-
PDF
- Split View
-
Views
-
Cite
Cite
Shaowen Wang, Gang Liu, Juan Wang, Jianteng Yu, Baiqu Huang, Miao Xing, Enhancing cellulase production in Trichoderma reesei RUT C30 through combined manipulation of activating and repressing genes, Journal of Industrial Microbiology and Biotechnology, Volume 40, Issue 6, 1 June 2013, Pages 633–641, https://doi.org/10.1007/s10295-013-1253-y
- Share Icon Share
Abstract
To investigate whether enzyme production can be enhanced in the Trichoderma reesei industrial hyperproducer strain RUT C30 by manipulation of cellulase regulation, the positive regulator Xyr1 was constitutively expressed under the control of the strong T. reesei pdc promoter, resulting in significantly enhanced cellulase activity in the transformant during growth on cellulose. In addition, constitutive expression of xyr1 combined with downregulation of the negative regulator encoding gene ace1 further increased cellulase and xylanase activities. Compared with RUT C30, the resulting transformant exhibited 103, 114, and 134 % greater total secreted protein levels, filter paper activity, and CMCase activity, respectively. Surprisingly, strong increases in xyr1 basal expression levels resulted in very high levels of CMCase activity during growth on glucose. These findings demonstrate the feasibility of improving cellulase production by modifying regulator expression, and suggest an attractive new single-step approach for increasing total cellulase productivity in T. reesei.
Introduction
Trichoderma reesei (anamorph of Hypocrea jecorina) is one of the most efficient producers of cellulolytic and xylanolytic enzymes used in a broad range of industrial applications, including the textile, food and feed, and pulp and paper industries [4]. Owing to increasing production demand for biofuels from lignocellulose, current research on T. reesei is focused on improving cellulase production, with the aim of reducing the relatively high cost of this process [7]. Strain improvement has previously been achieved using classical mutagenesis and screening procedures [18]. The availability of sophisticated gene manipulation methods and recent sequencing of the T. reesei genome [14] have raised the possibility of introducing molecular genetic methods into such improvement programs—for example, by upregulating activators and/or downregulating repressors of the cellulase genes. To accomplish this goal, however, a sound understanding of cellulase regulation is essential.
The major cellulase and hemicellulase genes in T. reesei are regulated in a coordinated manner by available carbon sources [8, 13]. Cellulose, lactose, and other materials induce expression of cellulase and hemicellulase genes, while glucose acts as a repressing carbon source. Several genes encoding regulators of cellulase and hemicellulase expression have been isolated. These regulators include the carbon catabolite repressor Cre1 [26], the repressor Ace1 [22], the activator Ace2 [2], the CCAAT binding complex Hap2/3/5 [30], and the activator Xyr1 [21]. In addition, the T. reesei protein methyltransferase LAE1 was recently shown to control cellulase gene expression [24]. The regulator Xyr1 is an ortholog of Aspergillus niger XlnR [31], a member of the zinc binuclear cluster protein family that binds with both the 5′-GGCTAA-3′ motif and several common 5′-GGC(A/T)3-3′ motifs found in the 5′-upstream region of Xyr1-regulated genes [5]. Xyr1 is a general and essential transcription factor controlling expression of the major cellulolytic and xylanolytic genes, regardless of inducer (xylose, xylobiose, sophorose, and lactose) and mode of expression (basal, derepression, and induction) [16, 27]. Mach-Aigner et al. [12] have constructed a recombinant strain expressing the xyr1 gene under control of the T. atroviride nag1 (N-acetyl-β-d-glucosaminidase-encoding) promoter. In this strain, a slightly earlier beginning of xylanase formation and altered transcription profile of genes encoding for enzymes associated with degradation of (hemi)celluloses were observed [12, 20]. However, the promoter of nag1 they used is not much stronger than the native xyr1 promoter, and thus the final enzyme activity in this strain was not significantly enhanced [10]. On the other hand, Seiboth et al. [24] overexpressed the xyr1 gene under the control of the constitutive pki1 promoter in QM9414, which resulted in increased cellulase formation. However, the efficiency of the pki1 promoter is still low compared with strong constitutive promoters, such as the T. reesei pdc gene (encoding pyruvate decarboxylase), which was recently identified and used to generate very high expression levels of recombinant xylanase II [11]. It is unknown whether an increase in constitutive expression of xyr1 could further improve enzyme production in and industrial production strain improved greatly by mutagenesis and screening programs.
The mutant strain T. reesei RUT C30, developed using several rounds of random mutagenesis, exhibits outstanding levels of protein secretion and high cellulolytic activity [18]. In our study, constitutive xyr1 expression under the control of the strong constitutive pdc promoter was introduced into RUT C30 to investigate whether increased xyr1 expression can enhance enzyme production in this industrial production strain. To further improve its cellulase production ability, xyr1 overexpression and RNAi-mediated gene silencing of the cellulase repressor-encoding gene ace1 were simultaneously introduced into RUT C30.
Materials and methods
Strains and culture conditions
Escherichia coli Top10F’ (Invitrogen, Carlsbad, CA, USA) was used as the host strain for recombinant plasmid construction. The hypercellulolytic mutant strain T. reesei RUT C30 (ATCC 56756), used as a parent strain throughout this study, was maintained on potato dextrose agar (PDA). To study cellulase and xylanase gene expression in response to different carbon sources, mycelia were precultured on a rotary shaker (220 rpm) at 28 °C for 48 h in 250-ml Erlenmeyer flasks containing 50 ml of MM medium [8] with 1 % (w/v) glycerol as a carbon source. Conidia at a final concentration of 1 × 108 l−1 were used as inoculum. Pre-grown mycelia were washed, and equal amounts were then resuspended in MM medium containing either 2 % (w/v) glycerol, glucose, or cellulose (Avicel PH-105, FMC BioPolymer, Philadelphia, PA, USA) as carbon sources. Incubation was continued for 8 and 48 h; mycelia were then harvested by filtration, washed twice with distilled water, and used for RNA extraction.
Construction of the Ppdc-xyr1-Tpdc cassette
Primers used for construction of the xyr1 expression cassette and RNAi vector
Primers . | Sequences (5′–3′)a . | Notes . |
---|---|---|
Ppdc-F | ATGAAAGGAGGGAGCATTCTTCGAC | For construction of the xyr1 expression cassette |
Ppdc-R | AGCGACGGAGAGGATTGGACAACATGATTGTGCTGTAGCTGCGCTGCTTT | |
xyr-F | AAAGCAGCGCAGCTACAGCACAATCATGTTGTCCAATCCTCTCCGTCGCT | |
xyr-R | CTACCCGGTCAGACTTCATGCCGGGTTAGAGGGCCAGACCGGTTCCGTTA | |
Tpdc-F | TAACGGAACCGGTCTGGCCCTCTAACCCGGCATGAAGTCTGACCGG | |
Tpdc-R | TTGCCTTTTGAGCTGGCGTCTT | |
Ppa-F | CGGGGTACCATGAAAGGAGGGAGCATTCTTCGAC | For construction of the RNAi vector for silencing the ace1 gene |
Ppa-R | CCGTAGGTAGCGAGCCAATCGATTGTGCTGTAGCTGCGCTGCTTT | |
aces-F | CAGCGCAGCTACAGCACAATCGATTGGCTCGCTACCTACGG | |
aces-R | TACAGCCAAAGTCAAAACCCGTTGAAGATGTCGGGCTGTG | |
it-F | CACAGCCCGACATCTTCAACGGGTTTTGACTTTGGCTGTA | |
it-R | CTAGTCTAGAGTTCTTCAACGGAGGATAAA | |
acea-F | CTAGTCTAGAGTTGAAGATGTCGGGCTGTG | |
acea-R | CCGGTCAGACTTCATGCCGGGGATTGGCTCGCTACCTACGG | |
Tpa-F | CCGTAGGTAGCGAGCCAATCCCCGGCATGAAGTCTGACCGG | |
Tpa-R | ACCCAAGCTTTTGCCTTTTGAGCTGGCGTCTT |
Primers . | Sequences (5′–3′)a . | Notes . |
---|---|---|
Ppdc-F | ATGAAAGGAGGGAGCATTCTTCGAC | For construction of the xyr1 expression cassette |
Ppdc-R | AGCGACGGAGAGGATTGGACAACATGATTGTGCTGTAGCTGCGCTGCTTT | |
xyr-F | AAAGCAGCGCAGCTACAGCACAATCATGTTGTCCAATCCTCTCCGTCGCT | |
xyr-R | CTACCCGGTCAGACTTCATGCCGGGTTAGAGGGCCAGACCGGTTCCGTTA | |
Tpdc-F | TAACGGAACCGGTCTGGCCCTCTAACCCGGCATGAAGTCTGACCGG | |
Tpdc-R | TTGCCTTTTGAGCTGGCGTCTT | |
Ppa-F | CGGGGTACCATGAAAGGAGGGAGCATTCTTCGAC | For construction of the RNAi vector for silencing the ace1 gene |
Ppa-R | CCGTAGGTAGCGAGCCAATCGATTGTGCTGTAGCTGCGCTGCTTT | |
aces-F | CAGCGCAGCTACAGCACAATCGATTGGCTCGCTACCTACGG | |
aces-R | TACAGCCAAAGTCAAAACCCGTTGAAGATGTCGGGCTGTG | |
it-F | CACAGCCCGACATCTTCAACGGGTTTTGACTTTGGCTGTA | |
it-R | CTAGTCTAGAGTTCTTCAACGGAGGATAAA | |
acea-F | CTAGTCTAGAGTTGAAGATGTCGGGCTGTG | |
acea-R | CCGGTCAGACTTCATGCCGGGGATTGGCTCGCTACCTACGG | |
Tpa-F | CCGTAGGTAGCGAGCCAATCCCCGGCATGAAGTCTGACCGG | |
Tpa-R | ACCCAAGCTTTTGCCTTTTGAGCTGGCGTCTT |
aRestriction sites are underlined; overlapping regions in primers are double underlined
Primers used for construction of the xyr1 expression cassette and RNAi vector
Primers . | Sequences (5′–3′)a . | Notes . |
---|---|---|
Ppdc-F | ATGAAAGGAGGGAGCATTCTTCGAC | For construction of the xyr1 expression cassette |
Ppdc-R | AGCGACGGAGAGGATTGGACAACATGATTGTGCTGTAGCTGCGCTGCTTT | |
xyr-F | AAAGCAGCGCAGCTACAGCACAATCATGTTGTCCAATCCTCTCCGTCGCT | |
xyr-R | CTACCCGGTCAGACTTCATGCCGGGTTAGAGGGCCAGACCGGTTCCGTTA | |
Tpdc-F | TAACGGAACCGGTCTGGCCCTCTAACCCGGCATGAAGTCTGACCGG | |
Tpdc-R | TTGCCTTTTGAGCTGGCGTCTT | |
Ppa-F | CGGGGTACCATGAAAGGAGGGAGCATTCTTCGAC | For construction of the RNAi vector for silencing the ace1 gene |
Ppa-R | CCGTAGGTAGCGAGCCAATCGATTGTGCTGTAGCTGCGCTGCTTT | |
aces-F | CAGCGCAGCTACAGCACAATCGATTGGCTCGCTACCTACGG | |
aces-R | TACAGCCAAAGTCAAAACCCGTTGAAGATGTCGGGCTGTG | |
it-F | CACAGCCCGACATCTTCAACGGGTTTTGACTTTGGCTGTA | |
it-R | CTAGTCTAGAGTTCTTCAACGGAGGATAAA | |
acea-F | CTAGTCTAGAGTTGAAGATGTCGGGCTGTG | |
acea-R | CCGGTCAGACTTCATGCCGGGGATTGGCTCGCTACCTACGG | |
Tpa-F | CCGTAGGTAGCGAGCCAATCCCCGGCATGAAGTCTGACCGG | |
Tpa-R | ACCCAAGCTTTTGCCTTTTGAGCTGGCGTCTT |
Primers . | Sequences (5′–3′)a . | Notes . |
---|---|---|
Ppdc-F | ATGAAAGGAGGGAGCATTCTTCGAC | For construction of the xyr1 expression cassette |
Ppdc-R | AGCGACGGAGAGGATTGGACAACATGATTGTGCTGTAGCTGCGCTGCTTT | |
xyr-F | AAAGCAGCGCAGCTACAGCACAATCATGTTGTCCAATCCTCTCCGTCGCT | |
xyr-R | CTACCCGGTCAGACTTCATGCCGGGTTAGAGGGCCAGACCGGTTCCGTTA | |
Tpdc-F | TAACGGAACCGGTCTGGCCCTCTAACCCGGCATGAAGTCTGACCGG | |
Tpdc-R | TTGCCTTTTGAGCTGGCGTCTT | |
Ppa-F | CGGGGTACCATGAAAGGAGGGAGCATTCTTCGAC | For construction of the RNAi vector for silencing the ace1 gene |
Ppa-R | CCGTAGGTAGCGAGCCAATCGATTGTGCTGTAGCTGCGCTGCTTT | |
aces-F | CAGCGCAGCTACAGCACAATCGATTGGCTCGCTACCTACGG | |
aces-R | TACAGCCAAAGTCAAAACCCGTTGAAGATGTCGGGCTGTG | |
it-F | CACAGCCCGACATCTTCAACGGGTTTTGACTTTGGCTGTA | |
it-R | CTAGTCTAGAGTTCTTCAACGGAGGATAAA | |
acea-F | CTAGTCTAGAGTTGAAGATGTCGGGCTGTG | |
acea-R | CCGGTCAGACTTCATGCCGGGGATTGGCTCGCTACCTACGG | |
Tpa-F | CCGTAGGTAGCGAGCCAATCCCCGGCATGAAGTCTGACCGG | |
Tpa-R | ACCCAAGCTTTTGCCTTTTGAGCTGGCGTCTT |
aRestriction sites are underlined; overlapping regions in primers are double underlined
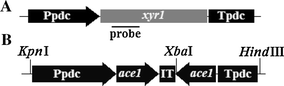
Schematic representation of the xyr1 expression cassette (a) and RNAi vector (b). Ppdc: Trichoderma reesei pdc promoter; ace1 (right arrow): 507-bp ace1 coding sequence; IT: 249-bp spacer fragment including intron 2 of the T. reesei eg2 gene; ace1 (left arrow): antisense strand of the 507-bp ace1 coding sequence; Tpdc: T. reesei pdc terminator. The line indicates the probe for Southern blot
Construction of the RNAi vector
The RNAi vector for silencing the T. reesei ace1 gene consisted primarily of an expression cassette for an intron-harboring hairpin RNA (ihpRNA) (Fig. 1b). The pdc promoter (Ppdc), the pdc terminator (Tpdc), two 507-bp ace1 fragments (ace1 sense and ace1 anti) covering the ace1 open reading frame from 1,780 to 2,286 bp, and a 249-bp spacer fragment (IT), which included intron 2 of the T. reesei eg2 gene were PCR-amplified using T. reesei QM9414 genomic DNA as a template. Primers used, and their targets, were as follows: Ppa-F and Ppa-R (for amplification of Ppdc, adding a KpnI site to the 5′ end), Tpa-F and Tpa-R (for Tpdc amplification, adding a HindIII site to the 3′ end), aces-F and aces-R (for ace1 sense amplification), acea-F and acea-R (for ace1 anti amplification, adding an XbaI site to the 5′ end), and it-F and it-R (for IT amplification, adding an XbaI site to the 3′ end). The amplified Ppdc, ace1 sense, and IT fragments were fused by overlap extension PCR using primers Pp-F and it-R, and the resulting fusion fragment was inserted into pUC19 using the above-mentioned restriction sites, generating the recombinant plasmid pPpdc-aces-IT. Amplified ace1 anti and Tpdc fragments were also fused by overlap extension PCR using primers acea-F and Tp-R. The fusion fragment was inserted into plasmid pPpdc-aces-IT, generating the RNAi plasmid pPDC-ACEi.
Fungal transformations
Protoplast transformation of T. reesei RUT C30 was performed as previously described [17] with the following modifications. Lysing enzymes (10 mg ml−1) from T. harzianum (Sigma-Aldrich, Brondby, Denmark, Cat. No. L1412) in 1 mol l−1 MgSO4 were used for protoplast formation. For overexpression of xyr1, T. reesei RUT C30 was co-transformed with equal amounts of plasmids (5 μg each) pPDC-XYR and pAN7.1. To combine xyr1 overexpression and ace1 downregulation, T. reesei RUT C30 was co-transformed with equal amounts of plasmids (5 μg each) pPDC-XYR, pPDC-ACEi, and pAN7.1. The plasmid pAN7.1 was used as an assisting plasmid to confer hygromycin B resistance on the transformants. The transformants were selected on PDA plates containing 100 μg ml−1 hygromycin B. The conidia of the candidate transformants were further spread onto hygromycin B selection plates to form single colonies. The single colonies were screened by genomic DNA PCR analysis to verify that xyr1 and/or ihpRNA expression cassettes were integrated into the genome of the transformants.
Southern-blot analysis of transformants
Fungal genomic DNA was isolated with a fungal DNA extraction kit (Sangon, Shanghai, China). Southern hybridization and detection was carried out with the PCR DIG Probe Synthesis Kit (Roche Diagnostics, Basel, Switzerland). To determine the copy number of the xyr1 expression cassettes, the genomic DNA (5 μg) of PX41, PXAi20 was digested with EcoRI. The 581-bp probe for the xyr1 gene was amplified with the primers S-XYR-F (TCCAGGATGGTCCAGAGG) and S-XYR-R (ATCGCACGCCAAAGACA), using plasmid pPDC-XYR as template.
Nucleic acid isolation
Mycelia in a liquid culture were harvested by centrifugation, frozen in liquid nitrogen, and stored at −86 °C. Genomic DNA was extracted as previously described [23]. Total RNA was isolated from frozen mycelia using Trizol regent (Invitrogen, Carlsbad, CA, USA) according to the manufacturer’s instructions. To remove genomic DNA, RNA extracts were treated with DNase I (Fermentas, Vilnius, Lithuania). The quantity and quality of extracted RNA was assessed on a GeneQuant 1300 spectrophotometer (Biochrom, Cambridge, UK) and by agarose gel electrophoresis.
Quantitative reverse-transcription PCR
Approximately 500 ng of total RNA was subjected to reverse transcription using an RT-PCR kit (Takara, Dalian, China), which contained a blend of oligo(dT) and random hexamer primers. Quantitative PCR (qPCR) was performed in an ABI Prim 7300 System (Applied Biosystems, Carlsbad, CA, USA). Each 20-μl reaction volume contained 2 μl of template (1:60 dilution of the reverse transcriptase (RT) reaction product), 10 μl of 2 × SYBR Premix Ex Taq (Takara), 300 nmol l−1 forward and reverse primers (Table 2), and nuclease-free water. The PCR protocol consisted of 30 s of initial denaturation at 95 °C, followed by 40 cycles of 5 s at 95 °C and 31 s at 60 °C. A melting curve analysis was performed after each run to check PCR product specificity. Primers were designed so that the amplicon length was 100–200 bp and complementary sequences within primers and mismatches were avoided. In addition, half of the primer hybridized to the 3′ end of one exon and the other half to the 5′ end of the adjacent exon, or, alternatively, the primer flanked a region containing at least one intron.
qPCR primers used in this study
Primers . | Sequences (5′–3′) . |
---|---|
sar1-F | TGGATCGTCAACTGGTTCTACGA |
sar1-R | GCATGTGTAGCAACGTGGTCTTT |
ace1-F | GGACGAGGAGGAGATTATG |
ace1-R | GTGAGTCTTCTCGTGCTT |
xyr1-F | CCATCAACCTTCTAGACGAC |
xyr1-R | AACCCTGCAGGAGATAGAC |
cbh1-F | CCGAGCTTGGTAGTTACTCTG |
cbh1-R | GGTAGCCTTCTTGAACTGAGT |
egl1-F | CGGCTACAAAAGCTACTACG |
egl1-R | CTGGTACTTGCGGGTGAT |
bgl1-F | AGTGACAGCTTCAGCGAG |
bgl1-R | GGAGAGGCGTGAGTAGTTG |
xyn2-F | CCGTCAACTGGTCCAACT |
xyn2-R | ACACGGAGAGGTAGCTGTT |
Primers . | Sequences (5′–3′) . |
---|---|
sar1-F | TGGATCGTCAACTGGTTCTACGA |
sar1-R | GCATGTGTAGCAACGTGGTCTTT |
ace1-F | GGACGAGGAGGAGATTATG |
ace1-R | GTGAGTCTTCTCGTGCTT |
xyr1-F | CCATCAACCTTCTAGACGAC |
xyr1-R | AACCCTGCAGGAGATAGAC |
cbh1-F | CCGAGCTTGGTAGTTACTCTG |
cbh1-R | GGTAGCCTTCTTGAACTGAGT |
egl1-F | CGGCTACAAAAGCTACTACG |
egl1-R | CTGGTACTTGCGGGTGAT |
bgl1-F | AGTGACAGCTTCAGCGAG |
bgl1-R | GGAGAGGCGTGAGTAGTTG |
xyn2-F | CCGTCAACTGGTCCAACT |
xyn2-R | ACACGGAGAGGTAGCTGTT |
qPCR primers used in this study
Primers . | Sequences (5′–3′) . |
---|---|
sar1-F | TGGATCGTCAACTGGTTCTACGA |
sar1-R | GCATGTGTAGCAACGTGGTCTTT |
ace1-F | GGACGAGGAGGAGATTATG |
ace1-R | GTGAGTCTTCTCGTGCTT |
xyr1-F | CCATCAACCTTCTAGACGAC |
xyr1-R | AACCCTGCAGGAGATAGAC |
cbh1-F | CCGAGCTTGGTAGTTACTCTG |
cbh1-R | GGTAGCCTTCTTGAACTGAGT |
egl1-F | CGGCTACAAAAGCTACTACG |
egl1-R | CTGGTACTTGCGGGTGAT |
bgl1-F | AGTGACAGCTTCAGCGAG |
bgl1-R | GGAGAGGCGTGAGTAGTTG |
xyn2-F | CCGTCAACTGGTCCAACT |
xyn2-R | ACACGGAGAGGTAGCTGTT |
Primers . | Sequences (5′–3′) . |
---|---|
sar1-F | TGGATCGTCAACTGGTTCTACGA |
sar1-R | GCATGTGTAGCAACGTGGTCTTT |
ace1-F | GGACGAGGAGGAGATTATG |
ace1-R | GTGAGTCTTCTCGTGCTT |
xyr1-F | CCATCAACCTTCTAGACGAC |
xyr1-R | AACCCTGCAGGAGATAGAC |
cbh1-F | CCGAGCTTGGTAGTTACTCTG |
cbh1-R | GGTAGCCTTCTTGAACTGAGT |
egl1-F | CGGCTACAAAAGCTACTACG |
egl1-R | CTGGTACTTGCGGGTGAT |
bgl1-F | AGTGACAGCTTCAGCGAG |
bgl1-R | GGAGAGGCGTGAGTAGTTG |
xyn2-F | CCGTCAACTGGTCCAACT |
xyn2-R | ACACGGAGAGGTAGCTGTT |

Southern-blot analysis of the transformants PX41, PXAi20. The genomic DNA (5 μg) was digested with EcoRI and hybridized with the amplified 581-bp probe for the xyr1 gene. PX41: recombinant strain constitutively expressing xyr1 under the control of the pdc promoter; PXAi20: recombinant strain with both xyr1 over-expression and ace1 downregulation
Enzyme activity assays
Total soluble protein concentration in the culture supernatant was assayed using Bradford reagent (Sangon Biotech, Shanghai, China). Xylanase activity was measured following [3], using birch xylan (Sigma-Aldrich, Brondby, Denmark) as the substrate. Filter paper activity (FPA) and carboxymethyl cellulase assay for β-1,4-endo-glucanase activity were measured according to the method of Ghose [6]. One unit of enzyme activity was defined as the amount of enzyme releasing 1 μmol of product per minute.
Results and Discussion
Construction of a strain with xyr1 overexpression
Xyr1 is an essential regulatory protein responsible for activation of major cellulolytic and xylanolytic enzymes in T. reesei [27]. Recently, Portnoy et al. [19] observed greatly increased xyr1 basal expression, compared with strain QM9414, in the industrial hyperproducer strain CL847. Regulation of xyr1 expression is thus known to have a significant impact on the ability of T. reesei to produce cellulose- and hemicellulose-degrading enzymes. To produce the regulator Xyr1 in T. reesei under the control of the strong constitutive promoter Ppdc, the cassette Ppdc-xyr1-Tpdc was constructed by linking the coding region of the xyr1 gene to 1.3 kb of the pdc promoter and 0.7 kb of the pdc gene 3′ flanking region comprising the transcription terminator. The expression cassette was co-transformed into T. reesei RUT C30 with plasmid pAN7.1. Upon screening of 40 randomly selected transformants by PCR, 27 showed successful integration of the xyr1 expression cassette. According to qPCR analysis, the xyr1 expression levels in five randomly selected transformants carrying the xyr1 expression cassette were much higher than those in RUT C30 (data not shown). One of them, the transformant PX41 with the highest xyr1 expression levels, was selected for the following studies. Southern-blot analysis showed that PX41 contained a single-copy insert of the xyr1 expression cassette (Fig. 2).
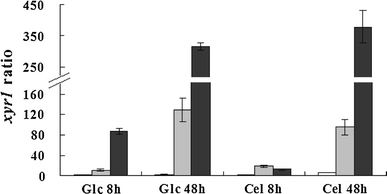
Expression ratio of xyr1 to steady-state sar1 reference gene in strains RUT C30 (open bars), PX41 (shaded bars), and PXAi20 (filled bars) pre-grown with glycerol as a carbon source and then transferred into glucose (Glc) or cellulose (Cel) medium for 8 or 48 h. RUT C30: parental strain; PX41: recombinant strain constitutively expressing xyr1 under the control of the pdc (encoding pyruvate decarboxylase) promoter; PXAi20: recombinant strain with both xyr1 over-expression and ace1 downregulation. Error bars indicate standard deviations
xyr1 overexpression leads to significant increases in enzyme production
To investigate the effects of xyr1 overexpression on cellulase expression and protein production, cellulase and xylanase activity and amounts of extracellular protein produced by the xyr1-overexpressing transformant (PX41) and the parent strain were measured from culture supernatants under inducing conditions. In addition, the effects of xyr1 overexpression on cellulase production were studied in glucose-based cultivations.
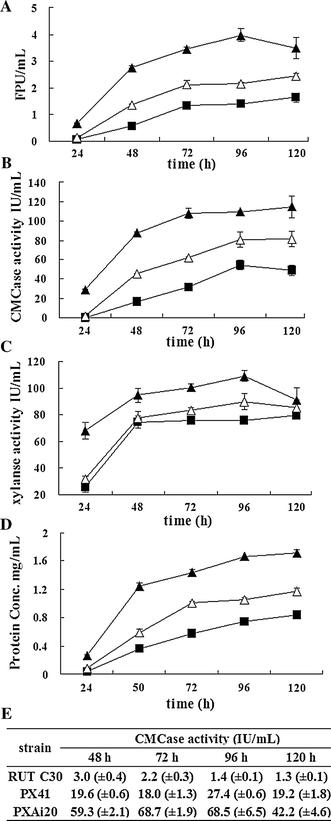
Enzyme activities and extracellular protein production at different times in strains RUT C30 (filled square), PX41 (open triangle), and PXAi20 (filled triangle) pre-grown with glycerol as a carbon source and then transferred to cellulose (a–d) or glucose (e). Filter paper (FPA), CMCase, and xylanase activities were measured using filter paper, sodium carboxymethyl cellulose (CMC-Na), and birch xylan as substrates, respectively. a FPA; b CMCase activity; c xylanase activity; d extracellular protein production; e CMCase activity in glucose-based cultivation. Averages from three parallel cultivations of each strain are shown with standard deviations
To determine whether expression of primary cellulase genes was indeed elevated in PX41 and to study the role of Xyr1 in cellulase and xylanase expression in the presence of different carbon sources, strains PX41 and RUT C30 were pre-grown on glycerol and then transferred into liquid minimal media containing a different carbohydrate—either glycerol, glucose, or cellulose—as the sole carbon source. mRNA levels of the major cellulase genes (cbh1, egl1, and bgl1) and one xylanase (xyn2) were monitored by qPCR of samples collected 8 and 48 h after transfer.
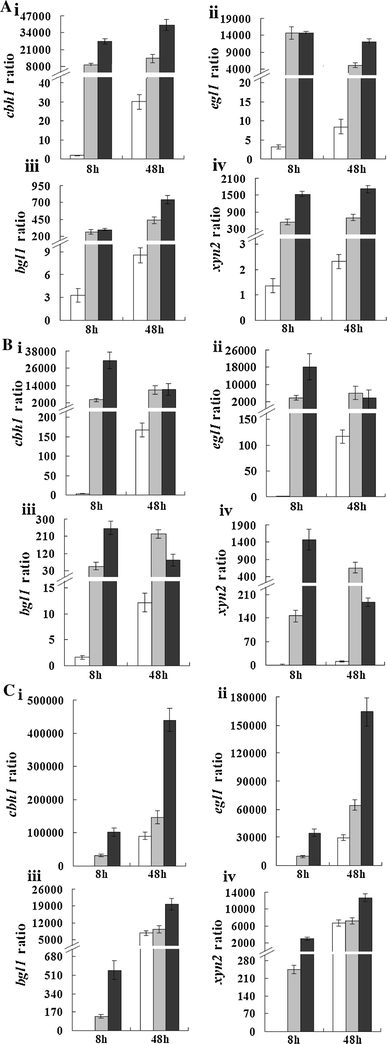
Expression ratio of (i) cbh1, (ii) egl1, (iii) bgl1, and (iv) xyn2 to the steady-state sar1 reference gene in strains RUT C30 (open bars), PX41 (shaded bars), and PXAi20 (filled bars) pre-grown with glycerol as a carbon source and then transferred to a glycerol, b glucose, or c cellulose for 8 or 48 h. RUT C30: parental strain; PX41: recombinant strain constitutively expressing xyr1 under the control of the pdc promoter; PXAi20: recombinant strain with both xyr1 over-expression and ace1 downregulation. Error bars indicate standard deviations
Consistent with observed CMCase activity, the relative transcript abundance of cellulase and xylanase genes in PX41 in glycerol and glucose media (Fig. 5a, b) was already very high at the earliest measured time point, indicating derepression and activation of cellulase and xylanase gene expression under non-inducing conditions. This implies that disruption of cre1 alone is insufficient for extensive cellulase expression, and that Xyr1 is essential for extensive activation of cellulase expression under both inducing and repressing conditions. These findings are consistent with the observation of Nakari-Setälä et al. [15] that deletion of cre1 only slightly improved protein production in the hypercellulolytic strain VTT-D-80133. In contrast to results observed with cellulose, bgl1 and xyn2 transcript levels were consistently higher in PX41 than in RUT C30 when grown on glycerol or glucose; this indicates that gene regulation was somewhat different for these two genes depending on whether inducing or non-inducing conditions were used.
Construction of a strain with both xyr1 overexpression and ace1 downregulation
Deletion of the ace1 repressor in T. reesei has been shown to increase expression of the major cellulase and hemicellulase genes in sophorose- and cellulose-induced cultures (Aro et al. [1]). In addition, RNAi-mediated gene silencing of T. koningii ace1 enhanced production of total proteins, cellulase, and xylanase under inducing conditions [29]. Gene transcription and protein production are often due to the synergistic action of different transcriptional factors. To explore whether enzyme production in RUT C30 could be further improved by simultaneous overexpression of xyr1 and silencing of ace1, we constructed a strain with these features by co-transforming an xyr1 expression cassette and an ace1 silencing vector with the aid of the plasmid pAN7.1.
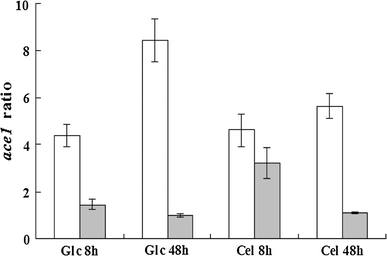
Expression ratio of ace1 to steady-state sar1 reference gene in strains RUT C30 (open bars) and PXAi20 (filled bars) pre-grown with glycerol as a carbon source and then transferred to glucose (Glc) or cellulose (Cel) for 8 or 48 h. RUT C30: parental strain; PXAi20: recombinant strain with both xyr1 over-expression and ace1 downregulation. Error bars indicate standard deviations
Further increased enzyme production by both xyr1 overexpression and ace1 downregulation
To study the combined effects of xyr1 overexpression and ace1 downregulation on enzyme production, PXAi20 was cultured in glucose and cellulose media as described previously for PX41 and RUT C30. As early as 24 h after transfer into cellulose media, cellulase and xylanase activities were clearly induced to higher levels in PXAi20 compared with PX41 and RUT C30 (Fig. 4). After 5 day of cultivation, FPA, CMCase activity, and secreted protein levels were 43, 41, and 45 % higher, respectively, in PXAi20 compared with PX41. Interestingly, downregulation of ace1 led to clearly higher levels of xylanase activity in PXAi20 compared with PX41 under cellulose cultivation (Fig. 4c).
Surprisingly, further increases in CMCase activity (about 1.2- to 2.8-fold higher) were detected in PXAi20 compared with PX41 under glucose-based cultivation (Fig. 4e). The overall results show that a combination of xyr1 overexpression and ace1 downregulation was more effective in boosting enzyme production in T. reesei RUT C30 than was xyr1 overexpression alone.
We also studied the effect of combined xyr1 overexpression and ace1 downregulation on cellulase and xylanase gene expression in PXAi20. PXAi20 was grown in glycerol, glucose, and cellulose cultures in the same manner as for PX41 and RUT C30. In media containing cellulose as the sole carbon source, cellulase and xylanase gene expression was significantly higher in PXAi20 than in PX41 (Fig. 5c). In contrast to PX41, xyn2 expression levels were clearly higher in PXAi20 than in RUT C30 under cellulose cultivation; this result implies that Ace1 negatively regulates, either directly or indirectly, xyn2 expression in RUT C30, and is consistent with previous findings that deletion of the ace1 gene leads to increased xyn2 expression in T. reesei QM9414 [1]. The above results accord well with the enzyme activity data. Similarly, at the early time point in glycerol and glucose media, cellulase and xylanase genes were expressed at a higher level in PXAi20 compared with PX41 (Fig. 5a, b); 48 h after transfer into glucose media, cbh1 and egl1 expression levels in PXAi20 resembled those in PX41, while bgl1 and xyn2 expression levels were lower in PXAi20 than in PX41.
These findings suggest that Xyr1 expression alone is sufficient for extensive cellulase induction in RUT C30 and indicate that increased xyr1 expression increases cellulase gene expression and consequently cellulase activity.
Conclusions
In this study, we improved cellulase production in hyperproducer strain RUT C30 by altering cellulase regulator expression. The positive regulator Xyr1 was constitutively expressed under the strong T. reesei pdc promoter in RUT C30, resulting in significant increases in cellulase activity and levels of corresponding mRNAs. Constitutive expression of xyr1 combined with downregulation of the repressor-encoding gene ace1 further increased cellulase and xylanase activities in RUT C30. These findings form the basis for an attractive new single-step approach for increasing total cellulase activity in T. reesei.
Acknowledgments
This research was supported in part by the National Natural Science Foundation of China (No. 31070044), the Shenzhen Municipal Science and Technology Basic Research Program (JC201005280559A), and Shenzhen Municipal Science and Technology key projects of Basic Research Program (JC201005250041A, JCYJ20120613115323982).
Conflict of interests
The authors declare that they have no competing interests.