-
PDF
- Split View
-
Views
-
Cite
Cite
Vanessa O Leitão, Eliane F Noronha, Brenda R Camargo, Pedro R V Hamann, Andrei S Steindorff, Betania F Quirino, Marcelo Valle de Sousa, Cirano J Ulhoa, Carlos R Felix, Growth and expression of relevant metabolic genes of Clostridium thermocellum cultured on lignocellulosic residues, Journal of Industrial Microbiology and Biotechnology, Volume 44, Issue 6, 1 June 2017, Pages 825–834, https://doi.org/10.1007/s10295-017-1915-2
- Share Icon Share
Abstract
The plant cell wall is a source of fermentable sugars in second-generation bioethanol production. However, cellulosic biomass hydrolysis remains an obstacle to bioethanol production in an efficient and low-cost process. Clostridium thermocellum has been studied as a model organism able to produce enzymatic blends that efficiently degrade lignocellulosic biomass, and also as a fermentative microorganism in a consolidated process for the conversion of lignocellulose to bioethanol. In this study, a C. thermocellum strain (designated B8) isolated from goat rumen was characterized for its ability to grow on sugarcane straw and cotton waste, and to produce cellulosomes. We also evaluated C. thermocellum gene expression control in the presence of complex lignocellulosic biomasses. This isolate is capable of growing in the presence of microcrystalline cellulose, sugarcane straw and cotton waste as carbon sources, producing free enzymes and residual substrate-bound proteins (RSBP). The highest growth rate and cellulase/xylanase production were detected at pH 7.0 and 60 °C, after 48 h. Moreover, this strain showed different expression levels of transcripts encoding cellulosomal proteins and proteins with a role in fermentation and catabolic repression.
Introduction
The increase in demand for fossil fuels together with the environmental impacts caused by their combustion has led to increased interest in the development of sustainable and environmentally friendly industrial processes for fuel production. In this context, bioethanol production from lignocellulosic biomass (2nd generation bioethanol) is a technically viable alternative. Using this approach, agro-industrial residues such as sugarcane straw and bagasse, cotton waste, corn stover and rice straw, as well as low-cost feedstocks high sugar content can be deconstructed and fermented to bioethanol [6, 23].
However, in order to obtain fermentable sugars for second-generation bioethanol production, a series of steps is first required to deconstruct the raw material. These steps are aimed at particle size reduction, removal of lignin/hemicellulose, and hydrolysis (sacharification). The hydrolysis step to obtain fermentable sugars can be accomplished using either chemicals or biocatalysts, but the latter alternative stands out as it produces less pollution and is more sustainable [18].
Lignocellulosic residues as sugarcane straw or bagasse, cotton waste, like others, presented differences in their content of cellulose, lignin and hemicelluloses. In general, plant cell walls are constituted of about 40–50% of cellulose, 30–40% of hemicelluloses and 9–20% of lignin [9, 34]. Cellulose is constituted by d-glucose units joined by β(1,4)-glycosidic bonds forming long filaments, which in association formed the cellulose fibers [8]. Among hemicelluloses, xylan is the major component, and also the second most prevalent polymer in plant cell walls. Xylan backbone is formed by d-xylose units joined by β(1,4)-glycosyl bonds; in contrast to cellulose, xylan could present acetyl, arabinose and glucuronic acid in its side chain [5, 31]. Lignin covers the layers of cellulose and hemicelluloses, enhancing the plant cell wall resistance and recalcitrance. It is a complex macromolecule composed of phenylpropane units, mainly coumaryl alcohol, coniferyl alcohol, and sinapyl alcohol, nonlinearly and covalent randomly linked [14].
Given the complex composition of the plant cell wall, its complete deconstruction requires different enzymes acting in synergy. Cellulosic fibers are hydrolyzed through the action of cellulases, classified into three types according to their mode of action: endoglucanases (EC 3.2.1.4), cellobiohydrolases (EC 3.2.1.91) and β-glucosidases (EC 3.2.1.21). Xylan hydrolysis follows a similar degradation pattern, where the main backbone is cleaved by β-xylanase (EC 3.2.1.8) and β-xylosidase (EC 3.2.1.37) yielding d-xylose, while side chains are hydrolyzed by α-l-arabinofuranosidase (EC 3.1.1.73), α-d-glucoronidase (EC 3.2.1.139), and acetylxylanestarase (EC 3.1.1.72) [6, 22, 39]. The laccases (polyphenol oxidases EC 1.10.3.2) and lignin peroxidases (E.C.1.11.1.7) are responsible for the biodegradation of lignin [19].
Among the microorganisms capable of degrading lignocellulosic biomass, Clostridium thermocellum is quite remarkable as it was the first to be recognized as capable of assembling enzymes into a well-organized complex named the cellulosome, which presents a high level of cellulolytic activity [11, 12, 32]. This feature made C. thermocellum an important source of robust industrial enzymes. Although C. thermocellum’s cellulosomes are the most highly studied to date, there is still a lack of information regarding the molecular mechanisms involved in the control of expression of plant cell wall-degrading enzymes, and the effect of carbon sources on the cellulosome’s composition and assembly. Considering the potential of thermophilic anaerobic bacteria as a source of enzymes for industry, we have been working on their isolation and characterization to obtain enzymes suitable for biotechnological processes. We isolated, from goat rumen, a bacterium (isolate B8) that was able to grow on cellulose and produce cellulases and hemicellulases, which were then selected for further analysis. The present study aimed to characterize C. thermocellum isolate B8, and to analyze transcript levels of cellulosomal and fermentative pathway genes during growth in the presence of cellulose, sugarcane straw and cotton waste.
Materials and methods
Isolation and maintenance of C. thermocellum
Clostridium thermocellum B8 was isolated and identified as previously described [15]. Bacterial cells were preserved in a glycerol solution (50% w/v) at −80 °C and then regrown for 2 days on liquid media to serve as pre-inoculum for liquid cultures, which were kept at 4 °C until used for seeding.
Effect of time, temperature and pH on C. thermocellum B8 growth
Bacterial growth was carried out as previously described [2], using commercial cellulose as a carbon source (microcrystalline cellulose, Sigma-Aldrich®). Briefly, C. thermocellum B8 was cultivated under anaerobic conditions in reducing liquid medium [27] containing 2% (w/v) commercial cellulose. Culture flasks containing 100 mL of media inoculated with a fresh C. thermocellum culture were incubated at 60 °C and cell growth was monitored for 60 h. To evaluate the effect of temperature on bacterial growth, cultures flasks were incubated for 48 h at 35, 45, 60, and 75 °C. The effect of pH on bacterial growth was evaluated at 60 °C for 48 h in culture media at pH 4, 5, 6, 7, and 8. Cell growth was quantified by measuring the culture optical density at 650 nm. All bacterial growth experiments were performed in triplicate.
Growth on lignocellulosic residues and microcrystalline cellulose
Before their use as carbon source, the residues (cotton waste and sugarcane straw) were first autoclaved at 120 °C for 40 min, washed with tap water to remove soluble sugars and impurities, dried at 75 °C and finally milled. The resulting powder was used as a carbon source to supplement the growth media. Cultivation on agricultural residues and commercial cellulose (microcrystalline cellulose, Sigma-Aldrich®) was carried out as previously described [2] using 2% (w/v) of microcrystalline cellulose, sugarcane straw or cotton waste, as carbon source. Growth was carried at 60 °C for 48 h to obtain enzymatic samples (supernatant and residual substrate-bound proteins-RSBP) and for 24, 34 or 48 h to obtain bacterial cells further used for RNA extraction.
Protein preparation
Supernatant and residual substrate-bound proteins (RSBP) were obtained and prepared as described in Blume et al. [2]. Briefly, bacterial cultures obtained as described above were filtered through Whatman filter paper #5 under vacuum, to retain the residual insoluble substrate (cellulose, sugarcane straw and cotton waste). The flow throw, named culture filtrate, was centrifuged at 4000×g for 10 min; the supernatants were collected, dialyzed, concentrated by lyophilization and then used in enzymatic assays and protein quantification.
Residual insoluble substrates, obtained as above described, were further washed with 200 mL of 0.5 M Tris–HCl buffer (pH 8.0). Next, to obtain residual substrate-bound proteins (RSBP), the cleaned residual substrates were washed twice with 50 mL of 0.5 M Tris–HCl buffer (pH 8.0), and then three times with 30 mL of distilled water. The resulting washed water was collected as source of the residual substrate-bound proteins (RSBP), which is a cellulosome-enriched sample. Before their use in enzymatic assays and protein quantification, RSBP samples were concentrated by lyophilization.
Enzymatic assays and protein quantification
CMCase (endoglucanase), xylanase and avicelase (cellobiohydrolase) activities were evaluated by mixing 50 µL of enzyme samples (supernatant and/or RSBP) with 50 µL of 50 mM sodium acetate bufffer (pH 5) and 50 µL of substrate (3% w/v) (carboxymethyl cellulose, xylan from oat spelt, Avicel). Reactions were carried out at 60 °C for 30 min (or 1 h for avicelase), and stopped by adding 300 µL of 3,5-dinitrosalicylic acid (DNS) [24], followed by boiling for 10 min. Absorbance was read at 540 nm. Enzymatic activities were expressed as micromoles of reducing sugar formed per minute per milliliter of enzyme solution (UI mL−1). d-Glucose and d-xylose were used as standards. Protein concentration was determined using the Bradford method [4], using bovine serum albumin as a standard. Statistical analysis was performed using the R Software version 3.0.2. for ANOVA and Tukey’s tests [17].
RT-qPCR analysis
Clostridium thermocellum cells were harvested from cultures containing microcrystalline cellulose, sugarcane straw and cotton waste as carbon source, grown for 24, 34 and 48 h at 60 °C. Briefly, each bacterial culture was filtered through Whatman filter paper #5 under vacuum, to retain the residual insoluble substrate (cellulose, sugarcane straw and cotton waste). The flow throw containing bacterial cells was centrifuged at 4000×g for 10 min, the supernatants were discarded, and cells collected to be used in the first step of RNA extraction.
Bacterial cells were then disrupted and RNA was extracted using TRI-Reagent (Sigma-Aldrich), according to the manufacturer’s instructions. Total RNA (5 µg) from each pooled sample was reverse transcribed into cDNA in the presence of random hexamer primers using the Revertaid™ First Strand cDNA synthesis kit (Fermentas). Quantitative real-time PCRs were performed in an iQ5 real-time PCR system (Bio-Rad) to evaluate transcript levels for genes encoding cellulosomal proteins: cipA (scaffoldin component of the cellulosome), celS (exoglucanase component of the cellulosome), manA (cellulosome-associated mannanase), olpA (cellulase-anchoring protein), celob (cellobiohydrolase) and sdbA (scaffoldin-dockerin binding protein); genes encoding fermentation enzymes: cbp (cellobiose phosphorylase), adhY (alcohol dehydrogenase), ldh (lactate dehydrogenase), ack (acetate kinase) and hydA (hydrogenase); and genes encoding catabolite repression proteins: crpZ and crp (cyclic AMP (cAMP) -binding proteins), and hprK and hprX (Hpr-related proteins involved in the phosphotransferase system regulating carbohydrate metabolism). Each reaction (20 μl) contained 10 μl of MAXIMA® SYBR-green PCR Master Mix (Fermentas), forward and reverse primers (500 nM each), cDNA template, and nuclease-free water. PCR cycling conditions were: 10 min at 95 °C (1 cycle), 15 s at 95 °C followed by 1 min at 60 °C (40 cycles), and a melting curve of 1 min at 95 °C followed by 30 s at 55 °C and a final ramp to 95 °C with continuous data collection (1 cycle) to test for primer dimers and nonspecific amplification. Primer pairs were designed using the Applied Biosystems Prime Express Software. The recA gene was used as calibrator. Transcripts levels were calculated from the threshold cycle according to the 2(−ΔΔCT) method [20].
Results and discussion
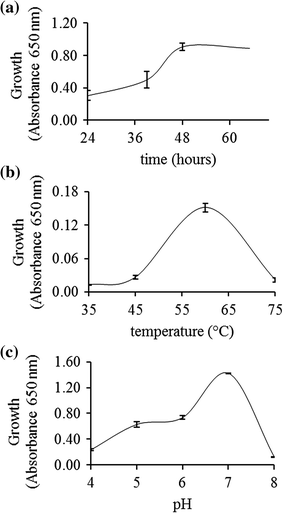
Effect of time (a), temperature (b) and pH (c) on C. thermocellum B8 growth in reducing liquid media containing microcrystalline cellulose (2%) as unique carbon source. Assays were performed in triplicate, and vertical bars represent standard deviation
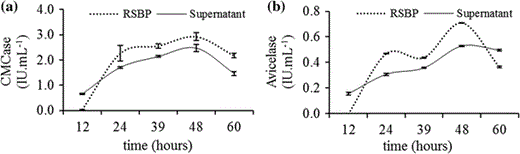
Time course production of CMCase (a) and Avicelase (b) by C. thermocellum B8 during growth in reducing liquid media containing microcrystalline cellulose (2%) as unique carbon source. Dotted lines represent activity from residual substrate-bound proteins (RSBP) and solid lines represent activity from supernatant samples. Vertical bars represent standard deviation from technical replicates (n = 3)

Effect of pH (a,b) and temperature (c,d) on CMCases (a,c) and avicelases production by Clostridium thermocellum B8 during growth in reducing liquid media containing cellulose (2%) as unique carbon source for 48 h. Dotted lines represent activities from residual substrate-bound proteins (RSBP) and solid lines are activities from supernatant samples. Vertical bars represent standard deviation from technical replicates (n = 3)
The influence of pH on enzyme production has been previously reported for T. reesei during cellulose degradation [1]; however, this has not been shown for C. thermocellum. Temperature control of gene expression is common, being a factor in organisms ranging from prokaryotes to eukaryotes. Bacillus licheniformes and S. cerevisiae have several genes differentially expressed in response to temperature during iron limitation and xylose fermentation, respectively [16, 28]. However, as with pH, there is also a lack of information about temperature-responsive genes in C. thermocellum.
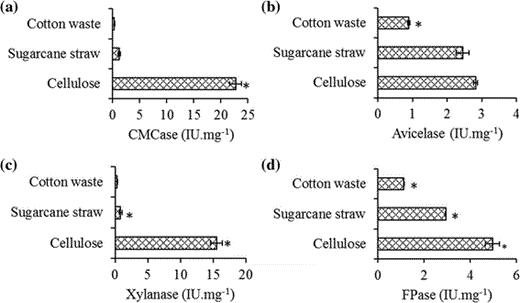
CMCase (a), avicelase (b), FPAse (c), and xylanase (d) activities of RSBP. C. thermocellum was grown for 48 h at 60 °C in reducing liquid media containing, cotton waste sugarcane straw and crystalline cellulose as carbon source. RSBP samples were obtained from the residual substrates. Narrow horizontal bars represent standard deviation from technical replicates (n = 3) *p < 0.5
Increased levels of avicelase and total cellulase activity (FPase) were detected for RSBP eluted from sugarcane straw and cotton waste (Fig. 4), showing that the carbon source can induce different patterns of cellulase and hemicellulase production. This is in agreement with previous papers that demonstrated different patterns of cellulases and xylanases produced by C. thermocellum in the presence of cellobiose, cellulose, glucose and fructose as carbon sources [7]. These different RSBP activities associated with the substrate used as carbon source highlight the need for proteomic analysis of RSBP samples; this information could be valuable in helping to understand plant cell wall degradation by cellulosomes.
In addition to its potential as a source of plant cell wall hydrolases organized in cellulossomes, C. thermocellum is also a promising candidate for consolidated processes, in which lignocellulosic biomasses are hydrolyzed and the sugars liberated fermented in a unique step to obtain bio products, like as bioethanol or H2. Therefore, our research group focused on the understanding of the overall process of lignocellulosic biomasses metabolizing by C. thermocellum B8, which includes at least three different levels: biomass hydrolysis, fermentation and metabolic flux control. Thus, in the present study we evaluated also the effect of different carbon sources in the expression of genes encoding enzymes related to plant cell wall deconstruction, fermentation, as well as catabolic control.
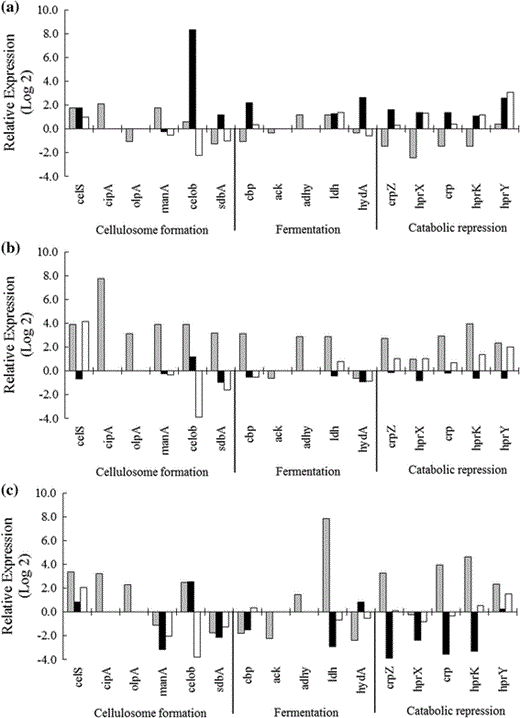
Analysis and quantification of transcript levels of cellulosomal proteins encoding genes, celS (exoglucanase), cipA (scaffoldin protein), olpA (cellulose-anchoring protein), manA (mannanase), celob (cellobiohydrolase), sdbA (scaffoldin-dockerin binding protein); fermentation genes, cbp (cellobiose phosphorylase), ack (acetate kinase), adhy (alcohol dehydrogenase), ldh (lactate dehydrogenase) and hydA (hydrogenase); catabolic repression genes, crpZ and crp (cyclic AMP (cAMP)-binding proteins), hprK, hprX and hprY (Hpr- system regulator of carbohydrate metabolism). C. thermocellum was grown for 24 (gray column), 34 (black column) and 48 h (white column) at 60 °C, in reducing liquid media containing microcrystalline cellulose (a), cotton waste (b) and sugarcane straw (c) as unique carbon source. The data were presented as fold change using the 2−ΔΔCT method
In contrast, celob and sdbA reached their highest transcript levels after 34 h of growth in the presence of commercial cellulose as the carbon source. Different patterns of expression were detected in the presence of cotton waste and sugarcane straw. Regarding cotton waste as the carbon source, the highest transcript levels of celob and sdbA were obtained at 24 h, decreasing after this time and reaching maximal down-regulation at 48 h. In the presence of sugarcane straw as the carbon source, sbpA was down-regulated and celob presented a constant level of expression at 24 and 34 h, becoming down-regulated at 48 h (Fig. 5).
The transcript level of cipA was increased after 24 h of growth in all carbon sources; however, the highest value was detected for growth in the presence of cotton waste. Another gene related to cellulosome assembly, olpa, also presented altered transcriptional levels, presenting increased expression in the presence of cotton waste and sugarcane straw and down-regulation in the presence of microcrystalline cellulose (Fig. 5).
The cellobiose phosphorylase-encoding gene (cbp) was up-regulated, presenting highest transcript levels after 24, 34 and 48 h of growth in the presence of cotton waste, microcrystalline cellulose or sugarcane straw as carbon source, respectively. In addition, celS, sdbA, manA, olpA and cbp transcripts presented their highest levels for growth cultures containing cotton waste as the carbon source (Fig. 5).
Increases in transcript levels after 24 or 34 h of growth characterize the B8 log phase of growth, during growth on commercial cellulose (Fig. 5). Indeed, high gene expression during growth in the presence of cellulose and agricultural wastes as carbon source could be directly related to increased energy requirements in the initial growth stages. The expression pattern of celS observed in the present work is in agreement with data previously published, in which Wilson et al. [37] showed an increase in celS transcript levels after the first 12 h of C. thermocellum ATCC 27405 growth in the presence of pretreated Populus and switch grass.
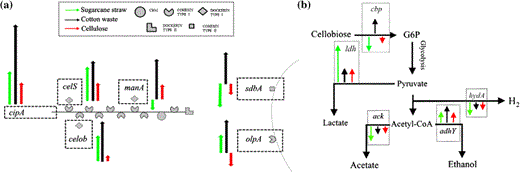
Diagram representing relative expression of cellulosomal proteins encoding genes, data presented in Fig. 5. a celS (exoglucanase), manA (mannanase), celob (cellobiohydrolase), cipA (scaffoldin), sdbA (scaffoldin-dockerin binding protein) and olpA (cellulase-anchoring protein). b fermentation genes, cbp (cellobiose phosphorylase), ldh (lactate dehydrogenase), hydA (hydrogenase), ack (acetate kinase) and adhY (alcohol dehydrogenase). Arrows size represents fold change. Sugarcane straw (green arrow), cotton waste (black arrow) and cellulose (red arrow)
Therefore, more recalcitrant substrates require increasing on the levels of cellulosome building blocks and then a larger number of the assembled complexes attached to bacterial cells to carry an efficient hydrolysis. Expression control of cellulosome-encoding genes by carbon sources has also been shown for C. thermocellum grown on cellulose, cellobiose and yellow poplar [30, 36, 38]. Bacterial cells grown on liquid media containing commercial cellulose as carbon source only showed the highest level of expression of the cellobiohydrolase encoding gene, celob, later than that detected for growth in the presence of cotton waste and sugar cane straw as carbon source (Figs. 5, 6a). These results suggest that celob expression in the presence of more recalcitrant cellulosic substrates is an earlier event and might have a central role on cotton waste and sugar cane straw deconstruction during the first 24 h.
Overall, time of growth and carbon sources modulated cellulossomal genes expression in C. thermocellum B8, which is in agreement with previous reports that showed the effect of cellulose and xylan onto cellulossomal genes expression control in C. thermocellum strains [25, 26]. Indeed, this sort of gene expression control is mediated by the interaction between sugars released during the substrate hydrolysis and sigma factors [25, 26]. Therefore, it seems that a different set of sugars are released during carbon sources hydrolysis by C. thermocellum B8 in a time-dependent manner, and these further modulate in different ways the molecular expression of cellulosomal genes.
The carbon source also seems to affect lactate, ethanol and acetate fermentation, and hydrogen production in C. thermocellum (Fig. 5, 6b). Transcript levels of ldh were highest for C. thermocellum B8 grown on sugarcane straw after 24 h of growth. In addition, ack was down-regulated regardless of all carbon sources, and adhY was up-regulated, presenting its highest levels for growth in the presence of sugarcane as the carbon source (Figs. 5, 6b). On the other hand, for hydA we detected up-regulation after 34 h when cellulose or sugar cane straw was used as the carbon source (Fig. 5).
The ability of C.thermocellum to deconstruct cellulosic material and metabolize monomeric sugars into chemicals of commercial interest such as lactate, acetate, ethanol and hydrogen is well known. Indeed, the balance between these fermentation processes and their products is directly correlated to the carbon source provided to C. thermocellum [13]. As previously described by Freier et al. [13], which showed that cellulose fermentation by C. thermocellum JW20 resulted in a higher concentration of hydrogen in comparison with ethanol and acetate production.
The result showing highest transcript levels for hydA during growth in crystalline cellulose indicates that the B8 isolate is also capable of producing hydrogen during fermentation, and this is in agreement with data previously reported [13]. Indeed, our data are consistent with a central role for hydA in hydrogen production by C. thermocellum, making this gene a potential target for the development of C. thermocellum strains that are more efficient in converting cellulose into ethanol or products other than hydrogen. However, the most remarkable result is the increased expression levels of genes related to ethanol and lactate production (adhY and ldh) in comparison to hydA and ack (Figs. 5, 6b), suggesting that during growth on sugarcane residue and cotton waste, C. thermocellum B8 preferentially produces ethanol and lactate, by redirecting electron flux to acetyl-CoA reduction.
The catabolic repressor-encoding genes crpZ, crp, hprK, hprY and hprX presented quite a different expression pattern depending on the carbon source (Fig. 5). Gene up-regulation is an early event for C. thermocellum grown on cotton waste and sugarcane straw in comparison to growth on cellulose as the carbon source (Fig. 5a, b, c). These results and the fact that these genes encode regulatory proteins with well-known roles in glycolytic pathway control strongly suggest that glycolysis is inhibited during the first 24 h on cotton waste and sugar cane straw.
This inhibition could be overcome after 34 h, when hydrolysis products produced by the action of cellulases and xylanases were directed to the glycolytic pathway and down-regulation of crpZ, crp, hprK, hprY and hprX was detected (Fig. 5a, b, c). It should be noted that for growth on cellulose the effect is the opposite (Fig. 5a). This may be because cellulose is a less recalcitrant substrate, being more easily degraded, and in this case glucose concentration increases promptly, increasing carbon flux into the glycolytic pathway and increasing the concentration of metabolites that could act as modulators of catabolic repression mechanisms in which crpZ, crp, hprK, hprY and hprX are involved. Therefore, d-glucose availability and substrate recalcitrance might be key factors controlling the expression levels of those genes and thereby ultimately influencing the carbon flux through the glycolytic pathway.
The present work raised questions about: (a) the effect of different carbon sources on C.thermocellum’s cellulosome architecture and secretome constitution; (b) expression control of cellulosomal protein-encoding genes and genes related to the global metabolism of C. thermocellum during growth in lignocellulosic biomasses; and (c) the effects of different pH and temperature levels on control of gene expression and protein secretion by C. thermocellum B8. These questions are a future focus for our research group.
Conclusions
Clostridium thermocellum B8 isolated from goat rumen was able to grow in the presence of microcrystalline cellulose, cotton waste and sugarcane straw, and produced free enzymes as well as enzymes bound to substrates (RSBP). RSBP is a cellulosome-enriched sample; therefore, the present work is the first report of cellulosome production by C. thermocellum during growth on cotton waste or sugar cane straw as the carbon source. Moreover, C. thermocellum B8 showed different expression levels of transcripts encoding cellulosomal proteins and proteins presenting a role in fermentation and catabolic repression. It also seems that pH and temperature are important factors controlling gene expression in this strain.
Acknowledgements
This work was supported by research grants from University of Brasilia—UnB, CAPES, CNPq, FINEP and FAPDF. Eliane F. Noronha is recipient of Brazilian Research Council’s (CNPq) research scholarship. The Embrapa Visiting Scientist Program supported B.F.Q. The authors would like to thank Robert Pogue PhD (Catholic University, Program of Genomic Sciences and Biotechnology, Brazil) for proofreading the manuscript.
References
Journal S, Statistics G, Sep N (2016) Interface Foundation of America R : A Language for Data Analysis and Graphics Author (s): Ross Ihaka and Robert Gentleman of Mathematical Statistics, and Interface Foundation of America Stable URL : http://www.jstor.org/stable/1390807 (Linked references. 5:299–314)