-
PDF
- Split View
-
Views
-
Cite
Cite
Linda P Guamán, Edmar R Oliveira-Filho, Carlos Barba-Ostria, José G C Gomez, Marilda K Taciro, Luiziana Ferreira da Silva, xylA and xylB overexpression as a successful strategy for improving xylose utilization and poly-3-hydroxybutyrate production in Burkholderia sacchari, Journal of Industrial Microbiology and Biotechnology, Volume 45, Issue 3, 1 March 2018, Pages 165–173, https://doi.org/10.1007/s10295-018-2007-7
- Share Icon Share
Abstract
Despite the versatility and many advantages of polyhydroxyalkanoates as petroleum-based plastic substitutes, their higher production cost compared to petroleum-based polymers has historically limited their large-scale production. One appealing approach to reducing production costs is to employ less expensive, renewable feedstocks. Xylose, for example is an abundant and inexpensive carbon source derived from hemicellulosic residues abundant in agro-industrial waste (sugarcane bagasse hemicellulosic hydrolysates). In this work, the production of poly-3-hydroxybutyrate P(3HB) from xylose was studied to develop technologies for conversion of agro-industrial waste into high-value chemicals and biopolymers. Specifically, this work elucidates the organization of the xylose assimilation operon of Burkholderia sacchari, a non-model bacterium with high capacity for P(3HB) accumulation. Overexpression of endogenous xylose isomerase and xylulokinase genes was successfully assessed, improving both specific growth rate and P(3HB) production. Compared to control strain (harboring pBBR1MCS-2), xylose utilization in the engineered strain was substantially improved with 25% increase in specific growth rate, 34% increase in P(3HB) production, and the highest P(3HB) yield from xylose reported to date for B. sacchari (Y P3HB/Xil = 0.35 g/g). This study highlights that xylA and xylB overexpression is an effective strategy to improve xylose utilization and P(3HB) production in B. sacchari.
Electronic supplementary material
The online version of this article (10.1007/s10295-018-2007-7) contains supplementary material, which is available to authorized users.
Introduction
Polyhydroxyalkanoates (PHAs) are polyesters accumulated in intracellular granules for carbon and energy storage [52] when bacteria are grown in medium with excess carbon source, but limited in at least one essential nutrient for growth. Their biodegradability, biocompatibility, and physical properties similar to synthetic polymers make PHAs an environmentally friendly alternative to petrochemical based plastics [24].
Since the first description of PHAs in 1926 by Lemoigne [30], several advances towards large-scale PHAs production have been achieved. However, high production costs make them more expensive than conventional plastics. One of the main factors contributing to high production costs is the high cost of carbon sources, which can account for up to 29% of the overall production cost, even when production is combined with onsite sugarcane milling [35]. Several efforts to lower poly-3-hydroxybutyrate P(3HB) production costs, including the use of inexpensive hemicellulosic biomass, have been conducted in recent years [40, 48]. Despite these efforts, production of biopolymers is still more expensive than petroleum-based polymers. In Brazil, sugarcane production has doubled over the past decade, corresponding to an increased bagasse availability of 208 million tons. [15, 54]. It is estimated that around 30% (dry weight basis) of the harvested sugarcane is hemicellulosic material [2] consisting of 70–80% xylose [38]. At least 50% of this xylose can be recovered after hydrolysis, resulting in an estimated 106 tons of xylose that could be available each harvest season in Brazil [48]. Instead, this biomass is currently either burned to generate energy in thermoelectrics or simply discarded [39].
Burkholderia sacchari LFM101 (LMG 19450T, CCT 6971T) [5, 42], hereafter LFM101, is able to utilize a variety of carbon sources including xylose and other hemicellulosic sugars from sugarcane bagasse [26, 28, 47]. LFM101 is able to accumulate up to 75% cell dry weight (CDW) as PHA [13] and produce high-value chemicals such as P(3HB), PHAs containing different HA-CoA monomers, xylitol, and xylonic acid [32–34, 41, 42]. Despite its capability to use xylose as a carbon source, the inherently slow growth rate of LFM101 and suboptimal yield using xylose must be significantly improved to use this bacterium as a chassis for bioproduction at industrial scale and elevate P(3HB) to an economically viable alternative to petroleum-based plastics.
In this work, the organization of the genes responsible for xylose assimilation in LFM101 is elucidated. In addition, the overexpression of the endogenous xylose isomerase and xylulokinase genes was demonstrated as an effective strategy to improve growth rate and P(3HB) production using xylose as a sole carbon source, reaching the highest yield reported to date corresponding to 95% of the maximum theoretical yield for B. sacchari.
Materials and methods
Strains and plasmids
Burkholderia sacchari LFM101 [5], recently reclassified as Paraburkholderia sacchari [10], was used in this study as a final host for plasmids. B. sacchari LFM1402 strain was constructed transforming pBBR1MCS-2-xylAB into LFM101. E. coli DH10B (F− endA1 deoR+ recA1 galE15 galK16 nupG rpsL Δ(lac)X74 φ80lacZΔM15 araD139 Δ(ara,leu)7697 mcrA Δ(mrr-hsdRMS-mcrBC) StrR λ−), was used for plasmid construction and propagation. The plasmid pBBR1MCS-2 [20] was used as a cloning and expressing vector (Fig. 1 Supplementary Material).
DNA manipulations
Plasmid DNA isolation, restriction enzyme digestion, agarose gel electrophoresis, and DNA ligation were performed by standard procedures [45]. The sequence of interest was amplified from the LFM101 genome using the primers xylA_F1 (ATAGCACCGCGGTTGCCGCCCTTCCCACCCCACG) and xylB_R1 (GTGACTCTCGAGCGCGCTACCAGGTAACGCGCC). Primers were designed to amplify the native promoter, RBS and the CDS of both xylA and xylB genes. Minimum Tm of 60 °C and 20 bp hybridization to target was used as a standard for primer design, and the desired restriction sites for cloning into pBBR1MCS-2 were added. The fragment was amplified using Phusion® High-Fidelity DNA Polymerase (Thermo Scientific, Waltham, Massachusetts, USA), in a Mastercycler nexus gradient thermal cycler (Eppendorf AG, Hamburg, Germany). Cycling conditions were as follows: 98 °C 1′, 30× (98 °C 30″, 63 °C 30″, 72 °C, 2′) 72 °C 5′).
The purified 2.94-kb PCR product was digested and ligated into SacII and XhoI sites of pBBR1MCS-2 to create pBBR1MCS-2-xylAB. Colonies were screened using blue/white selection and digestion with SacII and XhoI to confirm correct construction of pBBR1MCS-2-xylAB. Finally, plasmids from three colonies with expected sizes were isolated and sequenced using M13 primers (M13fwd, GTAAAACGACGGCCAGT, M13rev GAGCGGATAACAATTTCACACAGG). Electrocompetent cells of LFM101 were prepared according to previously established protocols [46]. Briefly, 1 mL of mid-exponential phase cell culture was inoculated into 50 mL of Luria–Bertani broth (LB) (250 mL flask) and incubated at 30 °C, 170 rpm for 6 h until cells reached OD600 = 0.6. The flask was incubated for 15 min on ice, cells were collected by centrifugation (15 min, 4500 rpm), washed twice with 50 mL of sterile nanopure water and once in sterile 10% glycerol. Finally, the cell pellet was resuspended and aliquoted (50 µL each) in sterile glycerol (20% final concentration). Plasmids were transformed by electroporation using the Gene Pulser Xcell electroporation system (Bio-Rad Laboratories, Inc. Hercules, California, USA) according to a previously described protocol [46]. 100 ng of DNA was added to 50 µL of electrocompetent cells and transferred to a 0.2-cm electroporation cuvette. Electroporation parameters were 25 µF, 200 Ohm, and 2500 V.
Culture media and growth conditions
LB (NaCl, 5 g/L; tryptone, 10 g/L; and yeast extract, 5 g/L) was used to construct and propagate plasmids in E. coli DH10B. When necessary, kanamycin (50 µg/mL), 5-bromo-4-chloro-3-indolyl-β-D- galactopyranoside (X-gal, 40 µg/mL), and Isopropyl β-D-1-thiogalactopyranoside (IPTG, 200 µM) were added to the medium. For growth curve and P(3HB) production assays, 10 mL of cells from an LB culture in mid-exponential phase was inoculated into 100 mL of mineral salts medium (MM) [44]. Xylose was autoclaved separately and aseptically added to the medium; the final concentration used is indicated in each case. For bioreactor assays, LFM1403 and LFM1402 cells were pre-incubated 24 h at 30 °C and agitation of 150 rpm in 1 L flasks with 200 mL MM [44] containing (NH4)2SO4 3 g/L and xylose 6 g/L. A set of fed-batch experiments was performed in two identical bioreactors (Applikon Biotechnology Inc. Delft, Netherlands) using a working volume of 2 L, at 30 °C for 60 h. The pH was set at 7.0 and controlled by automatic addition of NaOH (1 M) or H2SO4 (1 M). Dissolved oxygen (DO) was maintained above 40% of saturation by varying the agitation speed. Composition of MM to limit nitrogen availability (in g/L) was adapted as follows: KH2PO4 (0,39); (NH4)2SO4 (2,91); MgSO·7H2O (0.31); CaCl2·2H2O (0.010); (NH4)5Fe(C6H4O7)2 (0.06); NaCl (1); Xylose (40); trace elements solution (2 mL/L), which was prepared with H3BO3 (0.30 g/L); CoCl2·6H2O (0.20 g/L); ZnSO4·7H2O (0.10 g/L); MnCl2·4H2O (0.03 g/L); NaMoO4·2H2O (0.03 g/L); NiCl2·6H2O (0.02 g/L); CuSO4·5H2O (0.01 g/L). Cell dry weight (CDW), xylose and P(3HB) content were measured at the indicated times.
Gene annotation
LFM101 xylose isomerase (xylA) sequence previously reported by our group [27] was used to locate the xyl operon in the LFM101 genome. To annotate genes and to determine xylose operon structure, promoters, and terminators, a combination of Artemis Comparison Tool [7], Fgenes B and BLAST [3], was used. In addition, synteny analysis across Burkholderia species was performed using Absynte [9].
Xylose isomerase activity measurements
Xylose isomerase activity of B. sacchari extracts was measured in a buffer containing 50 mM Tris–HCl buffer (pH 7.5), 0.15 mM NADH, 1 mM TEA, 10 mM MgCl2, 10 U mL, and 50 mM of xylose following a described protocol [18]. Briefly, cells in early exponential growth phase grown in MMX medium (18 h, 30 °C, 150 rpm), were collected by centrifugation, washed twice with cold washing buffer (10% glycerol, 40 mM pH 8.0 Tris, 10 mM MgCl2, 5 mM NaCl and 10 mM β-mercaptoethanol), and resuspended in 1 mL of the same buffer. Cell pellets were disrupted by sonication and 35 µL of each cell extract was used for the xylose isomerase activity assay. Total cell protein concentration of extracts was determined by Bradford assay [4]. Absorbance at 340 nm for each strain was measured in biological triplicates in 96-well format, using Biotek Synergy H1 (BioTek, Winooski, Vermont, United States) at 25 °C and expressed as average ± SD.
Analytical methods
Biomass concentration
10 mL of culture was harvested by centrifugation at 10,600g and lyophilized in microtubes. Dry biomass was weighed using an analytical balance (Ohaus Adventurer, Parsippany, New Jersey, USA) and expressed as CDW (cell dry weight in grams per liter).
Xylose determination
Xylose concentration was determined by high-performance liquid chromatography (HPLC). 10 μL of each sample was injected into a Dionex HPLC (Ultimate 3000, Thermo Fisher Scientific Inc, Waltham, MA, USA) equipped with a sugar separation column (Aminex-HPX-87H). For detection, a differential refractometer (Shodex IR-101) was used. Separation occurred at 45 °C with H2SO4 solution (5 mM) at a flow rate of 0.6 mL/min. The standard curve was constructed using xylose solutions containing up to 3 g/L.
Poly-3-hydroxybutyrate content and composition measurements
12 mg of freeze-dried cells were subjected to propanolysis [43]. P(3HB) content was determined as described previously by Gomez, 1996 [13] with an Agilent 7890A GC System (Agilent Technologies, Santa Clara, California, USA) equipped with a HP-5 capillary column after sample split (1:25). Helium (0.8 mL/min) was used as carrier gas. Injector and FID temperature were 250 and 300 °C, respectively. The oven was programmed to maintain temperature at 100 °C for 1 min, then increase temperature at a rate of 8 °C/min up to 210 °C, which was maintained for 15 min. Benzoic acid was used as the internal standard (Sigma-Aldrich, Saint Louis, Missouri, USA).
Results and discussion
Xylose catabolism gene organization
Although the ability to use xylose and other pentoses as carbon sources has been described for different bacteria, including some E. coli and Burkholderia strains, the metabolic pathway responsible for xylose utilization in LFM101 has remained poorly understood. In E. coli, xylose isomerase pathway enzymes are encoded by two catabolic genes (xylA and xylB), two transporters (ABC-type transporter encoded by xylFGH and low affinity symporter xylE), and a transcriptional regulator encoded by xylR. The sequences and organization of xylose utilization loci present in some Burkholderia species are available [56], and a previous publication from our group has annotated the xylose isomerase (xylA) gene for LFM101 [27]. The only other study to suggest the organization of xyl genes in LFM101 inferred the organization using sequences of xyl loci present in other Burkholderia and Pseudomonas species. This previous study, suggested that in B. sacchari, xylB, encoding xylulokinase, is located distal to the xyl operon (> 500 000 bp) or may be encoded on a different chromosome. To further clarify the organization of the xyl operon in LFM101, and further understand some of the particular features of xylose catabolism in this bacterium, the sequences corresponding to xylB (GenBank submission ID 2074882), xylFGH, and xylR were identified in the B. sacchari genome draft published in 2015 by our group [1] using Artemis Comparison Tool [7], FgenesB [31] and BLAST [3]. As indicated in Table 1, LFM101 xylose catabolic and transporter proteins share high identity (55–67%) with E. coli xylose isomerase pathway proteins. Additional genomic scale searches failed to find other proteins involved in alternative xylose catabolic pathways (Weinberg and oxidoreductase) in LFM101. Taken together, these results suggest that in LFM101, the xylose isomerase pathway, is the main pathway responsible for xylose assimilation.
Features of B. sacchari xylose catabolic genes and protein identity to E. coli homologues
Gene . | Encoded protein . | Identitya (%) . | Protein length (aa) . |
---|---|---|---|
xylA | Xylose isomerase | 67 | 460 |
xylB | Xylulokinase | 60 | 497 |
xylF | Periplasmic binding subunit, xylose ABC transporter | 63 | 330 |
xylG | ATP-binding subunit, xylose ABC transporter | 55 | 518 |
xylH | Membrane binding subunit xylose ABC transporter | 55 | 399 |
xylR | Transcriptional dual regulator | 48 | 391 |
Gene . | Encoded protein . | Identitya (%) . | Protein length (aa) . |
---|---|---|---|
xylA | Xylose isomerase | 67 | 460 |
xylB | Xylulokinase | 60 | 497 |
xylF | Periplasmic binding subunit, xylose ABC transporter | 63 | 330 |
xylG | ATP-binding subunit, xylose ABC transporter | 55 | 518 |
xylH | Membrane binding subunit xylose ABC transporter | 55 | 399 |
xylR | Transcriptional dual regulator | 48 | 391 |
aIdentity with E. coli homologues
Features of B. sacchari xylose catabolic genes and protein identity to E. coli homologues
Gene . | Encoded protein . | Identitya (%) . | Protein length (aa) . |
---|---|---|---|
xylA | Xylose isomerase | 67 | 460 |
xylB | Xylulokinase | 60 | 497 |
xylF | Periplasmic binding subunit, xylose ABC transporter | 63 | 330 |
xylG | ATP-binding subunit, xylose ABC transporter | 55 | 518 |
xylH | Membrane binding subunit xylose ABC transporter | 55 | 399 |
xylR | Transcriptional dual regulator | 48 | 391 |
Gene . | Encoded protein . | Identitya (%) . | Protein length (aa) . |
---|---|---|---|
xylA | Xylose isomerase | 67 | 460 |
xylB | Xylulokinase | 60 | 497 |
xylF | Periplasmic binding subunit, xylose ABC transporter | 63 | 330 |
xylG | ATP-binding subunit, xylose ABC transporter | 55 | 518 |
xylH | Membrane binding subunit xylose ABC transporter | 55 | 399 |
xylR | Transcriptional dual regulator | 48 | 391 |
aIdentity with E. coli homologues

Organization of B. sacchari LFM101 xyl locus. xylAB (gray) encoding xylose isomerase and xylulokinase, respectively; xylF encodes a xylose-binding protein, xylG encodes an ATP-binding protein, and xylH encodes a membrane transporter ABC-type xylose transporter (white). xylR (black), encodes a transcriptional regulator of the the AraC family of regulators. Arrows represent the promoters identified using BPROM algorithm, a bidirectional region with two promoters in the intergenic region of xylR-A (solid arrows) and a promoter identified inside the coding sequence of xylB (dashed arrow)
Construction of a B. sacchari strain overexpressing XylAB proteins
The overexpression of genes involved in xylose catabolism, especially xylA, has been assessed in few microorganisms with variable success as a strategy to increase growth rate using xylose [16, 22, 25, 53]. Lopes and coworkers from our group reported that the overexpression of xylA did not improve either growth rate or biomass in B. sacchari [27]. In a separate study, overexpression of both xylA and xylB from Piromyces furiosus improved the production of ethanol in S. cerevisiae by eightfold [22]. Further, overexpression of E. coli xylA and xylB improved production of 1,3-propanediol by allowing simultaneous utilization of xylose and glycerol in Klebsiella pneumoniae [ 29 ].
Based on the xylose assimilation operon organization described in the present work, and as a step toward the development of B. sacchari strains capable of efficiently producing P(3HB) from renewable carbon sources, a strain carrying additional copies of B. sacchari x ylAB was constructed to test the effect of increased levels of xylA and xylB not only on growth rate (µ max/h), but also to potentially enhance production of P(3HB) using xylose. To do so, a 2.94-Kb fragment including the native promoter located 81 bp upstream of the xylA CDS, the native RBS, and the coding sequences of both xylA and xylB were amplified by PCR with the primers xylA_F1 and xylB_R1. The product was digested and ligated into the broad host range cloning vector pBBR1MCS-2 [20]. The confirmed pBBR1MCS-2-xylAB plasmid was transformed in B. sacchari LFM101 to construct B. sacchari LFM1402. Due to the lack of knowledge regarding molecular biology in B. sacchari LFM101, native promoter and RBS sequences were maintained to guarantee transcription and translation of xylAB.
xylAB overexpression leads to a substantial increase in xylose isomerase activity
Xylose isomerase is involved in the first step for assimilation of this pentose in bacteria. To evaluate the activity of this enzyme, LFM1402 and LFM101, transformed with pBBR1MCS-2 as a control (LFM 1403), were grown in MM with xylose as the sole carbon source. Total protein was extracted after 18 h of cultivation, and xylose isomerase activity was measured using a coupled enzymatic assay modified for 96-well plate format. As shown in Table 2, the strain LFM1402, transformed with pBBR1MCS-2-xylAB plasmid, showed a substantial increase (63%) in xylose isomerase-specific activity in comparison to both the wild-type LFM101 (0.0282 μmol/min mg/protein) and the strain LFM1403 harboring pBBR1MCS-2 (0.0304 μmol/min mg/protein). These data showed that, in B. sacchari, the multicopy effect results in overexpression of xylA in LFM1402 strain. Although the activity of xylB was not evaluated in the present study, considering: 1) the short distance of the intergenic region (78 bp), 2) the lack of a promoter region predicted by the bioinformatic analysis, and 3) experimental evidence showing that in other bacteria both genes are regulated as an operon [12, 51, 59], we assumed that expression of both proteins is increased in LFM1402 cells.
Xylose isomerase activities using a coupled enzymatic assay in B. sacchari
Strain . | Xylose isomerase specific activity (μmol/min mg/protein) . |
---|---|
B. sacchari LFM101 | 0.0282 ± 0.006 |
B. sacchari LFM1403 | 0.0304 ± 0.002 |
B. sacchari LFM1402 | 0.0845 ± 0.003 |
Strain . | Xylose isomerase specific activity (μmol/min mg/protein) . |
---|---|
B. sacchari LFM101 | 0.0282 ± 0.006 |
B. sacchari LFM1403 | 0.0304 ± 0.002 |
B. sacchari LFM1402 | 0.0845 ± 0.003 |
Xylose isomerase activities using a coupled enzymatic assay in B. sacchari
Strain . | Xylose isomerase specific activity (μmol/min mg/protein) . |
---|---|
B. sacchari LFM101 | 0.0282 ± 0.006 |
B. sacchari LFM1403 | 0.0304 ± 0.002 |
B. sacchari LFM1402 | 0.0845 ± 0.003 |
Strain . | Xylose isomerase specific activity (μmol/min mg/protein) . |
---|---|
B. sacchari LFM101 | 0.0282 ± 0.006 |
B. sacchari LFM1403 | 0.0304 ± 0.002 |
B. sacchari LFM1402 | 0.0845 ± 0.003 |
Overexpression of xylAB significantly improved specific growth rate and P(3HB) production using xylose
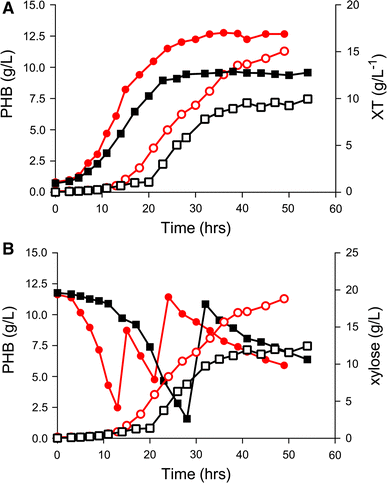
Fed-batch experiments in bioreactor in mineral medium, comparing B.sacchari LFM1403 (filled square and empty square) and recombinant LFM1402 harboring xylAB genes (filled circle and empty circle) using xylose as carbon source. a Comparison of total cell biomass (XT) in LFM1403 (filled square) and LFM1402 (filled circle) and P3HB (g/L) production by LFM1403 (empty square) and recombinant LFM1402 (empty circle); b comparison of P3HB (g/L) production profiles of LFM1403 (empty square) and recombinant LFM1402 (empty circle), plus xylose (g/L) concentration in bioreactor cultivation of control LFM 1403 (filled square) and recombinant LFM1402 (filled circle)
The accumulation phase initiated when cells stopped growing and started using the xylose to accumulate polymer within the cells (at a CDW of approximately 11 g/L for recombinant LFM1402). The maximum specific growth rate during the exponential growth phase was 0.20/h. After inoculation, xylose was manually fed to maintain sugar concentration around 15 g/L providing ideal conditions for P(3HB) accumulation. According to xylose consumption, manual feeding was needed after 30 h of growth in the case of LFM1403, and at 14 and 21 h for the recombinant strain (Fig. 2b). For both strains, gas chromatography analysis showed the presence of four-carbon monomers (Supplementary material—Fig. 2), characterizing the P(3HB) homopolymer (Fig. 2a, b), as previously described for B. sacchari when xylose was provided as carbon source [47]. The resulting parameters are summarized in Table 3.
Summary data from production of P(3HB) by control and recombinant B. sacchari in fed-batch bioreactor experiments in mineral medium with xylose as the sole carbon source
Strain . | CDW, g/L . | P(3HB), % . | P(3HB), g/L . | Time, h . | µmax/h . | Y P3HB/xyl, g/g . | P gP3HB/L h . |
---|---|---|---|---|---|---|---|
B. sacchari LFM1403 | 12.78 | 58 | 7.4 | 54 | 0.15 | 0.26 | 0.13 |
B. sacchari LFM1402 | 16.89 | 67 | 11.29 | 54 | 0.20 | 0.35 | 0.21 |
Strain . | CDW, g/L . | P(3HB), % . | P(3HB), g/L . | Time, h . | µmax/h . | Y P3HB/xyl, g/g . | P gP3HB/L h . |
---|---|---|---|---|---|---|---|
B. sacchari LFM1403 | 12.78 | 58 | 7.4 | 54 | 0.15 | 0.26 | 0.13 |
B. sacchari LFM1402 | 16.89 | 67 | 11.29 | 54 | 0.20 | 0.35 | 0.21 |
CDW Cell dry weight, %P3HB polymer content of CDW, µ max maximum specific growth rate, Y P3HB/xyl P3HB yield from xylose, P volumetric productivity
LFM1403—B. sacchari harboring pBBR1MCS-2
LFM1402—B. sacchari harboring pBBR1MCS-2-xylAB
Summary data from production of P(3HB) by control and recombinant B. sacchari in fed-batch bioreactor experiments in mineral medium with xylose as the sole carbon source
Strain . | CDW, g/L . | P(3HB), % . | P(3HB), g/L . | Time, h . | µmax/h . | Y P3HB/xyl, g/g . | P gP3HB/L h . |
---|---|---|---|---|---|---|---|
B. sacchari LFM1403 | 12.78 | 58 | 7.4 | 54 | 0.15 | 0.26 | 0.13 |
B. sacchari LFM1402 | 16.89 | 67 | 11.29 | 54 | 0.20 | 0.35 | 0.21 |
Strain . | CDW, g/L . | P(3HB), % . | P(3HB), g/L . | Time, h . | µmax/h . | Y P3HB/xyl, g/g . | P gP3HB/L h . |
---|---|---|---|---|---|---|---|
B. sacchari LFM1403 | 12.78 | 58 | 7.4 | 54 | 0.15 | 0.26 | 0.13 |
B. sacchari LFM1402 | 16.89 | 67 | 11.29 | 54 | 0.20 | 0.35 | 0.21 |
CDW Cell dry weight, %P3HB polymer content of CDW, µ max maximum specific growth rate, Y P3HB/xyl P3HB yield from xylose, P volumetric productivity
LFM1403—B. sacchari harboring pBBR1MCS-2
LFM1402—B. sacchari harboring pBBR1MCS-2-xylAB
The recombinant LFM1402 surpassed LFM1403 strain in all parameters. The yield (polymer produced from total xylose consumed (Y P3HB/xyl) was 0.35 g/g, which represents a 35% improvement compared to the control strain. Productivity (P) was 0.21 g/L.h, 61% higher than the control strain. Biomass increased 32% (from 12.78 to 16.89 g/L by the overexpression of xylAB). The enhanced xylose utilization strikingly improved the production of P(3HB) to 11.29 g/L, which represents a 52% improvement (7.4 g/L).
Several groups have worked over a decade to develop strains suitable for efficient pentose fermentation. Many of these groups are focused on using metabolic engineering for the development of novel, efficient pentose-fermenting strains of S. cerevisiae with variable levels of success [6, 14, 55]. One of the major drawbacks for the production of PHAs in S. cerevisiae is the low amount of P(3HB) accumulated per cell dry weight using glucose or xylose [21] The industrial workhorse, E. coli, has also been engineered to accumulate P(3HB) using xylose as the sole carbon source, although further work is still needed to increase P(3HB) productivity [57]. Even when using glucose as the carbon source coupled with genomic scale flux balance analysis and the introduction of a non-oxidative glycolysis pathway (NOG), E. coli was only able to generate 5.7 g/L P(3HB) and a mass yield of 0.31 g/g [58] In the case of E. coli, one of the major limitations for bioproduction using xylose is its slow growth with this pentose. This issue was recently addressed using a technique allowing simultaneous modulation of the expression of multiple genes in E. coli. CRISPR/Cas9-facilitated multiplex pathway optimization (CFPO), specifically, the replacement of the native regulatory sequences of xylAB, tktA (transketolase I), and talB (transaldolase B) enabled a growth rate of 0.25/h [61], the same value achieved in the present study.
Within the Burkholderia genus, B. cepacia has been reported to accumulate P(3HB-co-3HV) copolymers using xylose and levulinic acid as carbon source [17]. Using the same strain, Pan and coworkers achieved a production of 52% (CDW) of P(3HB); however, low productivity (0.09 g/L h) was reported due to the formation of inhibitory compounds after sugar maple hydrolysis to obtain xylose [36]. Recently, the model bacterium for PHAs production, Ralstonia eutropha was engineered to use xylose and accumulate P(3HB) through expression of xylAB genes from E. coli achieving 2.31 g/L of P(3HB) with a P(3HB) content of 30.95wt%) [19].
Recently, various approaches have been conducted in B. sacchari in order to improve both, the uptake of xylose and other hemicellulosic sugars and also the production of P(3HB) and other high-value metabolites [28]. In a different study using wheat straw hydrolysates rich in xylose and glucose, Cesario and coworkers studied growth and P(3HB) accumulation in B. sacchari, achieving 65% of CDW as P(3HB) with 0.22 g/g yield [8]. In a recent work of the same group, the maximum specific growth rate for B. sacchari growing in MM using xylose as carbon source was 0.18/h. However, it is important to mention that for reasons not described, this medium was supplemented with 1 g/L of yeast extract. Also, culture conditions in the bioreactor were optimized in the same research, and LFM101was able to produce P(3HB), xylitol, and xylonic acid [42] making B. sacchari even more interesting for industrial applications [32].
Conclusions
In the present work, the sequences corresponding to xylAB, xylFGH, and xylR were identified and the organization of the xylose utilization locus present in B. sacchari LFM101 is described. In addition, aiming to improve xylose utilization and potentially P(3HB) accumulation for large-scale production, a strain with multiple copies of xylAB was constructed (LFM1402). It is well documented in different microbes with xylose isomerase pathways that when using xylose as a carbon source, the flux distribution to the pentose pathway is substantially increased [23, 37, 51, 60]. Since NADPH is required for P(3HB) biosynthesis, higher specific growth rates might increase NADPH levels, also enhancing P(3HB) production. The P(3HB) improvement of the xylAB overexpressing strain without direct engineering of the P(3HB) production pathway indicates that the augmented xylose catabolism observed in the LFM1402 strain contributed to the improvement in P(3HB) yield. Our results demonstrate that xylAB overexpression is a successful strategy for improving xylose utilization as well as P(3HB) productivity, since LFM1402 xylose uptake is faster than in B. sacchari LFM1403.
Further studies should consider genetic modifications downstream of the xylose utilization pathway or combine this with other strategies to improve P(3HB) accumulation. For example, in addition to engineering the xylose utilization pathway, a transaldolase (talA) which catalyzes the conversion of sedo-7-heptulose (S7P) and glyceraldehyde-3-phosphate (G3P) to fructose-6-phosphate (F6P) and erythrose-4-phosphate (E4P) can be overexpressed to improve NADPH needed for P(3HB) biosynthesis as described by Song and coworkers [50]. Also, higher P(3HB) productivities may be obtained in higher density cultures as shown by Cesario using different fed batch strategies to scale-up P(3HB) production in B. sacchari [8]. Further engineering efforts in this bacterium would significantly benefit from having a platform allowing precise control of gene expression and application of synthetic biology and systems metabolic engineering approaches currently available when working with model microbes.
Results presented here are of great industrial interest since this work has improved the maximum specific growth rate in xylose by 31% as well as P(3HB) titer by overexpression of xylA and xylB in B. sacchari, achieving also the highest P(3HB) yield from xylose reported to date for B. sacchari (Y P3HB/Xil = 0.35 g/g).
The resulting strain is able to convert xylose to P(3HB) with 95% of the maximum theoretical yield representing an important advance towards lower cost, more economical production of P(3HB) from renewable sources [39].
Acknowledgements
This work was supported by the São Paulo Research Foundation (FAPESP 2010/51989-4 and 2016/00842-0, granted to L. F. S.), National Council for Scientific and Technological Development CNPq-Brazil (454371/2014-4, granted to L. F. S.) and Universidad Técnica de Ambato, Ecuador, through Department of Research and Development (DIDE) (Project 0932-CU-P-2016 and DIDE Internal Funding Project, granted to C. B. O.). Thanks to the National Council for Science Technology and Innovation of Ecuador (SENESCYT) for the Ph.D. fellowship granted to L. P. G.
Author contributions
LFS conceived and supervised the study; LPG, JGCG, MKT and LFS designed experiments; LPG, EROF and CBO performed experiments; LPG, EROF, CBO, JGCG, MKT and LFS analyzed the data; LPG and CBO wrote the manuscript; All authors made critical revisions of the manuscript and approved the final article.
References
LOPES MSG (2010) Produção de plásticos biodegradáveis utilizando hidrolisado hemicelulósico de bagaço de cana-de-açúcar
Raicher G (2011) Análise econômica da produção de polímeros biodegradáveis no contexto de uma biorefinaria a partir de cana-de-açucar. Doctoral dissertation
Sambrook J, Russell DW (2001) Molecular cloning: a laboratory manual. Cold Spring Harbor Laboratory Press, 2100p
Solovyev V, Salamov A (2011) Automatic annotation of microbial genomes and metagenomic sequences. In: Li RW (ed) Metagenomics and its applications in agriculture, biomedicine, and environmental studies. Nova Science Publishers, pp.61–78
UNICA (2016) Etimativa safra 2016/2017. In: Coletiva de imprensa ESTIMATIVA SAFRA 2016/2017. http://webcache.googleusercontent.com/search?q=cache:cHAHJ08nPjgJ:www.unica.com.br/download.php%3FidSecao%3D17%26id%3D10968146+&cd=1&hl=en&ct=clnk&gl=br. Accessed 15 Nov 2016