3.5 | 1.1 | 4.7 | 9441.1 |
Wild type | 0.5 | 1.3 | 17.3 | 4.2 | 692.3 | 14.6 | 643.5 | 6.3 | 0.0 | 0.0 | 3.1 | 744.5 | 57.8 | 13.4 | 7692.1 | 21.0 | 0.0 | 21.0 | 1.2 | 15.4 | 1.0 | 16.4 | 9950.2 |
Δcyp52m1Δmfe2 | 0.9 | 4.3 | 22.0 | 16.2 | 610.3 | 9.9 | 170.9 | 0.9 | 3.6 | 2.3 | 1.5 | 1066.3 | 47.6 | 45.4 | 4057.5 | 58.2 | 0.9 | 0.4 | 3.9 | 29.8 | 2.1 | 32.0 | 6155.0 |
Δcyp52m1 | 0.4 | 0.7 | 15.2 | 5.2 | 679.4 | 19.5 | 548.1 | 5.3 | 1.3 | 0.0 | 0.0 | 655.7 | 60.3 | 9.0 | 6436.8 | 23.8 | 0.0 | 0.0 | 2.6 | 8.4 | 0.0 | 8.4 | 8471.9 |
GC-FID analysis of total intracellular fatty acid composition of different S. bombicola strains at early stationary phase
mg/100 g . | C10:0 . | C12:0 . | C14:0 . | C15:0 . | C16:0 . | C17:0 . | C18:0 . | C20:0 . | C22:0 . | C24:0 . | C14:1 . | C16:1 . | C17:1 . | tC18:1 . | c9C18:1 . | c11C18:1 . | C20:1 . | C18:2n-6 . | C20:3n-6 . | C18:3n-3 . | C20:4n-3 . | n-3 PUFA . | Sum . |
---|---|---|---|---|---|---|---|---|---|---|---|---|---|---|---|---|---|---|---|---|---|---|---|
Δcyp52m1Δfaa1 | 1.2 | 4.2 | 52.9 | 0.8 | 1301.9 | 2.8 | 1141.5 | 13.5 | 1.1 | 0.8 | 1.4 | 783.7 | 1.3 | 12.3 | 6100.1 | 7.1 | 3.1 | 4.4 | 2.3 | 3.5 | 1.1 | 4.7 | 9441.1 |
Wild type | 0.5 | 1.3 | 17.3 | 4.2 | 692.3 | 14.6 | 643.5 | 6.3 | 0.0 | 0.0 | 3.1 | 744.5 | 57.8 | 13.4 | 7692.1 | 21.0 | 0.0 | 21.0 | 1.2 | 15.4 | 1.0 | 16.4 | 9950.2 |
Δcyp52m1Δmfe2 | 0.9 | 4.3 | 22.0 | 16.2 | 610.3 | 9.9 | 170.9 | 0.9 | 3.6 | 2.3 | 1.5 | 1066.3 | 47.6 | 45.4 | 4057.5 | 58.2 | 0.9 | 0.4 | 3.9 | 29.8 | 2.1 | 32.0 | 6155.0 |
Δcyp52m1 | 0.4 | 0.7 | 15.2 | 5.2 | 679.4 | 19.5 | 548.1 | 5.3 | 1.3 | 0.0 | 0.0 | 655.7 | 60.3 | 9.0 | 6436.8 | 23.8 | 0.0 | 0.0 | 2.6 | 8.4 | 0.0 | 8.4 | 8471.9 |
mg/100 g . | C10:0 . | C12:0 . | C14:0 . | C15:0 . | C16:0 . | C17:0 . | C18:0 . | C20:0 . | C22:0 . | C24:0 . | C14:1 . | C16:1 . | C17:1 . | tC18:1 . | c9C18:1 . | c11C18:1 . | C20:1 . | C18:2n-6 . | C20:3n-6 . | C18:3n-3 . | C20:4n-3 . | n-3 PUFA . | Sum . |
---|---|---|---|---|---|---|---|---|---|---|---|---|---|---|---|---|---|---|---|---|---|---|---|
Δcyp52m1Δfaa1 | 1.2 | 4.2 | 52.9 | 0.8 | 1301.9 | 2.8 | 1141.5 | 13.5 | 1.1 | 0.8 | 1.4 | 783.7 | 1.3 | 12.3 | 6100.1 | 7.1 | 3.1 | 4.4 | 2.3 | 3.5 | 1.1 | 4.7 | 9441.1 |
Wild type | 0.5 | 1.3 | 17.3 | 4.2 | 692.3 | 14.6 | 643.5 | 6.3 | 0.0 | 0.0 | 3.1 | 744.5 | 57.8 | 13.4 | 7692.1 | 21.0 | 0.0 | 21.0 | 1.2 | 15.4 | 1.0 | 16.4 | 9950.2 |
Δcyp52m1Δmfe2 | 0.9 | 4.3 | 22.0 | 16.2 | 610.3 | 9.9 | 170.9 | 0.9 | 3.6 | 2.3 | 1.5 | 1066.3 | 47.6 | 45.4 | 4057.5 | 58.2 | 0.9 | 0.4 | 3.9 | 29.8 | 2.1 | 32.0 | 6155.0 |
Δcyp52m1 | 0.4 | 0.7 | 15.2 | 5.2 | 679.4 | 19.5 | 548.1 | 5.3 | 1.3 | 0.0 | 0.0 | 655.7 | 60.3 | 9.0 | 6436.8 | 23.8 | 0.0 | 0.0 | 2.6 | 8.4 | 0.0 | 8.4 | 8471.9 |

BodiPy staining microscopy images of S. bombicola strains on day 2 (D2) and day 6 (D6)
Blocking β-oxidation in S. bombicola Δcyp52m1Δfaa1 further increases free fatty acid production
Several metabolic engineering strategies have been used to improve free fatty acid synthesis in both oleaginous and non-oleaginous yeast and fungi [1, 8]. However, while the deletion of Faa1 promotes fatty acid secretion regardless of the organism’s ability to accumulate lipids or not, other approaches to further increase fatty acid production appear to be species dependent. Elimination of competing pathways including β-oxidation is an example of such a diverse outcome. In a Y. lipolytica mutant secreting fatty acids, the additional deletion of Mfe2 resulted in an increased extracellular free fatty acid concentration [16]. However, deletion of Pex10, the major peroxisome assembly factor, resulted in reduced lipid production, and impaired growth of R. toruloides [33]. To study whether blocking β-oxidation in the S. bombicola Δcyp52m1Δfaa1 mutant can still increase free fatty acid titers achieved by S. bombicola despite the non-producing phenotype of the Δcyp52m1Δmfe2 strain, we engineered a triple-knockout strain Δcyp52m1Δfaa1Δmfe2. This additional mutation surprisingly resulted in 0.933 g/L (± 0.04) secreted free fatty acids (83% improvement with reference to the double knockout, Fig. 3) with a majority of C18:1 (43.8%) followed by C18:0 and C16:0 (40.0 and 13.2%, respectively). Recent findings described by Yang and colleagues [32] provide possible reasons underlying this seemingly contradicting behavior of the Mfe2 mutants. Metabolic profiling and flux distribution analysis of S. bombicola wild type and Δmfe2 strains revealed that the drastic decrease in sophorolipid synthesis by the latter one is directly linked to the limited availability of cytosolic acetyl-CoA. This shortage is caused by the accumulation of acyl-CoA upon blocking β-oxidation which in turn inhibits ATP: citrate lyase activity (Fig. 1). Hence, combination of Mfe2 knockout with Faa1 deactivation might prevent this negative feedback mechanism by resolving acyl-CoA pressure.
Till date, the highest reported production of extracellular free fatty acid is 10.4 g/L by a highly engineered Y. lipolytica strain [16]. However, such an improvement was only possible thanks to combined efforts of strain and fermentation process optimization. The obtained concentration of secreted free fatty acids by S. bombicola mutants is comparable to the ones achieved by model oleaginous yeast Y. lipolytica subjected to the same genetic manipulations [16] and proves the high potential of this non-conventional yeast to become a platform organism for various lipid metabolism-derived compounds.
Deletion of Faa1 in S. bombicola directs fatty acids of different origin to sophorolipid production
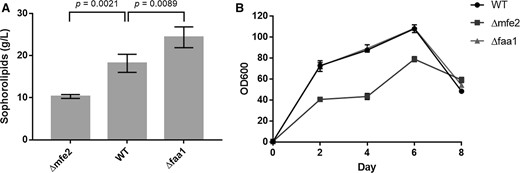
Sophorolipid production (a) and growth (b) of S. bombicola strains during shake flask growth experiment in Lang medium. Results presented are mean values ± SD, n = 3. P < 0.05
Nevertheless, the very high sophorolipid production titers that enabled commercialization of this non-conventional yeast and its sophorolipid production process are only achieved when a hydrophobic and a hydrophilic carbon source are provided in the production medium. To assess our strategy in an approach simulating commercial production conditions, we also evaluated sophorolipid synthesis by S. bombicola Δfaa1 on Lang medium to which rapeseed oil was added. After 8 days of cultivation in shake flasks both wild-type and mutant strains consumed all the provided carbon sources. While S. bombicola wild-type secreted 35.07 g/L (± 7.91), the Faa1 deletion strain secreted 52.69 g/L (± 5.12) sophorolipids, (P = 0.03, n = 3). Similar to de novo production, the composition of the formed glycolipids did not vary between the tested strains. Our results demonstrate that Faa1 in S. bombicola activates endogenous fatty acids as well as the ones provided in the culture media. Therefore, deletion of faa1 provided a bigger pool of free fatty acids that could be hydroxylated by Cytochrome P450 monooxygenase Cyp52m1 and used for sophorolipid synthesis.
Conclusion
In this research we used a metabolic engineering approach for extracellular fatty acid synthesis by S. bombicola, which included blocking the catabolic pathways such as glycolipid biosynthesis and β-oxidation and promoting free fatty acid secretion. The combination of these strategies (deletion of Faa1, Cyp52m1 and Mfe2) resulted in a S. bombicola strain secreting 0.933 g/L (± 0.04) free fatty acids. The produced fatty acids are predominantly oleic, stearic and palmitic acid. Interestingly, some of the modifications applied to create a free fatty acid-producing cell factory proved to be useful as well to increase sophorolipid synthesis. The overall concentration of de novo formed glycolipids by the Δfaa1 strain during the shake flask experiment with glucose as the sole carbon source was over 25% higher in comparison to the control strain (P = 0.0089). Moreover, the Faa1 deletion strain cultivated on medium containing both glucose and rapeseed oil, secreted about 50% more sophorolipids than the S. bombicola wild type (P = 0.03). However, the negative effect of the Mfe2 deletion on sophorolipid synthesis and yet beneficial effect on free fatty acid synthesis when combined with Δfaa1 demonstrates the complexity of the S. bombicola lipid metabolism and its interconnected pathways, inviting researchers for more in-depth investigation.
To conclude, we believe that our work is a promising start for the further exploration of S. bombicola as a platform for synthesis of oleochemicals. Moreover, the mutated strains that display increased sophorolipid production can be beneficial for further commercialization of S. bombicola in the biosurfactant market.
Acknowledgements
This work was funded by the Strategic Basic Research from the Research Foundation Flanders (FWO), (Sylwia Jezierska PhD Grant Number 151610). We would like to thank the Centre for Advanced Light Microscopy at Ghent University (Belgium) for the use of the fluorescence microscope during BodiPy staining experiments. We would also like to thank the Laboratory for Animal Nutrition and Animal Product Quality (Lanupro) at Ghent University for FID-MS analysis. The authors would like to acknowledge Dries Duchi for the excellent technical support during HPLC and UPLC analysis. The authors have no conflict of interest to declare.
References
Publisher's Note
Springer Nature remains neutral with regard to jurisdictional claims in published maps and institutional affiliations.