-
PDF
- Split View
-
Views
-
Cite
Cite
Tam Dinh Le Vo, Ji-seun Ko, Si Jae Park, Seung Hwan Lee, Soon Ho Hong, Efficient gamma-aminobutyric acid bioconversion by employing synthetic complex between glutamate decarboxylase and glutamate/GABA antiporter in engineered Escherichia coli, Journal of Industrial Microbiology and Biotechnology, Volume 40, Issue 8, 1 August 2013, Pages 927–933, https://doi.org/10.1007/s10295-013-1289-z
- Share Icon Share
Abstract
Gamma-aminobutyric acid (GABA) is a precursor of one of the most promising heat-resistant biopolymers, Nylon-4, and can be produced by the decarboxylation of monosodium glutamate (MSG). In this study, a synthetic protein complex was applied to improve the GABA conversion in engineered Escherichia coli. Complexes were constructed by assembling a single protein–protein interaction domain SH3 to the glutamate decarboxylase (GadA and GadB) and attaching a cognate peptide ligand to the glutamate/GABA antiporter (GadC) at the N-terminus, C-terminus, and the 233rd amino acid residue. When GadA and GadC were co-overexpressed via the C-terminus complex, a GABA concentration of 5.65 g/l was obtained from 10 g/l MSG, which corresponds to a GABA yield of 93 %. A significant increase of the GABA productivity was also observed where the GABA productivity increased 2.5-fold in the early culture period due to the introduction of the synthetic protein complex. The GABA pathway efficiency and GABA productivity were enhanced by the introduction of the complex between Gad and glutamate/GABA antiporter.
Introduction
Considering the limited supply and elevating costs of fossil resources, biomass-based production of energy carriers, chemicals, and polymers is becoming a new paradigm in biotechnology research. To replace the need for polystyrene or polyethylene-based plastics, several biomass-based polymers such as polylactic acid, poly-3-hydroxybutyrate, and poly(butylenes succinate) have been intensively studied. Another promising bio-based biodegradable polymer is polyamide 4, also known as Nylon-4, which is composed of a repeating gamma-aminobutyric acid (GABA) unit [9].
In Escherichia coli, the GABA pathway, which is constituted with glutamate decarboxylase (Gad) and glutamate/GABA antiporter, controls the acidification in the cytosolic environment through decarboxylating an acidic substrate (glutamate) into a neutral compound (GABA) [3, 8]. Glutamate decarboxylase (GadA and GadB) catalyzes the irreversible decarboxylation of L-glutamate to GABA. GABA is exported into the extracellular medium and glutamate is brought into the cell by glutamate/GABA antiporter (GadC), contributing to local alkalization of the extracellular environment [1].
Considering the industrial importance of GABA, various studies have been conducted to improve GABA production in recombinant bacteria. By the overexpression of rice Gad in Bifidobacterium longum, 0.1 g/l GABA was produced from 30 g/l monosodium glutamate (MSG) [7]. When Lactobacillus brevis Gad was overexpressed in Bacillus subtilis, 0.4 g/l GABA was produced from 30 g/l MSG [8]. It was also reported that overexpression of Gad from Lactobacillus plantarum ATCC 14917 in Lactobacillus sakei B2-16 elevated the final GABA concentration 1.4-fold [4]. When E. coli Gad and glutamate/GABA antiporter were co-overexpressed in a GABA aminotransferase knockout E. coli strain, a final GABA concentration of 5.46 g/l was obtained from 10 g/l MSG, which corresponds to a GABA yield of 89.5 % [10].
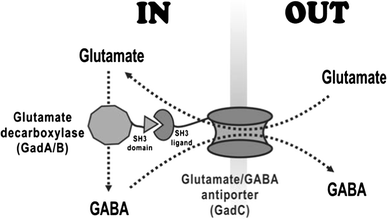
Schematic diagram of GABA conversion in recombinant E. coli. Glutamate decarboxylase (GadA/GadB) and the glutamate/GABA antiporter (GadC) were overexpressed
Materials and methods
Bacterial strains, plasmids, and medium
The bacterial strains and plasmids used in this study are listed in Table 1. E. coli XL1-Blue (XB) was used as the host strain. pGEMT vector (Promega, Madison, WI, USA) was used for DNA cloning and pMAL-p4X (New England Biolabs, Ipswich, MA, USA) was used to construct the expression plasmids. Chemically competent cells were prepared using a standard procedure and were stored at −80 °C until needed.
List of bacterial strains and plasmids used in this study
Strain, plasmid . | Genotype and/or property . | Reference . |
---|---|---|
Escherichia coli strains | ||
XL1-Blue | SupE44 hsdR17 recA1 endA1 gyrA96 thi relA1 lacF’[proAB+lacIqlacZΔM15 Tn10 (tetR)] | Laboratory stock |
Plasmid | ||
pGEMT | ApR | Promega (USA) |
pMAL-p4X | ApR | New England Biolabs (USA) |
pJD758 | Moon et al. [6] | |
pHAC | pMAL-p4X containing gadA and gadC | Vo et al. [10] |
pHBC | pMAL-p4X containing gadB and gadC | Vo et al. [10] |
pHAD | pMAL-p4X containing gadA-SH3D | This work |
pHBD | pMAL-p4X containing gadB-SH3D | This work |
pH1AC | pMAL-p4X containing gadA-SH3D and gadC-SH3L, N-terminal model | This work |
pH1BC | pMAL-p4X containing gadB-SH3D and gadC-SH3L, N-terminal model | This work |
pH2AC | pMAL-p4X containing gadA-SH3D and gadC-SH3L, sandwich model | This work |
pH2BC | pMAL-p4X containing gadB-SH3D and gadC-SH3L, sandwich model | This work |
pH3AC | pMAL-p4X containing gadA-SH3D and gadC-SH3L, C-terminal model | This work |
pH3BC | pMAL-p4X containing gadB-SH3D and gadC-SH3L, C-terminal model | This work |
Strain, plasmid . | Genotype and/or property . | Reference . |
---|---|---|
Escherichia coli strains | ||
XL1-Blue | SupE44 hsdR17 recA1 endA1 gyrA96 thi relA1 lacF’[proAB+lacIqlacZΔM15 Tn10 (tetR)] | Laboratory stock |
Plasmid | ||
pGEMT | ApR | Promega (USA) |
pMAL-p4X | ApR | New England Biolabs (USA) |
pJD758 | Moon et al. [6] | |
pHAC | pMAL-p4X containing gadA and gadC | Vo et al. [10] |
pHBC | pMAL-p4X containing gadB and gadC | Vo et al. [10] |
pHAD | pMAL-p4X containing gadA-SH3D | This work |
pHBD | pMAL-p4X containing gadB-SH3D | This work |
pH1AC | pMAL-p4X containing gadA-SH3D and gadC-SH3L, N-terminal model | This work |
pH1BC | pMAL-p4X containing gadB-SH3D and gadC-SH3L, N-terminal model | This work |
pH2AC | pMAL-p4X containing gadA-SH3D and gadC-SH3L, sandwich model | This work |
pH2BC | pMAL-p4X containing gadB-SH3D and gadC-SH3L, sandwich model | This work |
pH3AC | pMAL-p4X containing gadA-SH3D and gadC-SH3L, C-terminal model | This work |
pH3BC | pMAL-p4X containing gadB-SH3D and gadC-SH3L, C-terminal model | This work |
List of bacterial strains and plasmids used in this study
Strain, plasmid . | Genotype and/or property . | Reference . |
---|---|---|
Escherichia coli strains | ||
XL1-Blue | SupE44 hsdR17 recA1 endA1 gyrA96 thi relA1 lacF’[proAB+lacIqlacZΔM15 Tn10 (tetR)] | Laboratory stock |
Plasmid | ||
pGEMT | ApR | Promega (USA) |
pMAL-p4X | ApR | New England Biolabs (USA) |
pJD758 | Moon et al. [6] | |
pHAC | pMAL-p4X containing gadA and gadC | Vo et al. [10] |
pHBC | pMAL-p4X containing gadB and gadC | Vo et al. [10] |
pHAD | pMAL-p4X containing gadA-SH3D | This work |
pHBD | pMAL-p4X containing gadB-SH3D | This work |
pH1AC | pMAL-p4X containing gadA-SH3D and gadC-SH3L, N-terminal model | This work |
pH1BC | pMAL-p4X containing gadB-SH3D and gadC-SH3L, N-terminal model | This work |
pH2AC | pMAL-p4X containing gadA-SH3D and gadC-SH3L, sandwich model | This work |
pH2BC | pMAL-p4X containing gadB-SH3D and gadC-SH3L, sandwich model | This work |
pH3AC | pMAL-p4X containing gadA-SH3D and gadC-SH3L, C-terminal model | This work |
pH3BC | pMAL-p4X containing gadB-SH3D and gadC-SH3L, C-terminal model | This work |
Strain, plasmid . | Genotype and/or property . | Reference . |
---|---|---|
Escherichia coli strains | ||
XL1-Blue | SupE44 hsdR17 recA1 endA1 gyrA96 thi relA1 lacF’[proAB+lacIqlacZΔM15 Tn10 (tetR)] | Laboratory stock |
Plasmid | ||
pGEMT | ApR | Promega (USA) |
pMAL-p4X | ApR | New England Biolabs (USA) |
pJD758 | Moon et al. [6] | |
pHAC | pMAL-p4X containing gadA and gadC | Vo et al. [10] |
pHBC | pMAL-p4X containing gadB and gadC | Vo et al. [10] |
pHAD | pMAL-p4X containing gadA-SH3D | This work |
pHBD | pMAL-p4X containing gadB-SH3D | This work |
pH1AC | pMAL-p4X containing gadA-SH3D and gadC-SH3L, N-terminal model | This work |
pH1BC | pMAL-p4X containing gadB-SH3D and gadC-SH3L, N-terminal model | This work |
pH2AC | pMAL-p4X containing gadA-SH3D and gadC-SH3L, sandwich model | This work |
pH2BC | pMAL-p4X containing gadB-SH3D and gadC-SH3L, sandwich model | This work |
pH3AC | pMAL-p4X containing gadA-SH3D and gadC-SH3L, C-terminal model | This work |
pH3BC | pMAL-p4X containing gadB-SH3D and gadC-SH3L, C-terminal model | This work |
E. coli strains were cultured at 37 °C and 250 rpm in Luria–Bertani (LB) broth (10 g/l bacto-tryptone, 5 g/l bacto-yeast extract, and 5 g/l NaCl) supplemented with 100 μg/ml ampicillin sodium salt for the selection of transformants harboring recombinant plasmids.
Cloning of gad genes
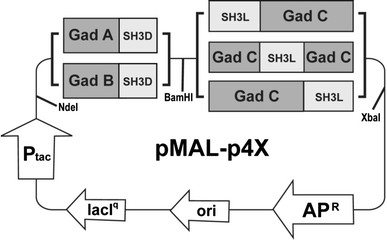
Schematic maps of gadABC complex plasmids. Two glutamate decarboxylase (GadA and GadB) and three glutamate/GABA antiporter-SH3 ligand models were tested in combination
Primers used for PCR in this study
Primer . | Sequence . | Gene . |
---|---|---|
Forward | 5′-GGAATTCCATATGATGGACCAGAAGCTGTTAA-3′ | gadA |
Reverse | 5′-GGGCCCGCACATACTCTGGCTGCCGCCGCTGCCGCTGCCGCTGCCGGTGTGTTTAAAGCTGTTCTG-3′ | |
Forward | 5′-GGAATTCCATATGATGGATAAGAAGCAAGTAACG-3′ | gadB |
Reverse | 5′-GGGCCCGCACATACTCTGGCTGCCGCCGCTGCCGCTGCCGCTGCCGGTATGTTTAAAGCTGTTCT-3′ | |
Forward | 5′-ATGGCTACATCAGTACAGA-3′ | gadC |
Reverse | 5′-GCTCTAGATTAGTGTTTCTTGTCATTCATC-3′ | |
Forward | 5′-CAGAGTATGTGCGGGCCC-3′ | SH3 domain |
Reverse | 5′-CGCGGATCCTTAATACTTCTCCACGTAAGG-3′ | |
Forward | 5′-CGGGATCCTTCACCAACAAGGACCATAGCATGCTGGAAGGCTCTGGCT-3′ | SH3 ligand |
Reverse | 5′-TCTGTACTGATGTAGCCATGCCGCTGCCGCTGCCGCCGCCGCTGCCTTCCAGGCCCGGACGACGACGT-3′ |
Primer . | Sequence . | Gene . |
---|---|---|
Forward | 5′-GGAATTCCATATGATGGACCAGAAGCTGTTAA-3′ | gadA |
Reverse | 5′-GGGCCCGCACATACTCTGGCTGCCGCCGCTGCCGCTGCCGCTGCCGGTGTGTTTAAAGCTGTTCTG-3′ | |
Forward | 5′-GGAATTCCATATGATGGATAAGAAGCAAGTAACG-3′ | gadB |
Reverse | 5′-GGGCCCGCACATACTCTGGCTGCCGCCGCTGCCGCTGCCGCTGCCGGTATGTTTAAAGCTGTTCT-3′ | |
Forward | 5′-ATGGCTACATCAGTACAGA-3′ | gadC |
Reverse | 5′-GCTCTAGATTAGTGTTTCTTGTCATTCATC-3′ | |
Forward | 5′-CAGAGTATGTGCGGGCCC-3′ | SH3 domain |
Reverse | 5′-CGCGGATCCTTAATACTTCTCCACGTAAGG-3′ | |
Forward | 5′-CGGGATCCTTCACCAACAAGGACCATAGCATGCTGGAAGGCTCTGGCT-3′ | SH3 ligand |
Reverse | 5′-TCTGTACTGATGTAGCCATGCCGCTGCCGCTGCCGCCGCCGCTGCCTTCCAGGCCCGGACGACGACGT-3′ |
Primers used for PCR in this study
Primer . | Sequence . | Gene . |
---|---|---|
Forward | 5′-GGAATTCCATATGATGGACCAGAAGCTGTTAA-3′ | gadA |
Reverse | 5′-GGGCCCGCACATACTCTGGCTGCCGCCGCTGCCGCTGCCGCTGCCGGTGTGTTTAAAGCTGTTCTG-3′ | |
Forward | 5′-GGAATTCCATATGATGGATAAGAAGCAAGTAACG-3′ | gadB |
Reverse | 5′-GGGCCCGCACATACTCTGGCTGCCGCCGCTGCCGCTGCCGCTGCCGGTATGTTTAAAGCTGTTCT-3′ | |
Forward | 5′-ATGGCTACATCAGTACAGA-3′ | gadC |
Reverse | 5′-GCTCTAGATTAGTGTTTCTTGTCATTCATC-3′ | |
Forward | 5′-CAGAGTATGTGCGGGCCC-3′ | SH3 domain |
Reverse | 5′-CGCGGATCCTTAATACTTCTCCACGTAAGG-3′ | |
Forward | 5′-CGGGATCCTTCACCAACAAGGACCATAGCATGCTGGAAGGCTCTGGCT-3′ | SH3 ligand |
Reverse | 5′-TCTGTACTGATGTAGCCATGCCGCTGCCGCTGCCGCCGCCGCTGCCTTCCAGGCCCGGACGACGACGT-3′ |
Primer . | Sequence . | Gene . |
---|---|---|
Forward | 5′-GGAATTCCATATGATGGACCAGAAGCTGTTAA-3′ | gadA |
Reverse | 5′-GGGCCCGCACATACTCTGGCTGCCGCCGCTGCCGCTGCCGCTGCCGGTGTGTTTAAAGCTGTTCTG-3′ | |
Forward | 5′-GGAATTCCATATGATGGATAAGAAGCAAGTAACG-3′ | gadB |
Reverse | 5′-GGGCCCGCACATACTCTGGCTGCCGCCGCTGCCGCTGCCGCTGCCGGTATGTTTAAAGCTGTTCT-3′ | |
Forward | 5′-ATGGCTACATCAGTACAGA-3′ | gadC |
Reverse | 5′-GCTCTAGATTAGTGTTTCTTGTCATTCATC-3′ | |
Forward | 5′-CAGAGTATGTGCGGGCCC-3′ | SH3 domain |
Reverse | 5′-CGCGGATCCTTAATACTTCTCCACGTAAGG-3′ | |
Forward | 5′-CGGGATCCTTCACCAACAAGGACCATAGCATGCTGGAAGGCTCTGGCT-3′ | SH3 ligand |
Reverse | 5′-TCTGTACTGATGTAGCCATGCCGCTGCCGCTGCCGCCGCCGCTGCCTTCCAGGCCCGGACGACGACGT-3′ |
GABA bioconversion and analysis
Recombinant strains were cultivated in 250 ml flasks in 100 ml LB (100 μg/ml ampicillin added) containing 10 g/l MSG at 37 °C and 250 rpm. When the OD600 reached 1.2, gene expression was induced with 0.5 mM IPTG. Then, the pH was adjusted to 3.5 and the strain was incubated at 30 °C and 250 rpm for 48 h.
The GABA bioconversions were quantitatively analyzed by HPLC using an OptimaPak C18 column (4.6 × 150 mm) (RS tech Corporation, Daejeon, Korea). The sample preparation was performed as follows. Samples were centrifuged at 12,000 rpm for 5 min and then 100 μl of the supernatant was added to an Eppendorf tube. Next, 200 μl of a 1 M sodium bicarbonate buffer at a pH of 9.8, 100 μl of 80 g/l dansyl chloride in acetonitrile, and 600 μl of double-distilled water were added to make a 1 ml reaction mixture. The mixture was then incubated at 80 °C for 40 min. Subsequently, 100 μl of 20 μl/ml acetic acid was added to stop the reaction. The mixture was then centrifuged at 12,000 rpm for 5 min. Next, the supernatant was filtered through a 0.22 μm Millipore filter and analyzed by HPLC on an Agilent system using UV detection. Separation of the derivatized samples was performed using a binary non-linear gradient with eluant A [tetrahydrofuran/methanol/50 mM sodium acetate with a pH of 6.2 (5:75:420, by volume)] and eluant B (methanol). The column temperature was set at 30 °C and the elution conditions were as follows: equilibration (6 min, 20 % B), gradient (20 min, 20–80 % B), and cleaning (3 min, 100 % B). The flow rate of the mobile phase was 1 ml/min and the samples were detected at a wavelength of 286 nm in the UV region. The standard curve for GABA was generated from 10 standard solutions (1, 2, 3, 4, 5, 6, 7, 8, 9, and 10 g/l GABA) (Sigma, St. Louis, MO, USA) using the same procedure.
Results
Plasmid construction, expression of gad genes, and complex
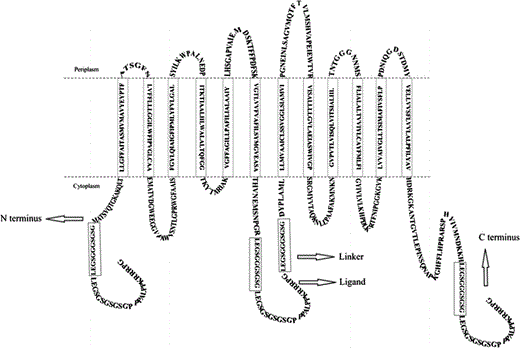
Fusion models of the glutamate/GABA antiporter (GadC) with a SH3 ligand via an 11-amino-acid linker (in box) at three positions: N-terminus, the 233rd amino acid residue, and C-terminus
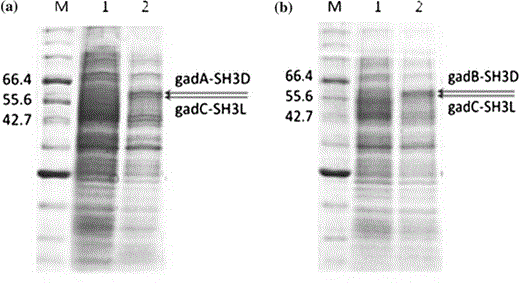
Results of the SDS-PAGE analysis of the overexpression of gad genes and complex device where M is the marker protein, lane 1 is the cell extract of the untransformed strain, and lane 2 is the cell extract of strains harboring a pH1AC (GadA-SH3D and GadC-SH3L) and b pH1BC (GadB-SH3D and GadC-SH3L). (GadA-SH3D: 60.12 kDa, GadB-SH3D: 60.1 kDa, and GadC-SH3L: 58.36 kDa)
GABA bioconversion
The results of the GABA conversion from MSG by various recombinant strains containing GadABC synthetic complex are summarized in Table 3. When GadA/B and GadC were not overexpressed, GABA was not produced (data not shown). When normal Gad and glutamate/GABA antiporter were overexpressed without a synthetic complex, about 4.5 g/l GABA was produced from 10 g/l MSG. An increase of the final GABA concentration was observed regardless of the type of complex when a synthetic complex was introduced to GadB and GadC. By introduction of the N-terminus synthetic complex to GadA and GadC, the final GABA concentration increased to 4.9 g/l. The maximum GABA concentration of 5.65 g/l was obtained with the C-terminus synthetic complex of GadA and GadC, which is 30 % higher than that obtained with normal GadA and GadC (Table 3). Based on the higher GABA concentration, it can be deduced that more glutamate, which can be used for other metabolites, was converted to GABA by complex of Gad and glutamate/GABA antiporter.
Final GABA concentrations (g/l) obtained from the conversion
Enzymes . | Complex type . | |||
---|---|---|---|---|
w/o complex . | N-terminus . | Sandwich . | C-terminus . | |
GadA and GadC | 4.34 ± 0.17 | 4.94 ± 0.37 | 5.06 ± 0.76 | 5.65 ± 0.04 |
GadB and GadC | 4.51 ± 0.61 | 4.84 ± 0.38 | 4.74 ± 0.25 | 4.65 ± 0.75 |
Enzymes . | Complex type . | |||
---|---|---|---|---|
w/o complex . | N-terminus . | Sandwich . | C-terminus . | |
GadA and GadC | 4.34 ± 0.17 | 4.94 ± 0.37 | 5.06 ± 0.76 | 5.65 ± 0.04 |
GadB and GadC | 4.51 ± 0.61 | 4.84 ± 0.38 | 4.74 ± 0.25 | 4.65 ± 0.75 |
Final GABA concentrations (g/l) obtained from the conversion
Enzymes . | Complex type . | |||
---|---|---|---|---|
w/o complex . | N-terminus . | Sandwich . | C-terminus . | |
GadA and GadC | 4.34 ± 0.17 | 4.94 ± 0.37 | 5.06 ± 0.76 | 5.65 ± 0.04 |
GadB and GadC | 4.51 ± 0.61 | 4.84 ± 0.38 | 4.74 ± 0.25 | 4.65 ± 0.75 |
Enzymes . | Complex type . | |||
---|---|---|---|---|
w/o complex . | N-terminus . | Sandwich . | C-terminus . | |
GadA and GadC | 4.34 ± 0.17 | 4.94 ± 0.37 | 5.06 ± 0.76 | 5.65 ± 0.04 |
GadB and GadC | 4.51 ± 0.61 | 4.84 ± 0.38 | 4.74 ± 0.25 | 4.65 ± 0.75 |
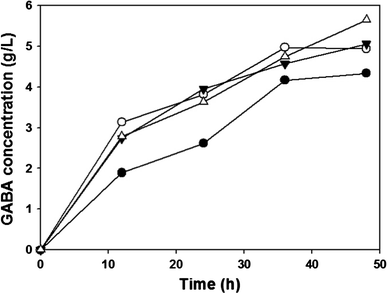
Time profiles of the GABA concentration obtained with and without GadA and GadC complexes: XB (pHAC) (filled circle, without complex), XB (pH1AC) (unfilled circle, N-terminus complex), XB (pH2AC) (inverted triangle, Sandwich complex), and XB (pH3AC) (unfilled square, C-terminus complex)
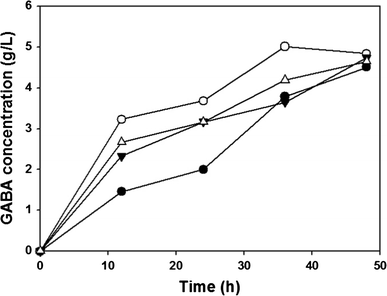
Time profiles of the GABA concentration obtained with and without GadB and GadC complexes: XB (pHBC) (filled circle, without complex), XB (pH1BC) (unfilled circle, N-terminus complex), XB (pH2BC) (inverted triangle, Sandwich complex), and XB (pH3BC) (unfilled square, C-terminus complex)
Produced GABA has two fates as it can either be directed to the TCA cycle by the function of the GABA aminotransferase and succinate-semialdehyde dehydrogenase or secreted to the media via GadC. To see if GABA was directed back to the TCA cycle by GABA aminotransferase (GabT), GabT knock-out E. coli strains (XBT) harboring pH1AC and pH1BC were tested. The knock-out E. coli XBT (pH1AC) and XBT (pH1BC) strains produced lower amounts of GABA when compared to normal E. coli strains harboring the same plasmids (data not shown). This result suggests that produced GABA is not redirected to TCA cycle by GabT and knock-out of GabT has little effect on GABA production.
Discussion
In this study, glutamate decarboxylase (GadA, GadB) and a glutamate/GABA antiporter (GadC) were co-overexpressed with a synthetic protein complex to improve the GABA production. When GadA and GadC were bound by a C-terminus complex, a GABA concentration of 5.65 g/l was obtained from 10 g/l MSG, which corresponds to a GABA yield of 92.6 %. The GABA productivity increased 2.5-fold in the early culture period by the introduction of a synthetic protein complex between the Gad and glutamate/GABA antiporter.
These results demonstrate the practical benefit of complex which results in improved pathway efficiency. Besides the increase of the final GABA concentration, the introduction of a synthetic protein complex resulted in a huge increase of the GABA productivity, especially in the early culture period. The higher productivity may be due to a shortened GABA reaction path. In the normal strain, once glutamate is imported into the cytoplasm via the glutamate/GABA antiporter, it has to travel all around the cytoplasm until it meets Gad to be converted to GABA. Then, GABA goes to the glutamate/GABA antiporter and is secreted to the media. With the introduction of a protein complex, however, Gad and glutamate/GABA antiporter were closely bound. Imported glutamate is immediately converted to GABA by Gad which is located right next to the glutamate/GABA antiporter via the protein complex. Then, GABA will be secreted via the nearby glutamate/GABA antiporter. Therefore, glutamate can be more effectively converted to GABA by the introduction of a synthetic complex between Gad and the glutamate/GABA antiporter, resulting in a higher GABA productivity.
A decrease of GAGA productivity was observed at the later culture period. At the early culture period, MSG can be efficiently converted to GABA via combined Gad and the glutamate/GABA antiporter, and high GABA productivity can be achieved. At the later culture period, however, MSG concentration became low, and it led to low GABA productivity. Especially, about 93 % of GABA yield was achieved in our system which suggested that only little MSG left at later culture period, and GABA productivity became low.
Considering the efficiency and cost-effectiveness of the GABA production process, productivity is one of the key factors as well as the final GABA concentration, as a high productivity results in a shorter processing time and lower production costs. Our study clearly shows that the pathway efficiency and productivity can be improved by the introduction of a synthetic protein complex. We believe that the synthetic complex strategy can be applied to other metabolite producing systems besides GABA to result in improved productivity and process efficiency. Thus, the results of this study will contribute to the ongoing discovery of new strategies for creating high-yield GABA producing strains.
Acknowledgments
This work was supported by a grant from the Next-Generation BioGreen 21 Program (SSAC, Grant Number: PJ00954904), Rural Development Administration, Republic of Korea.