-
PDF
- Split View
-
Views
-
Cite
Cite
Jyotisna Saxena, Ralph S Tanner, Effect of trace metals on ethanol production from synthesis gas by the ethanologenic acetogen, Clostridium ragsdalei, Journal of Industrial Microbiology and Biotechnology, Volume 38, Issue 4, 1 April 2011, Pages 513–521, https://doi.org/10.1007/s10295-010-0794-6
- Share Icon Share
Abstract
The effect of trace metal ions (Co2+, Cu2+, Fe2+, Mn2+, Mo6+, Ni2+, Zn2+, SeO4 − and WO4 −) on growth and ethanol production by an ethanologenic acetogen, Clostridium ragsdalei was investigated in CO:CO2-grown cells. A standard acetogen medium (ATCC medium no. 1754) was manipulated by varying the concentrations of trace metals in the media. Increasing the individual concentrations of Ni2+, Zn2+, SeO4 − and WO4 − from 0.84, 6.96, 1.06, and 0.68 μM in the standard trace metals solution to 8.4, 34.8, 5.3, and 6.8 μM, respectively, increased ethanol production from 35.73 mM under standard metals concentration to 176.5, 187.8, 54.4, and 72.3 mM, respectively. Nickel was necessary for growth of C. ragsdalei. Growth rate (μ) of C. ragsdalei improved from 0.34 to 0.49 (day−1), and carbon monoxide dehydrogenase (CODH) and hydrogenase (H2ase)-specific activities improved from 38.45 and 0.35 to 48.5 and 1.66 U/mg protein, respectively, at optimum concentration of Ni2+. At optimum concentrations of WO4 − and SeO4 −, formate dehydrogenase (FDH) activity improved from 32.3 to 42.6 and 45.4 U/mg protein, respectively. Ethanol production and the activity of FDH reduced from 35 mM and 32.3 U/mg protein to 1.14 mM and 8.79 U/mg protein, respectively, upon elimination of WO4 − from the medium. Although increased concentration of Zn2+ enhanced growth and ethanol production, the activities of CODH, FDH, H2ase and alcohol dehydrogenase (ADH) were not affected by varying the Zn2+ concentration. Omitting Fe2+ from the medium decreased ethanol production from 35.7 to 6.30 mM and decreased activities of CODH, FDH, H2ase and ADH from 38.5, 32.3, 0.35, and 0.68 U/mg protein to 9.07, 7.01, 0.10, and 0.24 U/mg protein, respectively. Ethanol production improved from 35 to 54 mM when Cu2+ was removed from the medium. The optimization of trace metals concentration in the fermentation medium improved enzyme activities (CODH, FDH, and H2ase), growth and ethanol production by C. ragsdalei.
Introduction
The environmental concerns over the use of petroleum-based fuels and their limited supplies have generated a great interest in producing ethanol as an alternative fuel. The use of bioethanol (ethanol from biomass) reduces the dependence on imported oil, supports agriculture, and limits greenhouse gas emissions. In the United States, bioethanol is primarily produced from the fermentation of corn starch, which is presently facing critical issues such as potential diversion of food and fodder-grade feedstocks towards biofuel production, its indirect effects on land-use change, and its limited supply [24]. Hence, to make the bioethanol production processes sustainable, rigorous criteria must be considered and best practices in terms of choice of feedstocks and its direct and indirect environmental and socio-economic effects should be utilized [11]. Bioethanol produced from lignocellulosic biomass grown on agriculturally marginal lands (e.g., switchgrass) and waste biomass (e.g., agricultural and forestry wastes) could compete with present supplies [21] and provide significant environmental and economic benefits over corn starch-based ethanol [11].
Lignocellulosic biomass can be converted to ethanol by direct fermentation of sugars obtained by acidic/enzymatic hydrolysis of the biomass (cellulosic ethanol) [19]. Although this process has tremendous potential, and over the past several years great advances have been made in this area [17], there are several disadvantages associated with this technology, such as the difficulties in handling and converting diverse sources of biomass to ethanol, and the lignin component of biomass is left unutilized. A promising approach for conversion of biomass to ethanol is the stepwise process of gasification and microbial fermentation [30]. In gasification, all biomass components including lignin are gasified to synthesis gas (syngas: a mixture of primarily CO, CO2, and H2) [30]. Syngas is fermented to several commodity chemicals, including ethanol by a group of obligatory anaerobic bacteria known as acetogens [30]. As a feed-stock flexible process, syngas fermentation can utilize a wide variety of biomass sources that are combustible and non-food based, such as municipal solid waste, tires, and residual agricultural biomass [30].
Acetogens utilize the acetyl-CoA/Wood-Ljungdahl pathway for production of acetyl-CoA, a precursor of cellular biomass, acetate, and ethanol. Key enzymes of this pathway include formate dehydrogenase (FDH), bifunctional carbon monoxide dehydrogenase/acetyl CoA synthase (CODH/ACS), and hydrogenase (H2ase), all of which are metalloenzymes [9]. In addition, solvent-producing clostridia also have iron- and zinc-containing alcohol dehydrogenase (ADH) [15, 26] that plays a key role by catalyzing the reduction of acetyl CoA to ethanol [6].
Considering the key role of metalloenzymes in the metabolism of acetogens, a study was undertaken to investigate the effect of trace metals (Co2+, Cu2+, Fe2+, Mn2+, Mo6+, Ni2+, Zn2+, SeO4 −, and WO4 −) on growth, production of ethanol, production of acetate and enzyme activities (CODH, FDH, H2ase, and ADH) by a recently isolated ethanologenic acetogen, Clostridium ragsdalei using syngas components. C. ragsdalei was isolated from duck pond sediment, and produces ethanol and acetate from syngas components [12] (US Pat. No. US 2008/0057554 A1). It was hypothesized that the optimization of trace metal concentrations in the culture medium might enhance the activity of metalloenzymes, thus improving growth and ethanol production by C. ragsdalei.
Materials and methods
Bacterial strain, media, and growth conditions
The bacterium used in this study was Clostridium ragsdalei (ATCC PTA 7826), which was isolated in our laboratory from duck pond sediment in Brandt Park, Norman, OK, USA. The standard acetogen medium (ATCC medium no. 1754) for cultivation of C. ragsdalei was prepared using strict anaerobic techniques [2] under an atmosphere of N2:CO2 (80:20). The medium (per liter) contained: mineral solution, 25 ml [29]; trace metal solution, 10 ml [29]; vitamin solution, 10 ml [29]; 2-(N-morpholino)ethanesulfonic acid (MES), 20 g; yeast extract (Difco, Becton Dickinson, Sparks, M.D.), 0.5 g; 0.1% resazurin, 0.1 ml and cysteine-sulfide reducing agent, 2.5 ml [29]. The pH of the medium was adjusted to 6.1 using NaOH. The trace metal solution (per liter) contained MnSO4·H2O (1.0 g), Fe(NH4)2(SO4)2·6H2O (0.8 g), CoCl2·6H2O (0.2 g), ZnSO4·7H2O (0.2 g), CuCl2·2H2O (0.02 g), NiCl2·6H2O (0.02 g), Na2MoO4·2H2O (0.02 g), Na2SeO4 (0.02 g), and Na2WO4 (0.02 g). The concentrations of individual trace metals in the media are presented in Table 1. Metals present in the yeast extract were removed as metal ion phosphates using a procedure derived from dephosphorylating media components [20].
Concentration of trace metals in standard growth medium, ATCC 1754 (1X)
Trace metal . | (μM) . |
---|---|
Co | 8.40 |
Cu | 1.17 |
Fe | 20.40 |
Mn | 59.17 |
Mo | 0.83 |
Ni | 0.84 |
Se | 1.06 |
W | 0.68 |
Zn | 6.96 |
Trace metal . | (μM) . |
---|---|
Co | 8.40 |
Cu | 1.17 |
Fe | 20.40 |
Mn | 59.17 |
Mo | 0.83 |
Ni | 0.84 |
Se | 1.06 |
W | 0.68 |
Zn | 6.96 |
Concentration of trace metals in standard growth medium, ATCC 1754 (1X)
Trace metal . | (μM) . |
---|---|
Co | 8.40 |
Cu | 1.17 |
Fe | 20.40 |
Mn | 59.17 |
Mo | 0.83 |
Ni | 0.84 |
Se | 1.06 |
W | 0.68 |
Zn | 6.96 |
Trace metal . | (μM) . |
---|---|
Co | 8.40 |
Cu | 1.17 |
Fe | 20.40 |
Mn | 59.17 |
Mo | 0.83 |
Ni | 0.84 |
Se | 1.06 |
W | 0.68 |
Zn | 6.96 |
Clostridium ragsdalei was grown in anaerobic culture tubes (Bellco Glass, Inc., Vineland, NJ, USA) containing 5 ml of medium and a gas mixture of CO:N2:CO2 (70:24:6 pressurized to 230 kPa gauge) in the head space. Since CO is sparingly soluble in water, the tubes were incubated on their sides at 36°C on a rotary shaker (100–120 rpm) to maximize gas–liquid mixing.
Fermentation experiment
Batch fermentation experiments were conducted to examine the effect of trace metals (Co2+, Cu2+, Fe2+, Mn2+, Mo6+, Ni2+, Zn2+, SeO4 −, and WO4 −) on growth and ethanol production by C. ragsdalei. The concentration of trace metals in standard trace metals solution (Table 1) is denoted as 1X throughout the study. The concentrations of each trace metal was either increased ten-fold (10X) with respect to standard metal concentration (1X) (Table 1) or eliminated (0X) from the media. Once effective metals for growth and ethanol production were identified, different concentrations (1X, 5X, 10X, and 20X) of each metal were titrated to find their optimal concentrations for enhanced ethanol production. Fermentation experiments were performed in triplicate in 160-ml Wheaton serum vials containing 10 ml of medium. C. ragsdalei cells were transferred three times in the media with each trace metal concentrations to minimize the metal ion carry over via inoculum into the experimental media. A 1% inoculum was used in all experiments. The bottles were fed with CO (440-kPa gauge) and incubated at 36°C on a rotary shaker. Once the cells were grown, the headspace gases were exchanged with CO (440-kPa gauge) every 24 h for 10 days. At the end of the experiments, final pH and growth were recorded and the samples were analyzed for acetate and ethanol.
A time course experiment was conducted on the optimized concentrations metals and to study their effects on growth and fermentation products formed over a period of 10 days. The time course experiment was conducted in 500-ml Wheaton serum bottles with 50 ml of medium. Experiments were conducted according to the procedure described above, except that samples (1.5 ml) were collected every 24 h for 10 days. The samples were analyzed for pH, growth, and fermentation products.
Analytical methods
Growth was monitored by measuring optical density (OD) at 600 nm with a Beckman Coulter DU 640 spectrophotometer (Bausch and Lomb, Rochester, NY). Growth rate (μ) was derived from OD data as described elsewhere [4]. The fermentation products (i.e., acetate and ethanol) were analyzed quantitatively using a Shimadzu GC-8A gas chromatograph (Shimadzu, Maryland, USA) equipped with a flame ionization detector (FID) and helium was used as the carrier gas. The column and the injector/detector temperatures were maintained at 155 and 200°C, respectively. The glass column (2.6 m) was packed with Carbopack BDA (80/120 mesh) and 4% Carbowax 20 M resin (Supelco Inc., Bellefonte, PA). Data were analyzed with a Shimadzu C-R8A integrator.
Enzyme assays
Enzyme activities (FDH, CODH, H2ase, and ADH) were determined spectrophotometrically as the substrate-dependent reduction of methyl viologen (MV) in 2-ml cuvettes as described previously [18]. The cultures in the enzyme assays were grown in 500-ml Wheaton serum bottles with 50 ml of media as stated above. The exponential phase cultures were used for enzyme assays. Protein concentrations were estimated using bicinchoninic acid method [28] with bovine serum albumin as a standard.
Results
Screening of trace metals for their effect on ethanol production
The effect of different trace metals on growth rate and production of ethanol and acetate by C. ragsdalei is presented in Table 2. C. ragsdalei produced 35 mM of ethanol and 9 mM of acetate under the standard medium (1X) condition. At higher concentrations (10X) of Ni2+, Zn2+, SeO4 − and WO4 − ethanol production increased to 176, 190, 75, and 55 mM, respectively. Ethanol production decreased to 27.01, 6.30, 22.02, and 1.55 mM when Co2+, Fe2+, Mo2+ and WO4 − were eliminated (0X) from the media, indicating that these metals were required for ethanol production. An increase in ethanol production to 54 mM was noticed when Cu2+ was removed (0X) from the media. Ethanol production did not change considerably at higher concentration (10X) of Cu2+, Co2+, Fe2+, and Mn2+, and also when Mn2+, Zn2+, and SeO4 − were omitted (0X) from the media. A decrease in ethanol production to 23.64 mM was observed at higher concentration (10X) of Mo2+.
Effect of trace metals on growth rate (μ), final pH (pHf), production of ethanol, and production of acetate by C. ragsdalei after 10 days of growth
Trace metals . | Growth rate (day−1) . | pHf . | Ethanol (mM) . | Acetate (mM) . |
---|---|---|---|---|
1Xa | 0.34 ± 0.006 | 5.9 | 35.73 ± 2.05 | 8.87 ± 0.31 |
Ni | ||||
0X | No growth | 6.1 | No growth | No growth |
10X | 0.49 ± 0.002 | 5.8 | 176.52 ± 5.62 | 14.96 ± 2.78 |
Zn | ||||
0X | 0.34 ± 0.018 | 5.9 | 33.48 ± 1.42 | 11.20 ± 3.24 |
10X | 0.48 ± 0.002 | 5.6 | 187.80 ± 8.56 | 18.56 ± 2.36 |
Se | ||||
0X | 0.30 ± 0.012 | 5.9 | 38.32 ± 2.12 | 9.56 ± 1.34 |
10X | 0.30 ± 0.012 | 5.9 | 54.35 ± 3.23 | 8.64 ± 0.96 |
W | ||||
0X | 0.30 ± 0.015 | 5.9 | 1.14 ± 0.04 | 18.56 ± 0.75 |
10X | 0.44 ± 0.003 | 5.9 | 72.29 ± 1.80 | 10.87 ± 0.82 |
Cu | ||||
0X | 0.30 ± 0.002 | 5.9 | 54.09 ± 2.62 | 11.83 ± 1.49 |
10X | 0.30 ± 0.008 | 5.9 | 36.63 ± 1.57 | 9.17 ± 1.27 |
Fe | ||||
0X | 0.33 ± 0.004 | 5.9 | 6.30 ± 0.92 | 15.34 ± 0.30 |
10X | 0.34 ± 0.007 | 5.9 | 35.87 ± 1.72 | 8.50 ± 1.15 |
Co | ||||
0X | 0.31 ± 0.001 | 5.9 | 27.01 ± 1.31 | 7.02 ± 0.85 |
10X | 0.34 ± 0.001 | 5.9 | 37.34 ± 0.69 | 6.70 ± 1.03 |
Mo | ||||
0X | 0.31 ± 0.004 | 5.9 | 22.02 ± 0.90 | 7.38 ± 0.65 |
10X | 0.31 ± 0.001 | 5.9 | 23.64 ± 1.42 | 8.06 ± 0.34 |
Mn | ||||
0X | 0.31 ± 0.001 | 5.9 | 33.11 ± 1.46 | 8.75 ± 0.35 |
10X | 0.31 ± 0.001 | 5.9 | 36.78 ± 0.24 | 9.11 ± 0.04 |
Trace metals . | Growth rate (day−1) . | pHf . | Ethanol (mM) . | Acetate (mM) . |
---|---|---|---|---|
1Xa | 0.34 ± 0.006 | 5.9 | 35.73 ± 2.05 | 8.87 ± 0.31 |
Ni | ||||
0X | No growth | 6.1 | No growth | No growth |
10X | 0.49 ± 0.002 | 5.8 | 176.52 ± 5.62 | 14.96 ± 2.78 |
Zn | ||||
0X | 0.34 ± 0.018 | 5.9 | 33.48 ± 1.42 | 11.20 ± 3.24 |
10X | 0.48 ± 0.002 | 5.6 | 187.80 ± 8.56 | 18.56 ± 2.36 |
Se | ||||
0X | 0.30 ± 0.012 | 5.9 | 38.32 ± 2.12 | 9.56 ± 1.34 |
10X | 0.30 ± 0.012 | 5.9 | 54.35 ± 3.23 | 8.64 ± 0.96 |
W | ||||
0X | 0.30 ± 0.015 | 5.9 | 1.14 ± 0.04 | 18.56 ± 0.75 |
10X | 0.44 ± 0.003 | 5.9 | 72.29 ± 1.80 | 10.87 ± 0.82 |
Cu | ||||
0X | 0.30 ± 0.002 | 5.9 | 54.09 ± 2.62 | 11.83 ± 1.49 |
10X | 0.30 ± 0.008 | 5.9 | 36.63 ± 1.57 | 9.17 ± 1.27 |
Fe | ||||
0X | 0.33 ± 0.004 | 5.9 | 6.30 ± 0.92 | 15.34 ± 0.30 |
10X | 0.34 ± 0.007 | 5.9 | 35.87 ± 1.72 | 8.50 ± 1.15 |
Co | ||||
0X | 0.31 ± 0.001 | 5.9 | 27.01 ± 1.31 | 7.02 ± 0.85 |
10X | 0.34 ± 0.001 | 5.9 | 37.34 ± 0.69 | 6.70 ± 1.03 |
Mo | ||||
0X | 0.31 ± 0.004 | 5.9 | 22.02 ± 0.90 | 7.38 ± 0.65 |
10X | 0.31 ± 0.001 | 5.9 | 23.64 ± 1.42 | 8.06 ± 0.34 |
Mn | ||||
0X | 0.31 ± 0.001 | 5.9 | 33.11 ± 1.46 | 8.75 ± 0.35 |
10X | 0.31 ± 0.001 | 5.9 | 36.78 ± 0.24 | 9.11 ± 0.04 |
a 1X standard concentrations of all the trace metals, 0X elimination of trace metal, 10X tenfold higher metal concentration than 1X
Effect of trace metals on growth rate (μ), final pH (pHf), production of ethanol, and production of acetate by C. ragsdalei after 10 days of growth
Trace metals . | Growth rate (day−1) . | pHf . | Ethanol (mM) . | Acetate (mM) . |
---|---|---|---|---|
1Xa | 0.34 ± 0.006 | 5.9 | 35.73 ± 2.05 | 8.87 ± 0.31 |
Ni | ||||
0X | No growth | 6.1 | No growth | No growth |
10X | 0.49 ± 0.002 | 5.8 | 176.52 ± 5.62 | 14.96 ± 2.78 |
Zn | ||||
0X | 0.34 ± 0.018 | 5.9 | 33.48 ± 1.42 | 11.20 ± 3.24 |
10X | 0.48 ± 0.002 | 5.6 | 187.80 ± 8.56 | 18.56 ± 2.36 |
Se | ||||
0X | 0.30 ± 0.012 | 5.9 | 38.32 ± 2.12 | 9.56 ± 1.34 |
10X | 0.30 ± 0.012 | 5.9 | 54.35 ± 3.23 | 8.64 ± 0.96 |
W | ||||
0X | 0.30 ± 0.015 | 5.9 | 1.14 ± 0.04 | 18.56 ± 0.75 |
10X | 0.44 ± 0.003 | 5.9 | 72.29 ± 1.80 | 10.87 ± 0.82 |
Cu | ||||
0X | 0.30 ± 0.002 | 5.9 | 54.09 ± 2.62 | 11.83 ± 1.49 |
10X | 0.30 ± 0.008 | 5.9 | 36.63 ± 1.57 | 9.17 ± 1.27 |
Fe | ||||
0X | 0.33 ± 0.004 | 5.9 | 6.30 ± 0.92 | 15.34 ± 0.30 |
10X | 0.34 ± 0.007 | 5.9 | 35.87 ± 1.72 | 8.50 ± 1.15 |
Co | ||||
0X | 0.31 ± 0.001 | 5.9 | 27.01 ± 1.31 | 7.02 ± 0.85 |
10X | 0.34 ± 0.001 | 5.9 | 37.34 ± 0.69 | 6.70 ± 1.03 |
Mo | ||||
0X | 0.31 ± 0.004 | 5.9 | 22.02 ± 0.90 | 7.38 ± 0.65 |
10X | 0.31 ± 0.001 | 5.9 | 23.64 ± 1.42 | 8.06 ± 0.34 |
Mn | ||||
0X | 0.31 ± 0.001 | 5.9 | 33.11 ± 1.46 | 8.75 ± 0.35 |
10X | 0.31 ± 0.001 | 5.9 | 36.78 ± 0.24 | 9.11 ± 0.04 |
Trace metals . | Growth rate (day−1) . | pHf . | Ethanol (mM) . | Acetate (mM) . |
---|---|---|---|---|
1Xa | 0.34 ± 0.006 | 5.9 | 35.73 ± 2.05 | 8.87 ± 0.31 |
Ni | ||||
0X | No growth | 6.1 | No growth | No growth |
10X | 0.49 ± 0.002 | 5.8 | 176.52 ± 5.62 | 14.96 ± 2.78 |
Zn | ||||
0X | 0.34 ± 0.018 | 5.9 | 33.48 ± 1.42 | 11.20 ± 3.24 |
10X | 0.48 ± 0.002 | 5.6 | 187.80 ± 8.56 | 18.56 ± 2.36 |
Se | ||||
0X | 0.30 ± 0.012 | 5.9 | 38.32 ± 2.12 | 9.56 ± 1.34 |
10X | 0.30 ± 0.012 | 5.9 | 54.35 ± 3.23 | 8.64 ± 0.96 |
W | ||||
0X | 0.30 ± 0.015 | 5.9 | 1.14 ± 0.04 | 18.56 ± 0.75 |
10X | 0.44 ± 0.003 | 5.9 | 72.29 ± 1.80 | 10.87 ± 0.82 |
Cu | ||||
0X | 0.30 ± 0.002 | 5.9 | 54.09 ± 2.62 | 11.83 ± 1.49 |
10X | 0.30 ± 0.008 | 5.9 | 36.63 ± 1.57 | 9.17 ± 1.27 |
Fe | ||||
0X | 0.33 ± 0.004 | 5.9 | 6.30 ± 0.92 | 15.34 ± 0.30 |
10X | 0.34 ± 0.007 | 5.9 | 35.87 ± 1.72 | 8.50 ± 1.15 |
Co | ||||
0X | 0.31 ± 0.001 | 5.9 | 27.01 ± 1.31 | 7.02 ± 0.85 |
10X | 0.34 ± 0.001 | 5.9 | 37.34 ± 0.69 | 6.70 ± 1.03 |
Mo | ||||
0X | 0.31 ± 0.004 | 5.9 | 22.02 ± 0.90 | 7.38 ± 0.65 |
10X | 0.31 ± 0.001 | 5.9 | 23.64 ± 1.42 | 8.06 ± 0.34 |
Mn | ||||
0X | 0.31 ± 0.001 | 5.9 | 33.11 ± 1.46 | 8.75 ± 0.35 |
10X | 0.31 ± 0.001 | 5.9 | 36.78 ± 0.24 | 9.11 ± 0.04 |
a 1X standard concentrations of all the trace metals, 0X elimination of trace metal, 10X tenfold higher metal concentration than 1X
Acetate production increased to 15 and 18.6 mM at increased concentrations (10X) of Ni2+ and Zn2+, respectively. An increase in acetate production to 15.34 and 18.64 mM was also observed when Fe2+ and WO4 − were eliminated (0X) from the media. There were no appreciable changes in acetate production at greater concentration (10X) of Co2+, Cu2+, Fe2+, Mn2+, Mo6+, SeO4 −, and WO4 −, and also when Co2+, Cu2+, Mn2+, Mo6+, Zn2+, and SeO4 − were omitted (0X) from the media.
The growth rate of C. ragsdalei improved at increased concentrations (10X) of Ni2+, Zn2+, and WO4 2−. Nickel was necessary for growth of C. ragsdalei. The growth rate of C. ragsdalei for remaining metals (Co2+, Cu2+, Fe2+, Mn2+, Mo6+, and SeO4 −) was similar to that with standard metals (1X) in growth medium. The final pH of the media was similar under the various metal concentrations.
Effect of optimized concentrations of Ni2+, Zn2+, WO4 −, and SeO4 − on growth rate, ethanol production, and acetate production
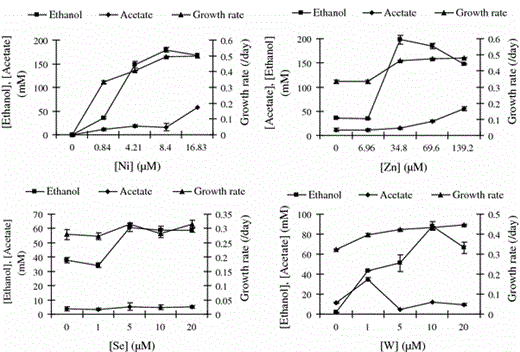
Effect of different concentrations of nickel (Ni), zinc (Zn), selenite (Se), and tungstate (W), on growth rate (/day), production of ethanol, and production of acetate by C. ragsdalei
Time course experiment
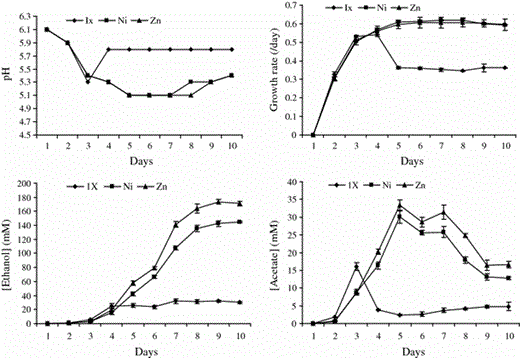
Effect of optimal concentrations of nickel (Ni) and zinc (Zn) on final pH, growth rate (/day), production of ethanol, and production of acetate by C. ragsdalei
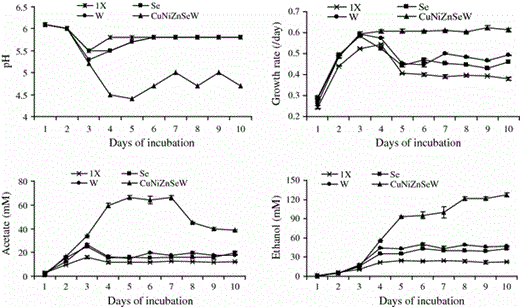
Effect of optimal concentrations of selenate (Se), tungstate (W), and optimal concentrations of copper-nickel-zinc-selenite-tungstate combined together (CuNiZnSeW) on final pH, growth rate (/day), production of ethanol, and production of acetate by C. ragsdalei
Effect of Ni2+, Zn2+, SeO4 −, and WO4 − on enzyme activities in Clostridium ragsdalei
The optimum concentration of Ni2+ stimulated the activities of CODH and H2ase; whereas, the activity of FDH was enhanced at optimum concentrations of SeO4 − and WO4 − (Table 3). Inclusion of optimum concentrations of SeO4 − and WO4 − together in the medium had the same effect on FDH activity as the individual additions of SeO4 − and WO4 − (Table 3). Enzyme activity of CODH, FDH, H2ase, and ADH were reduced when Fe2+ was removed from the medium, whereas at increased concentration of Fe2+ (10X), there was no change in enzyme activities as compared to those under the standard metal concentration (1X) (Table 3). Although the optimum concentration of Zn2+ increased the growth and ethanol production (Fig. 1), enzyme activities were not affected by the concentration of Zn2+ (Table 3).
Effect of trace metals on activities (U/mg protein) of carbon monoxide dehydrogenase (CODH), formate dehydrogenase (FDH), hydrogenase (H2ase), and alcohol dehydrogenase (ADH) by C. ragsdalei
Growth conditionsa . | FDH . | CODH . | H2ase . | ADH . |
---|---|---|---|---|
1X | 32.25 ± 1.41 | 38.45 ± 1.68 | 0.35 ± 0.01 | 0.68 ± 0.01 |
Ni | ||||
0X | No growth | No growth | No growth | No growth |
10X | 34.6 ± 1.13 | 48.46 ± 1.57 | 1.66 ± 0.06 | 0.65 ± 0.12 |
Se | ||||
0X | 31.97 ± 0.92 | 38.01 ± 0.49 | 0.32 ± 0.03 | 0.83 ± 0.06 |
5X | 42.62 ± 0.73 | 37.07 ± 3.35 | 0.28 ± 0.01 | 0.61 ± 0.03 |
W | ||||
0X | 8.79 ± 0.16 | 36.24 ± 0.29 | 0.33 ± 0.03 | 0.77 ± 0.01 |
10X | 45.44 ± 0.21 | 33.57 ± 0.79 | 0.41 ± 0.08 | 0.68 ± 0.05 |
Se (5X) + W (10X) | 35.46 ± 0.34 | 36.25 ± 0.41 | 0.31 ± 0.02 | 0.71 ± 0.13 |
Zn | ||||
0X | 31.96 ± 1.21 | 35.64 ± 2.50 | 0.32 ± 0.03 | 0.65 ± 0.03 |
5X | 34.02 ± 0.08 | 36.81 ± 2.39 | 0.44 ± 0.04 | 0.75 ± 0.03 |
Fe | ||||
0X | 7.01 ± 0.62 | 9.07 ± 0.04 | 0.10 ± 0.04 | 0.24 ± 0.02 |
10X | 35.65 ± 0.33 | 37.51 ± 0.50 | 0.39 ± 0.05 | 0.63 ± 0.01 |
Growth conditionsa . | FDH . | CODH . | H2ase . | ADH . |
---|---|---|---|---|
1X | 32.25 ± 1.41 | 38.45 ± 1.68 | 0.35 ± 0.01 | 0.68 ± 0.01 |
Ni | ||||
0X | No growth | No growth | No growth | No growth |
10X | 34.6 ± 1.13 | 48.46 ± 1.57 | 1.66 ± 0.06 | 0.65 ± 0.12 |
Se | ||||
0X | 31.97 ± 0.92 | 38.01 ± 0.49 | 0.32 ± 0.03 | 0.83 ± 0.06 |
5X | 42.62 ± 0.73 | 37.07 ± 3.35 | 0.28 ± 0.01 | 0.61 ± 0.03 |
W | ||||
0X | 8.79 ± 0.16 | 36.24 ± 0.29 | 0.33 ± 0.03 | 0.77 ± 0.01 |
10X | 45.44 ± 0.21 | 33.57 ± 0.79 | 0.41 ± 0.08 | 0.68 ± 0.05 |
Se (5X) + W (10X) | 35.46 ± 0.34 | 36.25 ± 0.41 | 0.31 ± 0.02 | 0.71 ± 0.13 |
Zn | ||||
0X | 31.96 ± 1.21 | 35.64 ± 2.50 | 0.32 ± 0.03 | 0.65 ± 0.03 |
5X | 34.02 ± 0.08 | 36.81 ± 2.39 | 0.44 ± 0.04 | 0.75 ± 0.03 |
Fe | ||||
0X | 7.01 ± 0.62 | 9.07 ± 0.04 | 0.10 ± 0.04 | 0.24 ± 0.02 |
10X | 35.65 ± 0.33 | 37.51 ± 0.50 | 0.39 ± 0.05 | 0.63 ± 0.01 |
Specific activity = 1 μmol of substrate reduced.min−1 mg−1 of protein
a 1X standard concentrations of all the trace metals, 0X elimination of trace metals, 5X fivefold higher concentration than 1X, 10X tenfold higher concentration than 1X
Effect of trace metals on activities (U/mg protein) of carbon monoxide dehydrogenase (CODH), formate dehydrogenase (FDH), hydrogenase (H2ase), and alcohol dehydrogenase (ADH) by C. ragsdalei
Growth conditionsa . | FDH . | CODH . | H2ase . | ADH . |
---|---|---|---|---|
1X | 32.25 ± 1.41 | 38.45 ± 1.68 | 0.35 ± 0.01 | 0.68 ± 0.01 |
Ni | ||||
0X | No growth | No growth | No growth | No growth |
10X | 34.6 ± 1.13 | 48.46 ± 1.57 | 1.66 ± 0.06 | 0.65 ± 0.12 |
Se | ||||
0X | 31.97 ± 0.92 | 38.01 ± 0.49 | 0.32 ± 0.03 | 0.83 ± 0.06 |
5X | 42.62 ± 0.73 | 37.07 ± 3.35 | 0.28 ± 0.01 | 0.61 ± 0.03 |
W | ||||
0X | 8.79 ± 0.16 | 36.24 ± 0.29 | 0.33 ± 0.03 | 0.77 ± 0.01 |
10X | 45.44 ± 0.21 | 33.57 ± 0.79 | 0.41 ± 0.08 | 0.68 ± 0.05 |
Se (5X) + W (10X) | 35.46 ± 0.34 | 36.25 ± 0.41 | 0.31 ± 0.02 | 0.71 ± 0.13 |
Zn | ||||
0X | 31.96 ± 1.21 | 35.64 ± 2.50 | 0.32 ± 0.03 | 0.65 ± 0.03 |
5X | 34.02 ± 0.08 | 36.81 ± 2.39 | 0.44 ± 0.04 | 0.75 ± 0.03 |
Fe | ||||
0X | 7.01 ± 0.62 | 9.07 ± 0.04 | 0.10 ± 0.04 | 0.24 ± 0.02 |
10X | 35.65 ± 0.33 | 37.51 ± 0.50 | 0.39 ± 0.05 | 0.63 ± 0.01 |
Growth conditionsa . | FDH . | CODH . | H2ase . | ADH . |
---|---|---|---|---|
1X | 32.25 ± 1.41 | 38.45 ± 1.68 | 0.35 ± 0.01 | 0.68 ± 0.01 |
Ni | ||||
0X | No growth | No growth | No growth | No growth |
10X | 34.6 ± 1.13 | 48.46 ± 1.57 | 1.66 ± 0.06 | 0.65 ± 0.12 |
Se | ||||
0X | 31.97 ± 0.92 | 38.01 ± 0.49 | 0.32 ± 0.03 | 0.83 ± 0.06 |
5X | 42.62 ± 0.73 | 37.07 ± 3.35 | 0.28 ± 0.01 | 0.61 ± 0.03 |
W | ||||
0X | 8.79 ± 0.16 | 36.24 ± 0.29 | 0.33 ± 0.03 | 0.77 ± 0.01 |
10X | 45.44 ± 0.21 | 33.57 ± 0.79 | 0.41 ± 0.08 | 0.68 ± 0.05 |
Se (5X) + W (10X) | 35.46 ± 0.34 | 36.25 ± 0.41 | 0.31 ± 0.02 | 0.71 ± 0.13 |
Zn | ||||
0X | 31.96 ± 1.21 | 35.64 ± 2.50 | 0.32 ± 0.03 | 0.65 ± 0.03 |
5X | 34.02 ± 0.08 | 36.81 ± 2.39 | 0.44 ± 0.04 | 0.75 ± 0.03 |
Fe | ||||
0X | 7.01 ± 0.62 | 9.07 ± 0.04 | 0.10 ± 0.04 | 0.24 ± 0.02 |
10X | 35.65 ± 0.33 | 37.51 ± 0.50 | 0.39 ± 0.05 | 0.63 ± 0.01 |
Specific activity = 1 μmol of substrate reduced.min−1 mg−1 of protein
a 1X standard concentrations of all the trace metals, 0X elimination of trace metals, 5X fivefold higher concentration than 1X, 10X tenfold higher concentration than 1X
Discussion
Acetogens utilize the acetyl-CoA/Wood-Ljungdahl pathway for production of acetyl-CoA, a precursor of cell biomass, acetate, and ethanol [9]. Hence, to improve the growth and fermentation products, it is necessary to improve the carbon flow to acetyl-CoA, which requires upstream manipulations in this pathway. In this study, the activities of the key metalloenzymes of this pathway were enhanced by optimizing the concentrations of trace metals in the growth medium, which improved growth and ethanol production by C. ragsdalei.
The ethanol production and enzyme activities (CODH, FDH, H2ase, and ADH) of C. ragsdalei were reduced when Fe2+ was removed (0X) from the medium. All the key metalloenzymes of acetyl-CoA pathway (CODH, FDH, and H2ase) are iron-sulfur proteins [5, 10, 32]. Hence, a reduction in enzyme activities (CODH, FDH, H2ase, and ADH) and ethanol production by C. ragsdalei upon elimination of Fe2+ from the growth media was anticipated. However, increasing Fe2+ concentration (10X) had no effect on the growth, production of ethanol, or production of acetate, which indicates that Fe2+ concentration in the growth medium became saturating at a concentration exceeding 20.4 μM (1X) for the growth and metabolism of C. ragsdalei.
FDH catalyzes the first reaction on the methyl branch of the acetyl Co-A pathway, where it reduces CO2 to formate [9]. The metal co-factors of FDH in clostridia are reported to be variable, where iron, tungsten, selenium, and/or molybdenum have been shown to be the components of FDH [16, 31, 32]. FDH in acetogens contain selenium and tungsten [14, 31, 32]; whereas, in non-acetogenic clostridia such C. formicoaceticum, molybdenum-containing FDH is present [16]. In acetate-producing acetogens, the presence of tungsten and selenium in FDH has been suggested to function as cofactors, promoting the FDH-mediated reduction of CO2 [32]. C. ragsdalei produced acetate and ethanol as the fermentation products when grown on CO:CO2. Whole-cell FDH activity of C. ragsdalei was enhanced at the optimum concentration of tungstate and selenite, and reduced upon elimination of tungstate and iron, suggesting that FDH of C. ragsdalei may be a tungsten-selenium-iron protein.
At the optimum concentration of Ni2+, an increase in ethanol production by C. ragsdalei was observed that was associated with increased CODH activity. CODH in acetogenic bacteria such as M. thermoacetica occurs as a bifunctional CODH/ACS enzyme where CODH generates CO from CO2, and the ACS combines the CO with CoA and a methyl group to form acetyl-CoA [10]. Both CODH and ACS contain iron-nickel-sulfur metal clusters (CODH: Fe-[NiFe3S4] and ACS: [Fe4S4]-Cys-Gly-Cys-Ni-Cys-Gly-Cys-Ni) at their active sites [10]. Therefore, CODH/ACS and the associated metals (Fe and Ni) play a key role in the metabolism of acetogens.
In addition to Fe2+ and Ni2+, Cu2+ also regulates the activity of ACS in acetogens, where Cu2+ has a negative effect on ACS activity [3, 10, 27]. The metal cluster of ACS contains binuclear metal sites: a proximal metal site (Mp) and a distal metal site (Md), both of which contain Ni2+ under the condition where no Cu2+ is present in the growth medium. However, in the presence of Cu2+, the proximal metal site in the A-cluster can undergo a metal substitution resulting in a binuclear site composition of Cu–Ni and Ni–Ni [10, 27]. The Ni–Ni form of ACS is active whereas Cu-Ni form of enzyme is inactive, which eliminates ACS activity (measured by ability to generate the NiFeC EPR signal) [27]. Although ACS activity in the present study was not measured, an increase in ethanol production was observed upon removing Cu2+ from the growth medium, which could be due to inhibition of ACS activity by Cu2+. Increasing the concentration of Cu2+ had no change on growth and product formation, suggesting Cu2+ concentration in standard trace metals solution (1X) was enough to cause ACS inhibition, probably due to the fact that the cells of acetogenic bacteria can concentrate Cu2+ over 85-fold and Ni2+ at least tenfold from the growth medium [27].
Hydrogenases catalyze the reversible oxidation of molecular hydrogen, and are of two types in bacteria: NiFe and Fe-only H2ase [5]. The NiFe-H2ase is typically involved in oxidation of hydrogen; whereas Fe-only H2ase catalyzes the reduction of protons as terminal electron acceptors to yield hydrogen [5]. Hydrogenase plays a key role in the metabolism of acetogens, most of which can grow autotrophically, as well as heterotrophically [9]. During autotrophic growth on H2 and CO2, H2ase-mediated oxidation of H2 provides reducing equivalents for fixation of CO2 into acetate and cell biomass [9]. In contrast, H2ase in acetogens growing on carbohydrates dispose off the excess reducing equivalents by reducing protons into H2 [1]. In addition, existence of multiple H2ases that account for different roles under different growth conditions have also been reported in acetogens [9]. H2ase of C. pasteurianum, a Fe-only H2ase has been most extensively studied both biochemically and spectroscopically [22]. H2ase purified from fructose grown cells of Acetobacterium woodii also does not contain nickel, and exhibits spectroscopic properties similar to that of Fe-only H2ase of C. pasteurianum [22, 25]. To our knowledge, all purification and spectroscopic studies of H2ase in acetogens have been done from heterotrophically grown acetogens, which has decreased the probability of isolating Fe–Ni hydrogenase, and have ruled out the presence of nickel in the enzyme. However, a genome sequence analysis of M. thermoacetica has revealed a gene cluster homologue of genes that make up an operon required for maturation of the Ni–Fe H2ase [23]. In the present study, H2ase activity was stimulated by increased concentrations of Ni2+, and reduced by the elimination of Fe2+ from the media. Thus, H2ases present in CO:CO2-grown C. ragsdalei may be Fe-only and/or NiFe H2ases. Since, CO:CO2 was used as growth substrate in present study, low H2ase activities obtained were probably due to inhibition of H2ase by CO [25].
In solventogenic clostridia, ADH catalyzes the reduction of acetyl CoA to ethanol [6]. The biochemical and genetic studies on ADH from different clostridia [6, 13] have indicated the role of ADH in solvent production. Clostridial ADHs have been shown to contain zinc [15] and iron [26]. A reduction in ADH activity of C. ragsdalei was observed when iron was removed from the media, suggesting that ADH of C. ragsdalei may be an iron-containing enzyme. Furthermore, supplementing the growth medium of C. ragsdalei with optimized levels of Zn2+ resulted in increased ethanol production and improved cell growth; however, no changes in enzyme activities (CODH, FDH, H2ase, and ADH) were observed upon varying Zn2+ concentration. These results indicate that both types of ADH may be present in C. ragsdalei.
The effects of trace metals on growth, homoacetogenesis, and activities of enzymes of the acetyl-CoA pathway (CODH, FDH, and H2ase) [8, 14] have been mostly studied in heterotrophically grown acetogens. However, the effects of trace metals on product formation under chemolithotrophic growth conditions have not been measured. The activities of key enzymes of Wood-Ljungdahl pathway (CODH, FDH, and H2ase) may vary under heterotrophic and chemolithotrophic growth conditions [7, 18] with higher enzyme activities obtained when cultures were grown on CO:CO2 and/or H2:CO2 than when grown on sugars [7, 18]. In the present study, the effects of trace metals on growth, enzyme activities, and ethanol production by an acetogen (C. ragsdalei) grown on CO:CO2 was studied, which can provide further insights about medium manipulation for increased ethanol production by solventogenic acetogens via syngas fermentation.
Conclusions
Bioethanol production from fermentation of lignocellulosic biomass-derived syngas is a promising alternative for biofuel production. This process offers advantages such as the utilization of whole biomass, including lignin, irrespective of the biomass source and the elimination of the extensive pretreatment steps to obtain fermentable sugars. One of the major limitations of syngas fermentation is the low ethanol yield of biocatalysts. In the present study, ethanol production by C. ragsdalei was improved four-fold by optimizing the trace metal concentrations in the growth medium, emphasizing the importance of medium optimization for improving the ethanol fermentation from syngas. The increase in ethanol production by C. ragsdalei was also substantiated by increased cell growth and metalloenzyme activities of the acetyl-CoA pathway. The presence of Cu2+ in the medium negatively affected the ethanol production by C. ragsdalei. The optimum medium concentrations of Cu2+, Ni2+, Zn2+, SeO4 −, and WO4 − for ethanol production were 0, 8.5, 35, 7, and 5 μM, respectively. Evaluation of other media components (minerals and vitamins) is warranted for further improvement of the growth and ethanol production by C. ragsdalei from syngas.
Acknowledgments
This research was supported in part by USDA-CSREES Special Grant awards 2005-34447-15711 and 2006-34447-16939. We would like to thank Dr. James A. Zahn for his constructive suggestions in this manuscript.
References
Huhnke R, Lewis R, Tanner RS (2008) Isolation and characterization of novel clostridial species. US patent application. Publication no. US 2008/0057554 A1
Mehta MD, Saxena J, Tanner RS (2004) Enzyme activities in clostridia producing ethanol from carbon monoxide. Abstr 104th Annu Meet Am Soc Microbiol, O-81, p 474
Peckman KR (1976) Investigation of the phylogenetic relationship of Sporomusa ureae to members of the Bacillaceae using primary structural characterization of 16S ribosomal ribonucleic acids. PhD thesis. University of Illinois, Urbana
Perlack RD, Wright LL, Turhollow AF, Graham RL, Stokes BJ, Erbach DC (2005) Biomass as feedstock for a bioenergy and bioproducts industry: the technical feasibility of a billion-ton annual supply. DOE/GO-102005-2135, Oak Ridge National Laboratory, Oak Ridge. http://:www.osti.gov/bridge