-
PDF
- Split View
-
Views
-
Cite
Cite
Haitao Zhang, Young Hwan Moon, Brian J Watson, Maxim Suvorov, Elizabeth Santos, Corinn A Sinnott, Steven W Hutcheson, Hydrolytic and phosphorolytic metabolism of cellobiose by the marine aerobic bacterium Saccharophagus degradans 2-40T, Journal of Industrial Microbiology and Biotechnology, Volume 38, Issue 8, 1 August 2011, Pages 1117–1125, https://doi.org/10.1007/s10295-011-0945-4
- Share Icon Share
Abstract
Saccharophagus degradans 2-40 is a marine gamma proteobacterium that can produce polyhydroxyalkanoates from lignocellulosic biomass using a complex cellulolytic system. This bacterium has been annotated to express three surface-associated β-glucosidases (Bgl3C, Ced3A, and Ced3B), two cytoplasmic β-glucosidases (Bgl1A and Bgl1B), and unusual for an aerobic bacterium, two cytoplasmic cellobiose/cellodextrin phosphorylases (Cep94A and Cep94B). Expression of the genes for each of the above enzymes was induced when cells were transferred into a medium containing Avicel as the major carbon source except for Bgl1B. Both hydrolytic and phosphorolytic degradation of cellobiose by crude cell lysates obtained from cellulose-grown cells were demonstrated and all of these activities were cell-associated. With the exception of Cep94B, each purified enzyme exhibited their annotated activity upon cloning and expression in E. coli. The five β-glucosidases hydrolyzed a variety of glucose derivatives containing β-1, (2, 4, or 6) linkages but did not act on any α-linked glucose derivatives. All but one β-glucosidases exhibited transglycosylation activity consistent with the formation of an enzyme-substrate intermediate. The biochemistry and expression of these cellobiases indicate that external hydrolysis by surface-associated β-glucosidases coupled with internal hydrolysis and phosphorolysis are all involved in the metabolism of cellobiose by this bacterium.
Haitao Zhang and Young Hwan Moon are joint first authors.
Electronic supplementary material
The online version of this article (doi:10.1007/s10295-011-0945-4) contains supplementary material, which is available to authorized users.
Introduction
Enzymatic degradation of cellulose (β-1,4-glucan) is of interest due to applications for the fermentative conversion of its constituent glucose to biofuels and specialty chemicals. As the main polymeric constituent of plant cell walls, cellulose is the most abundantly produced polysaccharide in most ecosystems yet little accumulates due to efficient mechanisms for mineralization of this durable polymer [4, 17]. Mineralization is thought to involve the synergistic action of endoglucanases and cellobiohydrolases secreted by cellulolytic microorganisms and subsequent hydrolysis of the released cellobiose by β-glucosidases [11, 21, 36].
Saccharophagus degradans 2-40 is a marine gamma proteobacterium capable of producing polyhydroxyalkanoates (PHA) from biomass directly [2, 9, 34]. This obligate aerobe expresses an atypical cellulolytic system [7, 34, 38] consisting of ten GH5 endoglucanases, one GH6 endoglucanase, and two GH9 endoglucanases, but lacks an obvious cellobiohydrolase typical of most other cellulolytic systems [35, 37, 38]. Instead, three of the endoglucanases (Cel5G, Cel5H, and Cel5J) appear to be processive, releasing cellobiose from several types of cellulose [37]. Sequence similarity to the above or analogy to other cellulolytic bacteria, e.g., [39], suggests that three other cellulases could also be processive (Cel5A, Cel9A, and Cel9B) [34]. Cellobiose, and to a lesser extent cellotriose, are the major products of the endoglucanases secreted by this bacterium [37]. Production of cellulases by this bacterium during growth on Avicel is supported by metabolic profiling and direct activity measurements [32].
The annotation of the S. degradans genome revealed seven enzymes that could be involved in the metabolism of cellobiose and cellotriose [35, 38] to PHA. Bgl1A and Bgl1B carry a strong homolog of GH1 but lack obvious signal sequences, suggesting they are cytoplasmic β-glucosidases. Similarly, Cep94A and Cep94B are predicted cytoplasmic cellobiose/cellodextrin phosphorylases. The predicted cellodextrinases Ced3A, Ced3B and β-glucosidase Bgl3C all retain signal sequences typical of complete type II protein secretion but also carry lipoboxes indicative of acylation. This suggests they may be attached to the outer membrane similarly to pullulanase [27, 35]. Thus, all annotated β-glucosidases appear to be cell-associated either as surface-associated external enzymes or are cytoplasmic enzymes.
As a first step toward understanding cellobiose metabolism by S. degradans, both hydrolytic and phosphorolytic degradation of cellodextrins were demonstrated. Expression, activity, and cellular locations of the predicted β-glucosidases were confirmed. Unusual for an obligate aerobe, phosphorolysis of cellobiose linked to Cep94A was demonstrated. Hydrolytic degradation was found to be three times greater than the phosphorolytic activity. The results are consistent with the involvement of all three pathways in the metabolism of cellulose.
Materials and methods
Microorganism and growth conditions
Saccharophagus degradans 2-40T (ATCC 43961) was grown at 28°C in defined medium containing 2.3% (w/v) Instant Ocean (Aquarium Systems, Mentor, OH), 0.05% yeast extract, 0.5% ammonium chloride, and 16.7 mM Tris pH 8.6 supplemented by 0.2% carbon source (2-40 medium; [8]). Escherichia coli DH5α and Rosetta2™ (DE3) (Novagen, Madison, WI) were grown at 37°C in Luria–Bertani (LB) broth or agar supplemented with chloramphenicol (34 μg/ml) and/or kanamycin (50 μg/ml). Unless indicated otherwise, chemicals were obtained from Sigma-Aldrich (St. Louis, MO).
Molecular cloning of S. degradans enzymes
Genomic DNA of S. degradans was prepared by using a genomic DNA purification kit (Promega, Madison, WI). Gene models and estimated molecular weights are derived from Taylor et al. [35]. Primers were designed to amplify the ORF of interest for in-frame insertion into the vector pET28b(+) (Novagen) (Supplementary Table 1). For those gene products predicted to be secreted, the coding sequence for the secretion signal as determined by the SMART algorithm was not included in the amplified product. Gene fragments were amplified by PCR using Pfu DNA polymerase (Agilent, Santa Clara, CA) and digested with the restriction enzymes whose unique cleavage sites were incorporated into the primer pair (Supplemental Table 1). Buffer transfers employed DNA purification kits from Qiagen (Valencia, CA) and Bio-Rad (Hercules, CA). Digested fragments were ligated into like-digested pET28b(+) (Novagen) and transformed into E. coli DH5α. Kanr transformants were screened by colony PCR for presence of the expected insert. Plasmid from insert-positive transformants were transformed into E. coli Rosetta2™ (DE3) for T7-linked expression of the cloned fragment by electroporation and transformants selected using Cm and Kan. Insertions were confirmed by sequence analysis.
Expression of cloned genes and purification of gene products
Rosetta2™(DE3) transformants were grown at 37°C to an OD600 of 0.6 and induced at 0.4 mM IPTG (isopropyl-β-D-thiogalactopyranoside). Cultures were maintained at 16°C with shaking for 16 h and cells harvested. Cells were lysed in 50 mM NaH2PO4, 300 mM NaCl, 10 mM imidazole pH 8.0 using a Mini bead beater (Biospec, Bartlesville, OK) and lysates clarified by centrifugation. The expressed enzymes were purified by Ni–NTA chromatography as suggested by the manufacturer (Qiagen, Valencia, CA). Active fractions were pooled and dialyzed against 50 mM HEPES buffer (pH 7.0). The protein concentration was measured using the Pierce Micro BCA™ protein assay kit (Pierce, Rockford, IL) with bovine serum albumin as the standard. Purity of protein preparations and the molecular weight of the primary product was evaluated by SDS–polyacrylamide gel electrophoresis.
Enzyme assays
For determination of the enzyme activity in crude lysates, S. degradans was grown for 6 h in minimal medium containing 0.2% glucose or Avicel as the primary carbon source. After 6-h culture, the cells were harvested and lysed in 50 mM buffer pH 8.0 supplemented with 300 mM NaCl and 10 mM imidazole using a Mini bead beater (Biospec, Bartlesville, OK). For assay of β-glucosidases, sodium phosphate buffer was used whereas for assay of phosphorylases HEPES–KOH buffer was used. All assays were done in triplicate and averages reported.
Hydrolysis of pNP-β-glucoside was determined by monitoring the formation of p-nitrophenol spectrophotometrically. The reaction mixture (500 μl) contained 20 mM PIPES, pH 6.5, and 0.15 mM pNP-β-glucoside initiated by the addition of β-glucosidase. After incubation at 37°C for the indicated time, the reaction was quenched by the addition of 500 μl of 1 M Tris base. The absorbance at 400 nm was used to calculate product formation. To determine pH optima, 20 mM citric acid (pH 5.0–6.0) and 20 mM PIPES buffer (pH 6.5–9.0) were used.
Cellobiose hydrolysis was monitored by the release of glucose as detected using a glucose oxidase-based kit (Sigma-Aldrich). The reaction mixture (200 μl) contained 20 mM PIPES pH 6.5 and 0.15 mM cellobiose unless indicated otherwise. Reactions were initiated by the addition of the β-glucosidase preparation. Cellobiose hydrolysis was calculated as 1/2 the rate of glucose accumulation. Cellotetraose hydrolysis employed 1% cellotetraose. Products were determined by thin-layer chromatography (TLC). Transglycosylation activity was tested by incubating the indicated enzymes with 10 or 20% cellobiose and 20 and 40% glucose for 12 h at 37°C as described by Saloheimo [30]. The reaction mixtures were then heated to 95°C for 10 min and reaction products determined by TLC [37].
Phosphorolytic activity was determined by measuring the amount of glucose 1- phosphate formed from the indicated substrate using a coupled enzyme assay [13]. The complete reaction mixture (1 ml) contained 50 mM PIPES, 8 mM KH2PO4 pH 7.0, 5 mM MgSO4, 0.5 mM NADP, 4 U of phosphoglucomutase, 2 U of glucose-6-phosphate dehydrogenase, 10 mM of cellobiose, and the phosphorylase preparation. The assay mixture was incubated at 42°C for 10 min and heated to 95°C for 5 min to stop the reaction. NADPH formation was calculated from the change in absorbance at 340 nm and glucose 1-phosphate formation estimated assuming molar equivalence to the NADPH formed. For estimation of optimum conditions, 2.5 mM cellobiose and 8 mM KH2PO4 were used in the reaction mixture. To find the optimum pH, the following buffers (50 mM) were used: 2-(N-morpholino)ethanesulfonic acid (MES) (pH 5.0–6.5), piperazine-N,N′-bis(2-ethanesulfonic acid) (PIPES) (pH 6.5–7.5), Tris[hydroxymethyl]aminomethane hydrochloride (Tris/HCl) (pH 7.5–8.5), and N-[2-hydroxyethyl]piperzine-N′-[2-ethanesulfonic acid] (HEPES) (pH 6.5–8.5). The inhibitory effects of glucose and glucose 1-phosphate were investigated by measuring glucose formation by the purified enzyme in the presence of glucose 1-phosphate or glucose 1-phosphate formation in the presence of glucose as described above. Ki values were calculated using the slope of Lineweaver–Burk plots of the activity.
qRT-PCR analysis of gene expression
Total RNA was extracted from S. degradans cells culture at the indicated times by using the RNeasy kit and RNAprotect™ reagent as recommended by the manufacturer (Qiagen). cDNA was synthesized using the QiantiTect™ Reverse Transcription Kit. For qRT-PCR, primer pairs (Supplemental Table 2) were designed using online software (http://frodo.wi.mit.edu/cgi-bin/primer3/primer3_www.cgi) to amplify 120–180 bp regions internal to the gene of interest. The housekeeping genes guanylate kinase (GK) and dihydrofolate reductase (DR) were used as controls. The qRT-PCR reaction (20 μl) contains 10 μl of 2 × LightCycler® 480 SYBR Green IMaster, 1 μl of cDNA, 1 μl of each 5 μM forward and reverse primers and 7 μl of dH2O. Real-time PCR was performed in a Light Cycler™ 480 (Roche Diagnostics, Penzberg, Germany). Cycling conditions were: 95°C for 4 min and then 45 cycles of 95°C for 15 s, 56°C for 15 s and 72°C for 20 s. Data was interpreted using Roche Diagnostics software. Basal expression represents transcript levels relative to that of guanylate kinase during exponential growth in broth medium containing glucose as the major carbon source. Induction is reported as the fold increase in transcript level after 4 h growth with Avicel as the major carbon source relative to growth on glucose. Data reported is the mean of three independent trials.
Results
Cellobiose degradation by crude lysates of S. degradans 2-40

Hydrolytic and phosphorolytic activity of clarified S. degradans lysates during growth on cellulose. The specific activity of phosphorolysis and hydrolysis in matched aliquots of the same lysate were determined separately. The results are the mean of three trials with standard deviation shown
These activities appeared to be tightly regulated. Cellobiose phosphorylase activity of cell lysates was essentially abolished by growth in media with glucose as the major carbon source and hydrolytic cellobiase activity was substantially reduced (Fig. 1). During exponential growth, the β-glucosidase activities of lysates obtained from glucose-grown cells was 15% that obtained from cells cultured with cellulose as the major carbon source. Phosphorolytic activity was 2.5% that of cellulose-grown cells.
Induction and expression of cellobiases
The expression of the genes thought to encode homologs of potential β-glucosidases and cellobiose phosphorylases was examined by qRT-PCR. During exponential growth in media containing Avicel as the major carbon source, the candidate β-glucosidases bgl1A, ced3A, and bgl3C were strongly induced (>50-fold) relative to their expression during growth on glucose (Table 1). Expression of both cep94A and cep94B were also strongly induced upon transfer to media containing cellulose. Modest expression of the genes of interest was observed during growth on glucose with the basal expression level of bgl1A much higher than that of the other β-glucosidase or phosphorylase genes. Thus the surface-associated Bgl3C as well as the cytoplasmic Bgl1A are the most likely candidates to contribute to the observed hydrolytic activity as they were the highest expressed during growth on Avicel whereas the relative role of the phosphorylases could not be resolved.
Expression of β-glucosidases and induction by Avicel
Gene . | Basal expressiona . | Inductionb . |
---|---|---|
bgl1A | 1,509 ± 541 | 53 ± 7 |
bgl1B | 159 ± 62 | 1 ± 1 |
ced3A | 25 ± 12 | 67 ± 34 |
ced3B | 430 ± 281 | 3 ± 1 |
bgl3C | 404 ± 264 | 108 ± 14 |
cep94A | 103 ± 22 | 1,240 ± 63 |
cep94B | 410 ± 101 | 167 ± 53 |
Gene . | Basal expressiona . | Inductionb . |
---|---|---|
bgl1A | 1,509 ± 541 | 53 ± 7 |
bgl1B | 159 ± 62 | 1 ± 1 |
ced3A | 25 ± 12 | 67 ± 34 |
ced3B | 430 ± 281 | 3 ± 1 |
bgl3C | 404 ± 264 | 108 ± 14 |
cep94A | 103 ± 22 | 1,240 ± 63 |
cep94B | 410 ± 101 | 167 ± 53 |
aThe average percentage transcript level with the standard deviation relative to the transcript level for guanylate kinase in three trials
bAverage fold increase in transcript level with standard deviation after 4 h growth in media containing Avicel as the major carbon source in three trials
Expression of β-glucosidases and induction by Avicel
Gene . | Basal expressiona . | Inductionb . |
---|---|---|
bgl1A | 1,509 ± 541 | 53 ± 7 |
bgl1B | 159 ± 62 | 1 ± 1 |
ced3A | 25 ± 12 | 67 ± 34 |
ced3B | 430 ± 281 | 3 ± 1 |
bgl3C | 404 ± 264 | 108 ± 14 |
cep94A | 103 ± 22 | 1,240 ± 63 |
cep94B | 410 ± 101 | 167 ± 53 |
Gene . | Basal expressiona . | Inductionb . |
---|---|---|
bgl1A | 1,509 ± 541 | 53 ± 7 |
bgl1B | 159 ± 62 | 1 ± 1 |
ced3A | 25 ± 12 | 67 ± 34 |
ced3B | 430 ± 281 | 3 ± 1 |
bgl3C | 404 ± 264 | 108 ± 14 |
cep94A | 103 ± 22 | 1,240 ± 63 |
cep94B | 410 ± 101 | 167 ± 53 |
aThe average percentage transcript level with the standard deviation relative to the transcript level for guanylate kinase in three trials
bAverage fold increase in transcript level with standard deviation after 4 h growth in media containing Avicel as the major carbon source in three trials
Cloning and expression of annotated β-glucosidases and cellobiose phosphorylases
The annotation of the genome provides hypotheses as to biochemical functions of gene products. To evaluate their annotated biochemical activity, the genes encoding likely β-glucosidases and cellobiose phosphorylases were amplified by PCR and ligated into pET28b to create His-tagged derivatives. The resulting constructs were transformed into the cellobiase- and cellobiose phosphorylase-deficient E. coli Rosetta2™ (DE3). In each case, induced E. coli transformants acquired the ability to either hydrolytically convert cellobiose to glucose when the expressed gene was predicted to encode a β-glucosidase or phosphorolytically converted cellobiose to glucose 1-phosphate and glucose when cep94A was expressed (data not shown). Attempts to detect cellodextrin phosphorylase activity in transformants expressing cep94B were unsuccessful due to the apparent poor stability of the Cep94B protein in the E. coli strains tested.
Properties of purified β-glucosidases
Mid log cultures of each of the Rosseta2™ (DE3) transformants carrying a cloned β-glucosidase gene were induced using IPTG and the expressed His-tagged protein was purified to near homogeneity by using Ni–NTA affinity chromatography. The MW of purified enzyme was similar to that predicted from the gene annotation (Table 2). The purified Bgl1A, Bgl1B, Bgl3C, Ced3A, and Ced3B all exhibited cellobiase activity as indicated by the release of glucose from cellobiose. The Km of Bgl1A and Bgl3C was near 1 mM whereas the Km of Bgl1B, Ced3A, and Ced3B was less than 0.5 mM (Table 3). All enzymes had a pH optimum between 6.5 and 7 with significant activity retained at pH 5.0 and pH 8.0. The enzymes were active up to 50°C. The highest activities were detected in 2.5% Instant Ocean (IO) for Bgl1A and Bgl1B and 5% IO for Bgl3C, Ced3A and Ced3B but these enzymes retained greater than 95% of their activity in the absence of IO. Cu2+ and Zn2+ (0.1 mM) inhibited the activities of all five enzymes whereas Ca2+, Fe2+, Mg2+ and Mn2+ were slightly stimulatory at this concentration.
Properties of β-glucosidases and cellobiose phosphorylases
Enzyme . | Predicted activitya . | Predicted MW (kD) . | Actual MW (kD)b . | pH opt . | Activity at T (°C) . | ||
---|---|---|---|---|---|---|---|
25 . | 37 . | 50 . | |||||
Bgl1A | BG | 52.8 | 52 | 6.5 | + | − | − |
Bgl1B | BG | 49.8 | 49 | 6.5 | + | + | − |
Ced3A | BG | 116 | 110 | 7.0 | + | + | + |
Ced3B | BG | 92.9 | 90 | 7.0 | + | + | + |
Bgl3C | BG | 95.4 | 92 | 7.0 | + | − | − |
Cep94A | CP | 91.7 | 96 | 7.0 | + | + | + |
Enzyme . | Predicted activitya . | Predicted MW (kD) . | Actual MW (kD)b . | pH opt . | Activity at T (°C) . | ||
---|---|---|---|---|---|---|---|
25 . | 37 . | 50 . | |||||
Bgl1A | BG | 52.8 | 52 | 6.5 | + | − | − |
Bgl1B | BG | 49.8 | 49 | 6.5 | + | + | − |
Ced3A | BG | 116 | 110 | 7.0 | + | + | + |
Ced3B | BG | 92.9 | 90 | 7.0 | + | + | + |
Bgl3C | BG | 95.4 | 92 | 7.0 | + | − | − |
Cep94A | CP | 91.7 | 96 | 7.0 | + | + | + |
a BG β-glucosidase/cellodextrinase, CP cellobiose phosphorylase
bAs determined by SDS–PAGE
Properties of β-glucosidases and cellobiose phosphorylases
Enzyme . | Predicted activitya . | Predicted MW (kD) . | Actual MW (kD)b . | pH opt . | Activity at T (°C) . | ||
---|---|---|---|---|---|---|---|
25 . | 37 . | 50 . | |||||
Bgl1A | BG | 52.8 | 52 | 6.5 | + | − | − |
Bgl1B | BG | 49.8 | 49 | 6.5 | + | + | − |
Ced3A | BG | 116 | 110 | 7.0 | + | + | + |
Ced3B | BG | 92.9 | 90 | 7.0 | + | + | + |
Bgl3C | BG | 95.4 | 92 | 7.0 | + | − | − |
Cep94A | CP | 91.7 | 96 | 7.0 | + | + | + |
Enzyme . | Predicted activitya . | Predicted MW (kD) . | Actual MW (kD)b . | pH opt . | Activity at T (°C) . | ||
---|---|---|---|---|---|---|---|
25 . | 37 . | 50 . | |||||
Bgl1A | BG | 52.8 | 52 | 6.5 | + | − | − |
Bgl1B | BG | 49.8 | 49 | 6.5 | + | + | − |
Ced3A | BG | 116 | 110 | 7.0 | + | + | + |
Ced3B | BG | 92.9 | 90 | 7.0 | + | + | + |
Bgl3C | BG | 95.4 | 92 | 7.0 | + | − | − |
Cep94A | CP | 91.7 | 96 | 7.0 | + | + | + |
a BG β-glucosidase/cellodextrinase, CP cellobiose phosphorylase
bAs determined by SDS–PAGE
Kinetic parameters of β-glucosidases
. | Bgl1A . | Bgl1B . | Bgl3C . | Ced3A . | Ced3B . | |
---|---|---|---|---|---|---|
Cellobiose | K m (mM) | 1.05 | 0.42 | 1.01 | 0.45 | 0.34 |
V max (nmol min−1 ug−1) | 3.13 | 0.06 | 0.39 | 0.17 | 0.11 | |
PNP-glucoside | K m | 1.58 | 1.01 | 1.89 | 0.81 | 0.20 |
V max | 2.54 | 14.0 | 18.4 | 6.05 | 3.60 |
. | Bgl1A . | Bgl1B . | Bgl3C . | Ced3A . | Ced3B . | |
---|---|---|---|---|---|---|
Cellobiose | K m (mM) | 1.05 | 0.42 | 1.01 | 0.45 | 0.34 |
V max (nmol min−1 ug−1) | 3.13 | 0.06 | 0.39 | 0.17 | 0.11 | |
PNP-glucoside | K m | 1.58 | 1.01 | 1.89 | 0.81 | 0.20 |
V max | 2.54 | 14.0 | 18.4 | 6.05 | 3.60 |
Kinetic parameters of β-glucosidases
. | Bgl1A . | Bgl1B . | Bgl3C . | Ced3A . | Ced3B . | |
---|---|---|---|---|---|---|
Cellobiose | K m (mM) | 1.05 | 0.42 | 1.01 | 0.45 | 0.34 |
V max (nmol min−1 ug−1) | 3.13 | 0.06 | 0.39 | 0.17 | 0.11 | |
PNP-glucoside | K m | 1.58 | 1.01 | 1.89 | 0.81 | 0.20 |
V max | 2.54 | 14.0 | 18.4 | 6.05 | 3.60 |
. | Bgl1A . | Bgl1B . | Bgl3C . | Ced3A . | Ced3B . | |
---|---|---|---|---|---|---|
Cellobiose | K m (mM) | 1.05 | 0.42 | 1.01 | 0.45 | 0.34 |
V max (nmol min−1 ug−1) | 3.13 | 0.06 | 0.39 | 0.17 | 0.11 | |
PNP-glucoside | K m | 1.58 | 1.01 | 1.89 | 0.81 | 0.20 |
V max | 2.54 | 14.0 | 18.4 | 6.05 | 3.60 |
Under optimal conditions, each of the purified β-glucosidases could hydrolyze pNP-β-glucose as well as the β-1,4 glucosidic bond of C2–C5 cellodextrins (Table 4). All five enzymes showed high activities on the positional isomers, sophorose (β-1,2 glucose), and gentiobiose (β1,6 glucose). Bgl1A and Bgl1B could also hydrolyze lactose (β-D-galactopyranosyl-(1 → 4)-d-glucose), whereas Bgl3C, Ced3A, and Ced3B had no activity on this sugar. None of the enzymes could hydrolyze glucosyl units linked to other sugars by α- linkages, such as found in sucrose or maltodextrins.
Substrate specificity of β-glucosidases
Substrate . | Linkage . | Hydrolysis by: . | ||||
---|---|---|---|---|---|---|
Bgl1A . | Bgl1B . | Bgl3C . | Ced3A . | Ced3B . | ||
Cellobiose & cellodextrins | β1,4 | +a | + | + | + | + |
pNP-glucose | β1,4 | + | + | + | + | + |
pNP-cellobioside | β1,4 | + | + | + | + | + |
Gentiobiose | β1,6 | + | + | + | + | + |
Sophorose | β1,2 | + | + | + | + | + |
Lactose | β1,4 | + | + | − | − | − |
Sucrose | α1,4 | − | − | − | − | − |
Maltose & maltotriose | α1,4 | − | − | − | − | − |
Substrate . | Linkage . | Hydrolysis by: . | ||||
---|---|---|---|---|---|---|
Bgl1A . | Bgl1B . | Bgl3C . | Ced3A . | Ced3B . | ||
Cellobiose & cellodextrins | β1,4 | +a | + | + | + | + |
pNP-glucose | β1,4 | + | + | + | + | + |
pNP-cellobioside | β1,4 | + | + | + | + | + |
Gentiobiose | β1,6 | + | + | + | + | + |
Sophorose | β1,2 | + | + | + | + | + |
Lactose | β1,4 | + | + | − | − | − |
Sucrose | α1,4 | − | − | − | − | − |
Maltose & maltotriose | α1,4 | − | − | − | − | − |
a+ release of the glucose monomer from 1% (w/v) substrate as detected by TLC
Substrate specificity of β-glucosidases
Substrate . | Linkage . | Hydrolysis by: . | ||||
---|---|---|---|---|---|---|
Bgl1A . | Bgl1B . | Bgl3C . | Ced3A . | Ced3B . | ||
Cellobiose & cellodextrins | β1,4 | +a | + | + | + | + |
pNP-glucose | β1,4 | + | + | + | + | + |
pNP-cellobioside | β1,4 | + | + | + | + | + |
Gentiobiose | β1,6 | + | + | + | + | + |
Sophorose | β1,2 | + | + | + | + | + |
Lactose | β1,4 | + | + | − | − | − |
Sucrose | α1,4 | − | − | − | − | − |
Maltose & maltotriose | α1,4 | − | − | − | − | − |
Substrate . | Linkage . | Hydrolysis by: . | ||||
---|---|---|---|---|---|---|
Bgl1A . | Bgl1B . | Bgl3C . | Ced3A . | Ced3B . | ||
Cellobiose & cellodextrins | β1,4 | +a | + | + | + | + |
pNP-glucose | β1,4 | + | + | + | + | + |
pNP-cellobioside | β1,4 | + | + | + | + | + |
Gentiobiose | β1,6 | + | + | + | + | + |
Sophorose | β1,2 | + | + | + | + | + |
Lactose | β1,4 | + | + | − | − | − |
Sucrose | α1,4 | − | − | − | − | − |
Maltose & maltotriose | α1,4 | − | − | − | − | − |
a+ release of the glucose monomer from 1% (w/v) substrate as detected by TLC
All of the β-glucosidases appeared to be exoenzymes acting from the non-reducing end of cellodextrins. In addition to the observed hydrolysis of pNP-β-glucose, these enzymes could also hydrolyze pNP-β-cellobioside to completion. Similarly, the time course for degradation of cellotetraose by low levels of these enzymes revealed sequential degradation of the substrate to glucose as little cellobiose could be detected early in the reaction (data not shown).
Bgl1B, Ced3A, Ced3B, and Bgl3C exhibited transglycosylation activity as indicated by the formation of cellotriose and cellotetraose from cellobiose (data not shown). This was most prevalent at high cellobiose concentrations (10–20% w/v). Little transglycosylase activity was associated with Bgl1A. The transglycosylation was dependent upon catalytic activity as no products were detected from 20% glucose during 12-h incubation.
Properties of Cep94A
Similar to the β-glucosidases, the induced His-Cep94A was purified from lysates of Rosetta2™ (DE3) transformants to near homogeneity by NTA chromatography. The purified enzyme exhibited cellobiose phosphorylase activity that was absolutely dependent upon phosphate (Table 5). The purified Cep94A was specific towards cellobiose, as phosphorolytic activity was not detected from other cellodextrins (DP 3-7; data not shown). The K m and Vmax for cellobiose phosphorylase were 1.75 mM for cellobiose and 1,625 μmol cellobiose cleaved/min/μmol of protein. The k cat was 27.1 s−1. The resulting catalytic efficiency (k cat/K m) of the enzyme for cellobiose was calculated to be 15.5 s−1mM−1.
Phosphorolytic activity of S. degradans
. | Relative activity . | ||
---|---|---|---|
2-40a . | Cep94Ab . | Cep94Bc . | |
Completed | 100e | 100f | –g |
Minus cellobiose | 2.9 | 0.1 | – |
Minus Pi | 6.2 | 0.2 | – |
Minus G6P dehydrogenase | 1.1 | 0.1 | – |
Minus NADP | 1.1 | 0.1 | – |
Minus phosphoglucomutase | 3.8 | 0.1 | – |
Minus the phosphorylase | 0.9 | 0.1 | – |
. | Relative activity . | ||
---|---|---|---|
2-40a . | Cep94Ab . | Cep94Bc . | |
Completed | 100e | 100f | –g |
Minus cellobiose | 2.9 | 0.1 | – |
Minus Pi | 6.2 | 0.2 | – |
Minus G6P dehydrogenase | 1.1 | 0.1 | – |
Minus NADP | 1.1 | 0.1 | – |
Minus phosphoglucomutase | 3.8 | 0.1 | – |
Minus the phosphorylase | 0.9 | 0.1 | – |
aCrude lysate of S.degradans 2-40 grown on Avicel as the major carbon source
bPurified Cep94A
cPurified Cep94B
dThe complete assay mixture for measurement of phosphorylase activity
e42.8 nmol product formed/min/mg protein equals 100% activity
f8332 nmol product formed/min/mg protein
g2.7 nmol product formed/min/mg protein
Phosphorolytic activity of S. degradans
. | Relative activity . | ||
---|---|---|---|
2-40a . | Cep94Ab . | Cep94Bc . | |
Completed | 100e | 100f | –g |
Minus cellobiose | 2.9 | 0.1 | – |
Minus Pi | 6.2 | 0.2 | – |
Minus G6P dehydrogenase | 1.1 | 0.1 | – |
Minus NADP | 1.1 | 0.1 | – |
Minus phosphoglucomutase | 3.8 | 0.1 | – |
Minus the phosphorylase | 0.9 | 0.1 | – |
. | Relative activity . | ||
---|---|---|---|
2-40a . | Cep94Ab . | Cep94Bc . | |
Completed | 100e | 100f | –g |
Minus cellobiose | 2.9 | 0.1 | – |
Minus Pi | 6.2 | 0.2 | – |
Minus G6P dehydrogenase | 1.1 | 0.1 | – |
Minus NADP | 1.1 | 0.1 | – |
Minus phosphoglucomutase | 3.8 | 0.1 | – |
Minus the phosphorylase | 0.9 | 0.1 | – |
aCrude lysate of S.degradans 2-40 grown on Avicel as the major carbon source
bPurified Cep94A
cPurified Cep94B
dThe complete assay mixture for measurement of phosphorylase activity
e42.8 nmol product formed/min/mg protein equals 100% activity
f8332 nmol product formed/min/mg protein
g2.7 nmol product formed/min/mg protein
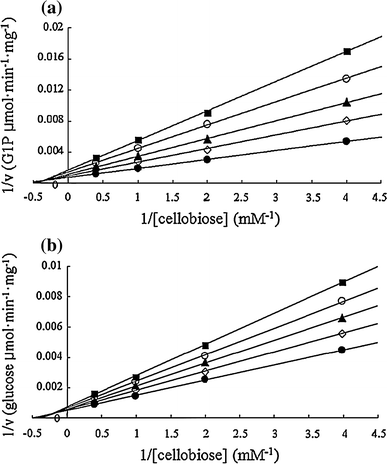
Inhibition of Cep94A activity by the reaction products. a Glucose inhibition of Cep94A activity. b Glucose 1-phosphate inhibition of Cep94A activity. Filled circle, no inhibitor added; open diamond, 0.5 mM glucose or glucose 1-phosphate; filled diamond, 1 mM glucose or glucose 1-phosphate; open circle, 1.5 mM glucose or glucose 1-phosphate; filled square, 2 mM glucose or glucose 1-phosphate
Discussion
S. degradans 2-40 is a marine bacterium able to grow on cellulose and cellobiose as major carbon sources and capable of producing industrially useful products, such as PHA [34]. Utilization of cellulose by this bacterium involves secreted GH5, GH6, and GH9 endoglucanases that release cellobiose and short cellodextrins as metabolic intermediates [35, 37]. How this bacterium metabolizes the resulting cellobiose is therefore of interest. This study confirms the predictions of Taylor et al. [35] who deduced from the genome annotation that S. degradans 2-40 uses both phosphorolytic and hydrolytic pathways for cellobiose metabolism. Five hydrolytic β-glucosidases and a phosphorolytic cellobiose phosphorylase were demonstrated and shown to function in the metabolism of cellobiose. From the relative activities, the dominant pathway was determined to be the hydrolytic pathway.
The five β-glucosidases expressed by S. degradans 2-40 exhibited properties similar to known β-glycosidases. For each of these enzymes, their Km for cellobiose was in the same range as other bacterial β-glucosidases. The MW and pH optima of these enzymes match the characteristics of most bacterial β-glucosidases, but the temperature optima of the five S. degradans β-glucosidases are among the lowest known for bacterial enzymes. Like the β-glucosidases of other bacteria [5, 20], the S. degradans β-glucosidases were sensitive to many metal ions at μM concentrations. S. degradans β-glucosidases differed from most previously characterized β-glucosidases [13, 15] in that the addition of divalent ions such as Mg2+, Ca2+, and Mn2+ stimulated activity. This is similar to the β-glucosidase of the fungus Chalara paradoxa [20].
Bacterial β-glucosidases are usually very specific to the degradation of β1–4 glucosyl linkage of cellobiose [25]. The five β-glucosidases from S. degradans, however, exhibited broad substrate specificities to hydrolyze cellobiose, gentiobiose, sophorose, cellodextrin (DP 2-7), barley β-glucan, pNP-β-glucose and pNP-β-cellobioside to their constituent glucose. Bgl1A and Bgl1B could also hydrolyze the β1,4 linkage of lactose to release galactose and glucose. Most β-glucosidases possess the ability to hydrolyze gentibiose, and some can also degrade sophorose [15]. The β-glucosidase from Gram-negative bacteria Flavobacterium johnsoniae can hydrolyze gentiobiose but is very slow on sophorose and cellobiose [24]. β-glucosidases from Streptomyces sp. QM-B814 and Thermobifida fusca were also reported to have weak lactase activities [26, 33].
Purified Bgl1B, Ced3A, Ced3B, and Bgl3C exhibited transglycosylation activity at high substrate concentrations. This transglycosylation activity mirrored the catalytic specificity of the enzymes. This phenomenon is more common with fungal and archaeal β-glucosidases [6, 14, 23]. Transglycosylation indicates the formation of an enzyme-substrate intermediate during catalysis that appears to involve the non-reducing end glucosyl unit. For Ced3A, transglycosylation activity could be detected with the position isomers sophorose, cellobiose, and gentobiose, which share in common the non-reducing end glucosyl unit modified at the C1 position. This transglycosylase activity coupled with the hydrolysis of the pNP- glucose derivatives and sequential degradation of cellotriose argues that the non-reducing terminal glucose forms a covalent intermediate with the enzyme. This intermediate is then hydrolyzed to release glucose or the glucosyl unit transferred to another cellodextrin molecule under high substrate conditions during transglycosylation.
The production of five β-glucosidases by S. degradans is unusual, as most other cellulolytic organisms only produce one or a few β-glucosidases [25, 33]. One explanation for the multiple apparent β-glucosidase isozymes may be structural. Ced3A, Ced3B, and Bgl3C appear to be surface-attached, whereas Bgl1A, Bgl1B, and Cep94A are cytoplasmic. The surface-attached enzymes would act on externally produced cellobiose, whereas the cytoplasmic enzymes would act on internalized cellobiose. The ability to phosphorolytically cleave cellobiose using an enzyme with properties of a cytoplasmic enzyme argues that this bacterium has the ability to import cellobiose by a presently unidentified disaccharide transporter. This would also explain why expression of bgl1A, the gene for another apparent cytoplasmic enzyme, is also induced during growth on cellulose. The expression of these enzymes may also be different in that they may be induced by different substrates or under different conditions. Each of these genes appears to be expressed as individual transcriptional units.
The detection of a cellobiose phosphorylase in an obligate aerobe is unusual. Cellobiose phosphorylase was first found in Clostridium thermocellum, then later in other cellulolytic bacteria, including Cellvibrio gilvus [12, 31], Ruminococcus flavefaciens [3], Ruminococcus albus [18], Prevotella ruminicola [19], Clostridium thermocellum [1, 16, 22, 42], Clostridium stercorarium [29], Clostridium cellulolyticum [10], Thermotoga neapolitana [41], and Thermotoga maritime [28]. Most of these bacteria are anaerobes. These cellobiose phosphorylases are thought to function in energy conservation to minimize ATP consumption during fermentative metabolism. Full-length homologs of Cep94A are also present in the genomes of many other cellulolytic bacteria such as Teredinibacter turnerae [40], Celvibrio japonicus [7], and Fibrobacter succinogenes (http://genome.jgi-psf.org/fibsu/fibsu.download.html). Thus, phosphorolytic processing of cellobiose may be common among cellulolytic bacteria. The presence of this enzyme in these aerobes suggests that this enzyme may be important to conserve cellular energy under any stressful condition, such as nutrient limitation.
These results indicate that there are three possible mechanisms for this obligate aerobe to convert cellobiose to glucose phosphate. Highly expressed, surface-attached Ced3A and Ced3B (and to a lesser extent Bgl3C) hydrolytically cleave cellobiose and cellodextrins to glucose. Interestingly, little or no glucose or cellobiose accumulates in the medium when S. degradans 2-40 is grown on cellulose (Santos and Hutcheson, unpublished results). One of several glucose-transporter homologs present in the genome then would efficiently import this glucose with subsequent ATP-dependent phosphorylation by a glucokinase homolog to create glucose 6 phosphate. Alternatively, cellobiose could be imported by a presently unknown cellobiose transporter and hydrolytically converted to glucose via the activity of cytoplasmic Bgl1A and/or Bgl1B. The imported cellobiose could also be converted phosphorolytically to glucose 1-phosphate and glucose by the activity of Cep94A. A phosphoglucomutase homolog has been detected in the genome that would then catalyze the conversion of glucose 1-phosphate to glucose 6-phosphate. Subsequent metabolism of glucose 6-phosphate appears to occur via the Entner-Doudoroff (ED) pathway as the genome of this aerobic bacterium is Pseudomonas-like in character [7, 38], the homologs to the enzymes required for the ED pathway are present in the genome of this obligate aerobe (Hutcheson, unpublished results), and are expressed when the bacterium is actively metabolizing cellulose (Zhang and Hutcheson, in preparation).
The major pathway for cellobiose metabolism appears to be the hydrolytic pathway as the genes for β-glucosidases were highly expressed and more than 75% of the cellobiase activity of crude lysates of cellulose-grown cells was hydrolytic. Hydrolytic cleavage appears to be the dominant mechanism for processing cellobiose, even among cellulolytic anaerobic bacteria. Lou et al. [19], for example, found that the rate of hydrolytic cleavage of cellobiose in the P. ruminicola was three-fold faster than the rate of phosphorolytic cleavage. The presence of multiple pathways for processing cellobiose and other cellodextrins provides metabolic flexibility so that cellobiose can be hydrolyzed on the cell surface and directly imported as glucose or imported as cellobiose/cellodextrin and then either hydrolytically or phosphorolytically processed to glucose.
Acknowledgments
This work was supported by a grant from the National Science Foundation (DEB0621297) to SWH. YHM was partially supported by a Korea Research Foundation Grant (KRF-2008-357-D00088).