-
PDF
- Split View
-
Views
-
Cite
Cite
Mohammad Shafiei, Abed-Ali Ziaee, Mohammad Ali Amoozegar, Purification and characterization of an organic-solvent-tolerant halophilic α-amylase from the moderately halophilic Nesterenkonia sp. strain F, Journal of Industrial Microbiology and Biotechnology, Volume 38, Issue 2, 1 February 2011, Pages 275–281, https://doi.org/10.1007/s10295-010-0770-1
- Share Icon Share
Abstract
A halophilic α-amylase produced by Nesterenkonia sp. strain F was purified to homogeneity by 80% ethanol precipitation, Q-Sepharose anion exchange, and Sephacryl S-200 gel filtration chromatography. The purified amylase exhibited specific activity of 357 unit/mg protein that corresponds to twofold purification. The molecular mass of the amylase was determined to be 57 kDa by sodium dodecyl sulfate polyacrylamide gel electrophoresis (SDS-PAGE) and gel filtration chromatography. The optimal pH and temperature for enzyme activity were 6.5 and 45°C, respectively. The amylase was active over a wide range of salt concentrations (0–4 M) with maximum activity at 0.75–1 M NaCl. The α-amylase activity was stimulated by Ca2+ and inhibited by ethylenediamine tetraacetic acid (EDTA), suggesting that this enzyme is a metalloenzyme. The purified enzyme showed remarkable stability towards surfactants (Tween 20, Tween 80, and Triton X-100), and its activity was increased by β-mercaptoethanol. The halophilic α-amylase was stable in the presence of various organic solvents such as benzene, chloroform, toluene, and cyclohexane. These properties indicate wide potential applications of this α-amylase in starch-processing industries.
Introduction
Halophiles are extremophilic microorganisms that live, grow, and multiply in highly saline environments, and can be a source of halophilic enzymes [20]. Halophilic enzymes show optimal activity at high salt concentrations and are of interest in many harsh industrial processes where concentrated salt solutions would inhibit many enzymatic conversions [35]. In contrast to enzymes from extremely halophilic bacteria, which are active only at extremely high salt concentrations [18], exoenzymes from moderately halophilic bacteria can be active over a wide range of salt concentrations even in the absence of salt and therefore have potential for use in various biotechnological processes [36].
Amylases are among the most important enzymes and are of great significance in present-day biotechnology [13]. This class of industrial enzymes plays an important role in starch degradation and at present constitutes about 25–33% of the world market for the industrial enzymes [31]. Amylases have a wide spectrum of applications in many fields such as starch saccharification, textile, food, baking, brewing, and distilling industries [26]. Because of the industrial importance of amylase, there is ongoing interest in the isolation of new amylases suitable for new industrial applications [3]. In this respect, halophilic amylases have been isolated from various halophilic bacteria and characterized [28].
The ability of enzymes to remain active in the presence of organic solvents has received a great deal of attention over the past two decades [30]. There are numerous advantages of using enzymes in organic solvents or aqueous solutions containing organic solvents compared with in water, such as increased solubility of nonpolar substrates and elimination of microbial contamination in the reaction mixture [22]. In general, enzymes are easily denatured and their catalytic activities disappear in the presence of organic solvents, even if they are almost water insoluble [23]. Nevertheless, enzymes that naturally remain stable in the presence of organic solvents without the need for special stabilization could be very useful for biotechnological applications in which such solvents are used [24]. Organic-solvent-tolerant enzymes have been purified and characterized from various microorganisms [9, 34]. Because salt has the effect of reducing water activity, halophilic enzymes are thought to be important biocatalysts in other low-water-activity environments, such as in aqueous/organic and nonaqueous media [21]. Among halophilic enzymes, a halophilic protease with organic-solvent-tolerant properties has been purified from Salinivibrio sp. strain AF-2004 [15]. Also, Fukushima et al. [10] reported an organic-solvent-tolerant halophilic α-amylase from the extremely halophilic archaea, Haloarcula sp. strain S-1. However, to the best of our knowledge, there are no reports on organic-solvent-tolerant α-amylases from moderately halophilic species. Previously, we purified and characterized a halophilic α-amylase with raw-starch-digesting activity from a moderately halophilic bacterium, Nesterenkonia sp. strain F [32]. In the present study we report the purification and characterization of another halophilic α-amylase from this strain, including organic solvent tolerance of the enzyme.
Materials and methods
Bacterial strain and culture conditions
The moderately halophilic bacterium Nesterenkonia sp. strain F that produced extracellular amylases was isolated from Aran-Bidgol Lake in the center of Iran. For maximum amylase production, preculture was prepared by inoculating 0.5 ml of the strain suspension containing 1.5 × 108 cfu/ml in 20 ml culture medium consisted of the following (w/v): 1% peptone, 0.5% yeast extract, 1% soluble starch, and 7.5% NaCl (pH 7.0). The preculture was incubated at 33°C on a rotary shaker at 220 rpm for 24 h. Erlenmeyer flasks (500 ml), containing 100 ml culture medium were inoculated with 1 ml preculture and incubated for 72 h at the same conditions as preculture medium. Cell-free supernatant containing the extracellular amylases was harvested by centrifugation at 25,000×g for 80 min at 4°C and used for amylase purification.
Purification of the amylase
All purification procedures were carried out at 4°C. The enzyme was precipitated by bringing the supernatant to 80% (v/v) saturation with ethanol and stirred for 2 h. The precipitate was centrifuged at 12,000×g for 20 min and dissolved in a minimum volume of 20 mM Tris–HCl (pH 7.5) containing 10 mM CaCl2 and 0.2 M NaCl (buffer A). The concentrated enzyme was dialyzed against the same buffer overnight. This solution was applied to a column (1.6 cm × 14 cm) of Q-Sepharose HP previously equilibrated with buffer A. After the column was washed with the same buffer, bound proteins were eluted with a stepwise gradient of NaCl concentrations (0.2, 0.25, 0.3, 0.35, and 0.4 M) in buffer A at flow rate of 1 ml/min. Fractions exhibiting amylase activity were pooled and concentrated by freeze-drying. The resulting concentrate was dissolved in a minimal volume of 20 mM Tris–HCl (pH 7.5) containing 0.3 M NaCl and 10 mM CaCl2 (buffer B), and dialyzed against the same buffer for 24 h. The dialyzed sample was loaded onto a Sephacryl S-200 gel filtration column (1.6 cm × 60 cm), previously equilibrated with buffer B. Fractions of 2 ml were collected at flow rate of 0.33 ml/min. Fractions containing amylase were pooled and concentrated by ultrafiltration (Centricon, Amicon, USA). The purified enzyme was used for further analysis. The molecular mass of the purified enzyme was determined using the same column, which was calibrated previously with alcohol dehydrogenase (150 kDa), bovine serum albumin (BSA) (67 kDa), bovine carbonic anhydrase (29 kDa), and cytochrome C (12.4 kDa). Blue Dextran was used to determine the void volume of the column.
Amylase assay
Amylase activity was assayed using the previously described method [32]. In brief, 50 μl of suitably diluted enzyme solution was added to 450 μl 1% (w/v) soluble starch in 20 mM Tris–HCl (pH 6.5) containing 0.75 M NaCl (buffer C) and incubated at 45°C for 30 min. The reaction was stopped by addition of dinitrosalicylic acid (DNS) solution. After appropriate dilution, the reducing sugar in the dilute was determined by using the DNS method [1]. One unit of amylase activity was defined as the amount of enzyme that produced 1 μmol reducing sugar as maltose per minute under the assay conditions. Protein concentrations were estimated according to the method of Bradford [2].
Polyacrylamide gel electrophoresis
Purity and molecular mass of the amylase were analyzed by sodium dodecyl sulfate polyacrylamide gel electrophoresis (SDS–PAGE) using 12% acrylamide, as described by Laemmli [19]. Samples were heated at 100°C for 5 min before electrophoresis. After completion of electrophoresis, the gel was stained with Coomassie Brilliant Blue R-250. A ready-to-use molecular size marker (Fermentas; SM#0431) was used.
Effect of pH, temperature, and NaCl
The effect of pH on amylase activity was measured in the pH range of 4 to 10, using the appropriate buffers at concentration of 100 mM (4.0–5.5, sodium acetate; 6.0–7.5, sodium phosphate; 8.0–9.0, Tris–HCl; 9.5–12.0, glycine–NaOH) under standard assay conditions. The optimum temperature for enzyme activity was determined by conducting the assay at various temperatures ranging from 15°C to 70°C. To determine the effect of NaCl concentrations on enzyme activity, the enzyme assay was performed in the presence of 0–4 M NaCl.
Effect of different metal ions and chemical reagents
The effect of different metal ions on amylase activity was studied by pre-incubating the enzyme with 10 mM of various metal ions at 25°C for 30 min. Then, the remaining activity was determined using the standard enzyme assay. Activity in the absence of metal ions was taken as 100%.
To determine the influence of EDTA, phenylmethylsulfonyl fluoride (PMSF), β-mercaptoethanol (10 mM), and various concentrations of some surfactants (Triton X-100, Tween 80, Tween 20, SDS, and sarcosyl), the same procedure was used. Activity in the absence of any additives was taken as 100%.
Substrate specificity
To determine the substrate specificity of the amylase, the enzyme assay was performed in reaction mixture containing 1% each of soluble starch, glycogen, amylose, and pullulan in buffer C for 30 min at 45°C.
Organic solvent stability of the amylase
To examine stability of the amylase to different organic solvents, 1.0 volume of organic solvent was added to 4.0 volumes of the amylase solution and incubated at 25°C with constant shaking at 150 rpm for 7 days. After incubation for appropriate periods of time, aliquots were withdrawn and remaining activity was measured under enzyme assay conditions. The effect of higher concentration of solvents on amylase stability [50% and 80% (v/v)] was also studied in the same manner.
Results
Purification and properties of the amylase
Summary of purification steps of the amylase from Nesterenkonia sp. strain F
Purification steps . | Total activity (units) . | Total protein (mg) . | Specific activity (units/mg) . | Purification (fold) . | Yield (%) . |
---|---|---|---|---|---|
Crude enzyme | 1010 | 60.3 | 16.7 | 1 | 100 |
80% Ethanol | 595 | 11.8 | 50.4 | 3 | 59 |
Q-Sepharose | 81 | 0.84 | 96.4 | 5.77 | 8 |
Sephacryl S-200 | 20 | 0.056 | 357 | 21.4 | 2 |
Purification steps . | Total activity (units) . | Total protein (mg) . | Specific activity (units/mg) . | Purification (fold) . | Yield (%) . |
---|---|---|---|---|---|
Crude enzyme | 1010 | 60.3 | 16.7 | 1 | 100 |
80% Ethanol | 595 | 11.8 | 50.4 | 3 | 59 |
Q-Sepharose | 81 | 0.84 | 96.4 | 5.77 | 8 |
Sephacryl S-200 | 20 | 0.056 | 357 | 21.4 | 2 |
Summary of purification steps of the amylase from Nesterenkonia sp. strain F
Purification steps . | Total activity (units) . | Total protein (mg) . | Specific activity (units/mg) . | Purification (fold) . | Yield (%) . |
---|---|---|---|---|---|
Crude enzyme | 1010 | 60.3 | 16.7 | 1 | 100 |
80% Ethanol | 595 | 11.8 | 50.4 | 3 | 59 |
Q-Sepharose | 81 | 0.84 | 96.4 | 5.77 | 8 |
Sephacryl S-200 | 20 | 0.056 | 357 | 21.4 | 2 |
Purification steps . | Total activity (units) . | Total protein (mg) . | Specific activity (units/mg) . | Purification (fold) . | Yield (%) . |
---|---|---|---|---|---|
Crude enzyme | 1010 | 60.3 | 16.7 | 1 | 100 |
80% Ethanol | 595 | 11.8 | 50.4 | 3 | 59 |
Q-Sepharose | 81 | 0.84 | 96.4 | 5.77 | 8 |
Sephacryl S-200 | 20 | 0.056 | 357 | 21.4 | 2 |
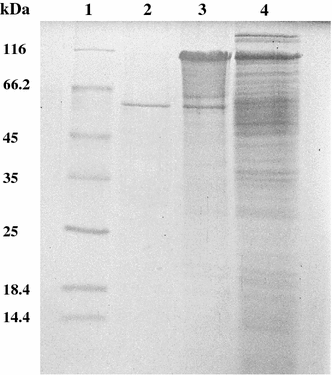
SDS–PAGE analysis of different steps of amylase purification. Lane 1 molecular mass markers, lane 2 Sephacryl S-200 purified amylase, lane 3 Q-Sepharose column, lane 4 ethanol-precipitated enzyme
The kinetic parameters K m and V max were determined by performing the enzyme assay in the presence of various concentration of soluble starch as substrate. The values of K m and V max were calculated to be 5.8 mg/ml and 1.07 mg/ml/min, respectively.
Substrate specificity analysis of the amylase indicated that soluble starch was hydrolyzed at a higher rate than glycogen and amylose. No activity against pullulan was detected (Table 2).
Substrate specificity of the amylase
Substrate [1% (w/v)] . | Relative activity (%) . |
---|---|
Starch | 100 ± 1.7 |
Glycogen | 39.2 ± 1.2 |
Amylose | 17 ± 2.0 |
Pullulan | 0 |
Substrate [1% (w/v)] . | Relative activity (%) . |
---|---|
Starch | 100 ± 1.7 |
Glycogen | 39.2 ± 1.2 |
Amylose | 17 ± 2.0 |
Pullulan | 0 |
Mean ± standard deviation (SD) values for three independent experiments are shown
Substrate specificity of the amylase
Substrate [1% (w/v)] . | Relative activity (%) . |
---|---|
Starch | 100 ± 1.7 |
Glycogen | 39.2 ± 1.2 |
Amylose | 17 ± 2.0 |
Pullulan | 0 |
Substrate [1% (w/v)] . | Relative activity (%) . |
---|---|
Starch | 100 ± 1.7 |
Glycogen | 39.2 ± 1.2 |
Amylose | 17 ± 2.0 |
Pullulan | 0 |
Mean ± standard deviation (SD) values for three independent experiments are shown
Effect of pH, temperature, and NaCl concentration on the activity of the amylase
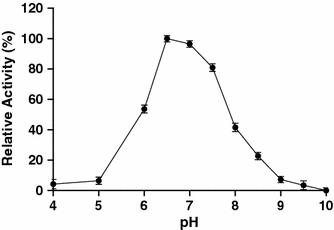
Effect of pH on the activity of the amylase. Relative activity was defined as the percentage of maximum activity detected in the assay. Values are mean ± SD of three independent experiments
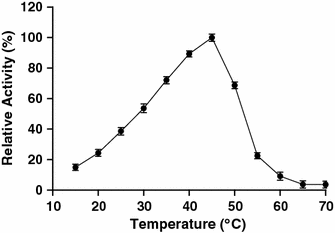
Effect of temperature on the activity of the amylase. Relative activity was defined as the percentage of maximum activity detected in the assay. Values are mean ± SD of three independent experiments
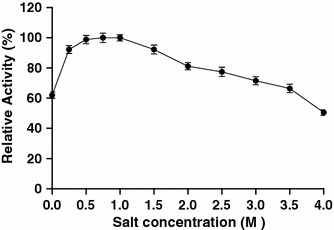
Effect of NaCl concentrations on the amylase activity. Activity was measured in the reaction mixture containing various concentrations of NaCl. Relative activity was defined as the percentage of maximum activity detected in the assay. Values are mean ± SD of three independent experiments
Effect of metal ions and chemical reagents on the amylolytic activity
The effect of various metal ions on the amylolytic activity of the purified enzyme was tested (Table 3). Metal ions had different effects on the enzyme activity. While Rb+, Li+, Cs+, and Mg2+ had no significant effect, complete inhibition by Fe3+, Cu2+, and Zn2 was observed. Also, the amylase activity was inhibited by Mn2+, Ni2+, and Al3+ (25.5%, 50%, and 84.5% inhibition, respectively). However, Ca2+ stimulated amylase activity by 62%. The presence of the chelating agent EDTA inhibited enzyme activity by 53%. PMSF had no significant effect on enzyme activity, whereas β-mercaptoethanol was found to enhance activity (to 136% of original activity).
Effect of various metal ions on amylase activity
Metal ion (10 mM) . | Residual activity (%) . |
---|---|
CaCl2 | 163 ± 2.7 |
MgCl2 | 95 ± 1.8 |
LiCl | 100 ± 0.9 |
CsCl | 100 ± 1.2 |
RbCl | 98.4 ± 1.9 |
Ni(NO3)2 | 50 ± 2.3 |
CuSO4 | 6.8 ± 2.0 |
AlCl3 | 15.5 ± 1.7 |
Fe2(SO4)3 | 4 ± 1.4 |
MnSO4 | 74.5 ± 2.2 |
ZnSO4 | 6 ± 1.5 |
Metal ion (10 mM) . | Residual activity (%) . |
---|---|
CaCl2 | 163 ± 2.7 |
MgCl2 | 95 ± 1.8 |
LiCl | 100 ± 0.9 |
CsCl | 100 ± 1.2 |
RbCl | 98.4 ± 1.9 |
Ni(NO3)2 | 50 ± 2.3 |
CuSO4 | 6.8 ± 2.0 |
AlCl3 | 15.5 ± 1.7 |
Fe2(SO4)3 | 4 ± 1.4 |
MnSO4 | 74.5 ± 2.2 |
ZnSO4 | 6 ± 1.5 |
Enzyme was dialyzed against 20 mM Tris–HCl buffer (pH 6.5). Samples were pre-incubated with various metal ions (10 mM) for 30 min at 25°C, and the remaining activity was measured under standard assay. Activity in the absence of any additives was taken as 100%. Mean ± SD values for three independent experiments are shown
Effect of various metal ions on amylase activity
Metal ion (10 mM) . | Residual activity (%) . |
---|---|
CaCl2 | 163 ± 2.7 |
MgCl2 | 95 ± 1.8 |
LiCl | 100 ± 0.9 |
CsCl | 100 ± 1.2 |
RbCl | 98.4 ± 1.9 |
Ni(NO3)2 | 50 ± 2.3 |
CuSO4 | 6.8 ± 2.0 |
AlCl3 | 15.5 ± 1.7 |
Fe2(SO4)3 | 4 ± 1.4 |
MnSO4 | 74.5 ± 2.2 |
ZnSO4 | 6 ± 1.5 |
Metal ion (10 mM) . | Residual activity (%) . |
---|---|
CaCl2 | 163 ± 2.7 |
MgCl2 | 95 ± 1.8 |
LiCl | 100 ± 0.9 |
CsCl | 100 ± 1.2 |
RbCl | 98.4 ± 1.9 |
Ni(NO3)2 | 50 ± 2.3 |
CuSO4 | 6.8 ± 2.0 |
AlCl3 | 15.5 ± 1.7 |
Fe2(SO4)3 | 4 ± 1.4 |
MnSO4 | 74.5 ± 2.2 |
ZnSO4 | 6 ± 1.5 |
Enzyme was dialyzed against 20 mM Tris–HCl buffer (pH 6.5). Samples were pre-incubated with various metal ions (10 mM) for 30 min at 25°C, and the remaining activity was measured under standard assay. Activity in the absence of any additives was taken as 100%. Mean ± SD values for three independent experiments are shown
Amylase stability was also studied in the presence of some surfactants. The enzyme was highly stable in the presence of nonionic surfactants, retaining full activity in the presence of 3% each of Triton X-100, Tween 80, and Tween 20. However, the enzyme lost 48% and 29% activity in the presence of 0.5% SDS and sarcosyl, respectively.
Effect of organic solvents on the amylase stability
The effects of various organic solvents on the stability of the amylase were studied. Mixtures of enzyme solution and organic solvents were incubated at 25°C with shaking at 150 rpm, and remaining activity was determined at appropriate time intervals over a period of 7 days. The half-lives of enzyme activity in the presence of various organic solvents are summarized in Table 4. In the absence of organic solvent, the half-life of the amylase was about 54 days. The half-lives of the enzyme in the presence of 20% ethanol, acetone, and cyclohexanol were 52, 51, and 28 days, respectively. In the case of 20% 1-butanol, the half-life was 7.5 days. However, in the presence of 20% organic solvents with log P ow ≥1.97, the half-life was longer than 79 days. In particular, no inactivation of the enzyme was observed in the presence of 20% benzene, chloroform, toluene, cyclohexane, and 1-decanol. At higher concentrations of the solvents (50% and 80%), the half-life of the amylase was drastically decreased in the presence of water-soluble organic solvents with log P ow <1.97, but in the presence of organic solvents with higher log P ow, the half-lives were higher than in the absence of the solvents (Table 4).
Half-lives of the amylase from Nesterenkonia sp. strain F in the presence of various organic solvents
Organic solvent . | Log P ow . | Half-life (days) . | ||
---|---|---|---|---|
20% . | 50% . | 80% . | ||
None | − | 54 ± 1.5 | 54 ± 1.5 | 54 ± 1.5 |
Ethanol | −0.3 | 52 ± 1.7 | 5.5 ± 0.2 | 3.9 ± 0.12 |
Acetone | −0.24 | 51 ± 1.4 | 2.5 ± 0.08 | 0.17 ± 0.006 |
1-Butanol | 0.88 | 7.5 ± 0.25 | 2 ± 0.06 | 0.083 ± 0.002 |
Cyclohexanol | 1.23 | 28 ± 1.0 | 2.9 ± 0.1 | 0.208 ± 0.005 |
Chloroform | 1.97 | >100 | >100 | >100 |
Benzene | 2.13 | >100 | >100 | >100 |
Toluene | 2.73 | >100 | >100 | >100 |
1-Octanol | 2.97 | 79 ± 3.0 | 66 ± 2.1 | 60 ± 1.7 |
Cyclohexane | 3.3 | >100 | >100 | >100 |
1-Decanol | 4.1 | >100 | >100 | 71.5 ± 2.4 |
Organic solvent . | Log P ow . | Half-life (days) . | ||
---|---|---|---|---|
20% . | 50% . | 80% . | ||
None | − | 54 ± 1.5 | 54 ± 1.5 | 54 ± 1.5 |
Ethanol | −0.3 | 52 ± 1.7 | 5.5 ± 0.2 | 3.9 ± 0.12 |
Acetone | −0.24 | 51 ± 1.4 | 2.5 ± 0.08 | 0.17 ± 0.006 |
1-Butanol | 0.88 | 7.5 ± 0.25 | 2 ± 0.06 | 0.083 ± 0.002 |
Cyclohexanol | 1.23 | 28 ± 1.0 | 2.9 ± 0.1 | 0.208 ± 0.005 |
Chloroform | 1.97 | >100 | >100 | >100 |
Benzene | 2.13 | >100 | >100 | >100 |
Toluene | 2.73 | >100 | >100 | >100 |
1-Octanol | 2.97 | 79 ± 3.0 | 66 ± 2.1 | 60 ± 1.7 |
Cyclohexane | 3.3 | >100 | >100 | >100 |
1-Decanol | 4.1 | >100 | >100 | 71.5 ± 2.4 |
Enzyme solution was incubated at 25°C with shaking at 150 rpm in presence or absence of different concentrations of organic solvents for 7 days. Residual activity was measured after 0, 0.04, 0.75, 1, 2, 4, and 7 days. Half-life was calculated from the exponential regression curve. Mean ± SD for three independent experiments are shown
Half-lives of the amylase from Nesterenkonia sp. strain F in the presence of various organic solvents
Organic solvent . | Log P ow . | Half-life (days) . | ||
---|---|---|---|---|
20% . | 50% . | 80% . | ||
None | − | 54 ± 1.5 | 54 ± 1.5 | 54 ± 1.5 |
Ethanol | −0.3 | 52 ± 1.7 | 5.5 ± 0.2 | 3.9 ± 0.12 |
Acetone | −0.24 | 51 ± 1.4 | 2.5 ± 0.08 | 0.17 ± 0.006 |
1-Butanol | 0.88 | 7.5 ± 0.25 | 2 ± 0.06 | 0.083 ± 0.002 |
Cyclohexanol | 1.23 | 28 ± 1.0 | 2.9 ± 0.1 | 0.208 ± 0.005 |
Chloroform | 1.97 | >100 | >100 | >100 |
Benzene | 2.13 | >100 | >100 | >100 |
Toluene | 2.73 | >100 | >100 | >100 |
1-Octanol | 2.97 | 79 ± 3.0 | 66 ± 2.1 | 60 ± 1.7 |
Cyclohexane | 3.3 | >100 | >100 | >100 |
1-Decanol | 4.1 | >100 | >100 | 71.5 ± 2.4 |
Organic solvent . | Log P ow . | Half-life (days) . | ||
---|---|---|---|---|
20% . | 50% . | 80% . | ||
None | − | 54 ± 1.5 | 54 ± 1.5 | 54 ± 1.5 |
Ethanol | −0.3 | 52 ± 1.7 | 5.5 ± 0.2 | 3.9 ± 0.12 |
Acetone | −0.24 | 51 ± 1.4 | 2.5 ± 0.08 | 0.17 ± 0.006 |
1-Butanol | 0.88 | 7.5 ± 0.25 | 2 ± 0.06 | 0.083 ± 0.002 |
Cyclohexanol | 1.23 | 28 ± 1.0 | 2.9 ± 0.1 | 0.208 ± 0.005 |
Chloroform | 1.97 | >100 | >100 | >100 |
Benzene | 2.13 | >100 | >100 | >100 |
Toluene | 2.73 | >100 | >100 | >100 |
1-Octanol | 2.97 | 79 ± 3.0 | 66 ± 2.1 | 60 ± 1.7 |
Cyclohexane | 3.3 | >100 | >100 | >100 |
1-Decanol | 4.1 | >100 | >100 | 71.5 ± 2.4 |
Enzyme solution was incubated at 25°C with shaking at 150 rpm in presence or absence of different concentrations of organic solvents for 7 days. Residual activity was measured after 0, 0.04, 0.75, 1, 2, 4, and 7 days. Half-life was calculated from the exponential regression curve. Mean ± SD for three independent experiments are shown
Discussion
Being intrinsically stable and active at high salt concentrations, halophilic enzymes have important potential biotechnological applications. A considerable amount of effort has been dedicated to study of extracellular salt-tolerant enzymes from moderately halophilic bacteria, especially towards use of such enzymes in biotechnological processes [36]. In this paper we described an organic-solvent-tolerant halophilic α-amylase from a moderate halophilic bacterium, Nesterenkonia sp. strain F.
Like many amylolytic enzymes, the amylase from strain F is produced during the exponential growth phase and reached maximum level during the stationary phase (data not shown). According to SDS–PAGE analysis, the amylase is a single polypeptide chain of about 57 kDa. This molecular weight is close to the estimated molecular weight of halophilic α-amylases from Micrococcus varians subsp. halophilus (60 kDa) [17], Haloferax mediterranei (58 kDa) [27], and Chromohalobacter sp. TVSP 101 (62 kDa) [28]. However, the molecular weight of the amylase is lower than that of another α-amylase from strain F (100 kDa). Studies to determine biochemical properties of the enzyme revealed that, similar to another strain F α-amylase, the amylase showed an optimum temperature for activity of 45°C, with loss of activity above 55°C. This property might limit its use in industrial applications that require high temperatures, but favors its application in processes that require complete inactivation of the enzyme with increasing temperature in the process, i.e., in the baking industry [7]. The optimal pH for the amylase (pH 6.5) was similar to that reported for halophilic amylases from various microorganisms [8, 14, 25]. The amylase is highly active over a board range of salt concentrations (0–4 M). This type of extreme halotolerance has been observed in halophilic amylases from Nesterenkonia halobia [25], Halomonas meridiana [7], Bacillus dipsosauri [8], Chromohalobacter sp. TVSP 101 [28], and Halobacterium salinarum [11]. Amylases with this characteristic may have interesting applications in treatment of saline waters or waste solutions with starch residues and high salt content.
Enzyme inhibition studies showed that the amylase activity was stimulated by Ca2+ ion and inhibited by EDTA, indicating that the amylase is a metalloenzyme [33]. In contrast to another α-amylase from strain F [32], the amylase described herein was stimulated by β-mercaptoethanol. This result suggests that disulfide bonds are not essential for its enzyme activity and could play a role in regulating molecule flexibility to preserve enzyme activity [4]. Also, reduction of disulfide bonds in the amylase may lead to changes in the amylase molecule conformation, allowing the active site and the substrate to interact easily [6]. A similar finding has not been previously reported for other halophilic α-amylases. The effect of various surfactants on the enzyme revealed that, although the amylase was inhibited by 0.5% anionic surfactants (SDS and sarcosyl), it was stable in the presence of nonionic surfactants at higher concentrations than those reported for other halophilic α-amylases [5, 16, 32]. To determine the type of the amylase, substrate specificity tests were carried out; the results suggest that this amylase is able to hydrolyze 1–4 bounds but not 1–6 bounds. Also, the amylase released maltotriose and maltotetraose as the main end products from soluble starch, indicating that it is an α-amylase (data not shown) [26].
Organic-solvent-tolerant halophilic enzymes appear to be quite attractive for industrial applications such as bioremediation of carbohydrate-polluted salt marshes and industrial wastewaters contaminated with organic solvents. However, halophilic α-amylase with organic solvent tolerance has been reported only from an extremely halophilic archaea, Haloarcula sp. strain S-1 [10]. For these reasons, the behavior of the halophilic α-amylase from strain F in the presence of some organic solvents was tested. As shown in Table 4, the half-lives of the α-amylase in the presence of 20% of all organic solvents tested, with the exception of 1-butanol and cyclohexanol, were similar to or longer than in the absence of these solvents. Thus, the α-amylase was stable in the presence of 20% of these organic solvents. Also, the effect of higher concentrations of the solvents revealed the stability of the amylase in the presence of high concentration (50% and 80% v/v) of organic solvents with log P ow ≥1.97. Together these results indicated that, in contrast to the organic solvent stability of some proteases [12, 15] and lipases [24, 29], which had no relationship with the polarity (log P ow value) of the added organic solvents, the amylase stability was dependent on the polarity of the solvent and decreased only in the presence of high concentration of solvent with log P ow values <1.97. The α-amylase from extremely halophilic archaea, Haloarcula sp. strain S-1, retained 70% of its activity after 1 h of incubation in the presence of water-insoluble organic solvents and chloroform, and was completely inhibited by water-soluble organic solvents. Also, the strain S-1 α-amylase was active only in the presence of high concentration of salts (3 M or higher) [10]. To the best of our knowledge, this is the first report on an organic-solvent-tolerant halophilic α-amylase from a moderately halophilic bacterium.
In conclusion, the enzyme described herein was stable in the presence of some surfactants and may have applications in detergent industries. Also, considering its high activity over a wide range of NaCl concentration, even without NaCl, and stability in the presence of organic solvents, the Nesterenkonia sp. strain F α-amylase can be used in biotechnological processes where high salt concentrations and organic solvents are present.
Acknowledgments
Financial support for this work was provided by the Research Council, University of Tehran.