-
PDF
- Split View
-
Views
-
Cite
Cite
Ana Joyce Muñoz, Georgina Hernández-Chávez, Ramon de Anda, Alfredo Martínez, Francisco Bolívar, Guillermo Gosset, Metabolic engineering of Escherichia coli for improving l-3,4-dihydroxyphenylalanine (l-DOPA) synthesis from glucose, Journal of Industrial Microbiology and Biotechnology, Volume 38, Issue 11, 1 November 2011, Page 1845, https://doi.org/10.1007/s10295-011-0973-0
- Share Icon Share
Abstract
l-3,4-dihydroxyphenylalanine (l-DOPA) is an aromatic compound employed for the treatment of Parkinson's disease. Metabolic engineering was applied to generate Escherichia coli strains for the production of l-DOPA from glucose by modifying the phosphoenolpyruvate:sugar phosphotransferase system (PTS) and aromatic biosynthetic pathways. Carbon flow was directed to the biosynthesis of l-tyrosine (l-Tyr), an l-DOPA precursor, by transforming strains with compatible plasmids carrying genes encoding a feedback-inhibition resistant version of 3-deoxy-d-arabino-heptulosonate-7-phosphate synthase, transketolase, the chorismate mutase domain from chorismate mutase-prephenate dehydratase from E. coli and cyclohexadienyl dehydrogenase from Zymomonas mobilis. The effects on l-Tyr production of PTS inactivation (PTS− gluc+ phenotype), as well as inactivation of the regulatory protein TyrR, were evaluated. PTS inactivation caused a threefold increase in the specific rate of l-Tyr production (ql-Tyr), whereas inactivation of TyrR caused 1.7- and 1.9-fold increases in ql-Tyr in the PTS+ and the PTS− gluc+ strains, respectively. An 8.6-fold increase in l-Tyr yield from glucose was observed in the PTS− gluc+ tyrR − strain. Expression of hpaBC genes encoding the enzyme 4-hydroxyphenylacetate 3-hydroxylase from E. coli W in the strains modified for l-Tyr production caused the synthesis of l-DOPA. One of such strains, having the PTS− gluc+ tyrR − phenotype, displayed the best production parameters in minimal medium, with a specific rate of l-DOPA production of 13.6 mg/g/h, l-DOPA yield from glucose of 51.7 mg/g and a final l-DOPA titer of 320 mg/l. In a batch fermentor culture in rich medium this strain produced 1.51 g/l of l-DOPA in 50 h.
Introduction
Bacteria and plants have the metabolic capacity for synthesizing a large number of aromatic compounds. This class of chemicals includes products employed as pharmaceuticals, food additives, polymer precursors, among other applications. l-3,4-dihydroxyphenylalanine (l-DOPA) is an aromatic compound having pharmaceutical applications, mainly, the treatment of Parkinson's disease. l-DOPA can be produced employing chemical, enzymatic, or microbial processes [15, 17, 26]. Biotechnological production of l-DOPA can be based on a reaction catalyzed by tyrosine phenol-lyase employing catechol, pyruvate, and ammonia as substrates [13]. Other methods for producing this compound involve hydroxylation of the amino acid l-tyrosine (l-Tyr) by the enzyme p-hydroxyphenylacetate 3-hydroxylase or tyrosinase [15, 16, 24]. Such processes are performed with whole cell or purified enzyme catalysts. An E. coli strain with the capacity to synthesize l-DOPA from glucose was developed by performing genetic modifications that redirect carbon flow from central metabolism to the l-Tyr biosynthetic pathway and expressing the genes encoding the enzyme p-hydroxyphenylacetate 3-hydroxylase [14]. This development opens the possibility of applying metabolic engineering strategies to increase l-DOPA production in E. coli.
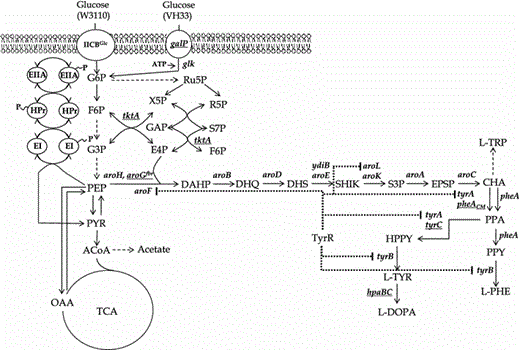
Pathways related to the biosynthesis of aromatic amino acids in E. coli. Proteins involved in glucose transport and phosphorylation in strains W3110 and VH33 are also shown. Dashed arrows indicate two or more enzyme reactions. Dotted lines originating from the transcriptional regulator TyrR indicate genes repressed by this protein. Underlined genes were overexpressed from plasmids (aroG fbr, tktA, pheA CM, tyrC and hpaBC) or the chromosome (galP). EI enzyme I, HPr phosphohistidine carrier protein, IICB Glc integral membrane glucose permease, galP galactose permease; glk glucokinase, tktA transketolase gene, ATP adenosine triphosphate, F6P fructose-6-phosphate, G3P glyceraldehyde-3-phosphate, G6P glucose-6-phosphate, E4P d-erythrose 4-phosphate, R5P ribose-5-phosphate, Ru5P ribulose-5-phosphate, S7P sedoheptulose-7-phosphate, X5P xylulose-5-phosphate, PEP phosphoenolpyruvate, PYR pyruvate, AcCoA acetyl-CoA, OAA oxaloacetate, TCA tricarboxylic acid, DAHP 3-deoxy-d-arabino-heptulosonate 7-phosphate, DHQ 3-dehydroquinic acid, DHS dehydroshikimate, SHIK shikimate, S3P shikimate 3-phosphate, EPSP 5-enolpyruvoylshikimate-3-phosphate, CHA chorismate, PPA prephenate, HPPY 4-hydroxyphenylpyruvate, PPY phenylpyruvate, l -TYR l-tyrosine, l -PHE l-phenylalanine, l -TRP l-tryptophan, l -DOPA l-3,4-dihydroxyphenylalanine, aroF DAHP synthase feedback inhibited by l-tyrosine, aroG fbr DAHP synthase feedback inhibition resistant, aroH DAHP synthase feedback inhibited by l-tryptophan, aroB 3-dehydroquinate synthase gene, aroD 3-dehydroquinate dehydratase gene, aroE shikimate dehydrogenase isozyme gene, ydiB shikimate dehydrogenase isozyme gene, aroK shikimate kinase I gene, aroL shikimate kinase II gene, aroA 3-phosphoshikimate-1-carboxyvinyltransferase gene, aroC chorismate synthase gene, tyrA chorismate mutase-prephenate dehydrogenase, pheA chorismate mutase-prephenate dehydratase, tyrC cyclohexadienyl dehydrogenase, pheA CM chorismate mutase domain from chorismate mutase-prephenate dehydratase, tyrB tyrosine aminotransferase gene, hpaBC p-hydroxyphenylacetate 3-hydroxylase genes
The elimination of regulatory constraints has the potential for augmenting flux in a particular biosynthetic pathway. The protein TyrR is a transcriptional dual regulator that represses transcription of several genes encoding enzymes that participate in l-Tyr biosynthesis in E. coli [23]. Therefore, inactivation of TyrR is expected to increase l-Tyr biosynthetic capacity. Strains with inactive tyrR have been employed in l-Tyr production, however, no comparison has been performed with an isogenic tyrR + strain; therefore, the quantitative effect of such modification on production capacity is still not known [18, 28].
In this work, we report the construction of an E. coli strain with the metabolic capacity for converting glucose into l-DOPA. We explored strategies for strain improvement by determining the effects of tyrR inactivation and the PTS− glc+ phenotype on l-Tyr and l-DOPA production from glucose.
Materials and methods
Bacterial strains and plasmids used or constructed in this study are listed in Table 1. Strain VH33 is a derivative of W3110 with an inactive PTS and modified to restore glucose import by replacement of the native galP promoter by the strong trc promoter; glucose phosphorylation is dependent on the endogenous level of Glk [6]. The native galP promoter is repressed by glucose and induced when galactose is present in the medium, whereas the trc promoter is constitutive in strain VH33. As a result of these modifications, VH33 displays a PTS− gluc+ phenotype. Strains W3110ΔtyrR and VH33ΔtyrR are tyrR − derivatives of W3110 and VH33, respectively. Plasmid pJLBaroG fbr tktA has a replication origin from pACYC184, it carries gene aroG fbr encoding a feedback resistant version of DAHP synthase and gene tktA encoding transketolase, both from E. coli [3]. The aroG fbr gene is transcribed from the lacUV5 promoter that is regulated by the LacI repressor and can be induced by addition of isopropyl-β-d-thiogalactopyranoside (IPTG). The tktA gene is transcribed from its native promoter and is considered to be constitutive. Plasmid pTrctyrCpheA CM has a replication origin from pBR322; it carries gene tyrC encoding the feedback inhibition-insensitive enzyme cyclohexadienyl dehydrogenase (TyrC) from Zymomonas mobilis and the gene pheA CM encoding the chorismate mutase domain from chorismate mutase-prephenate dehydratase (PheACM) from E. coli [4]. These genes form an operon transcribed from the trc promoter that is regulated by the LacI repressor.
E. coli strains and plasmids used in this study
Strain or plasmid . | Relevant features . | Reference or source . |
---|---|---|
W3110 | Escherichia coli K-12 F- λ- | ATCC27325 |
VH33 | W3110 ΔptsI, ptsH, crr::kan ΔlacI, lacZ::loxP, PgalP::Ptrc | [6] |
E. coli W | hpaBC operon in chromosome | [16] |
W3110ΔtyrR | W3110 ΔtyrR::FRT | This study |
VH33ΔtyrR | VH33 ΔtyrR::FRT | This study |
W3110_TIR | W3110 transformed with pJLBaroG fbr tktA and pTrctyrCpheA CM | This study |
VH33_TIR | VH33 transformed with pJLBaroG fbr tktA and pTrctyrCpheA CM | This study |
W3110ΔtyrR_TIR | W3110ΔtyrR transformed with pJLBaroG fbr tktA and pTrctyrCpheA CM | This study |
VH33ΔtyrR_TIR | VH33ΔtyrR transformed with pJLBaroG fbr tktA and pTrctyrCpheA CM | This study |
W3110ΔtyrR_DOPA | W3110ΔtyrR transformed with pJLBaroG fbr tktA and pTrchpaBCTrctyrCpheA CM | This study |
VH33ΔtyrR_DOPA | VH33ΔtyrR transformed with pJLBaroG fbr tktA and pTrchpaBCTrctyrCpheA CM | This study |
pTrc99A | Cloning vector, carries bla and lacI q genes and trc promoter | [1] |
pJLBaroG fbr tktA | aroG fbr under control of the lacUV5 promoter; and tktA under its native promoter, carries lacI q and tet genes | [3] |
pTrctyrCpheA CM | tyrC and pheA CM under control of the trc promoter | [4] |
pTrchpaBC | hpaBC under control of the trc promoter | This study |
pTrchpaBCTrctyrCpheA CM | hpaBC, tyrC and pheA CM under control of the trc promoter | This study |
Strain or plasmid . | Relevant features . | Reference or source . |
---|---|---|
W3110 | Escherichia coli K-12 F- λ- | ATCC27325 |
VH33 | W3110 ΔptsI, ptsH, crr::kan ΔlacI, lacZ::loxP, PgalP::Ptrc | [6] |
E. coli W | hpaBC operon in chromosome | [16] |
W3110ΔtyrR | W3110 ΔtyrR::FRT | This study |
VH33ΔtyrR | VH33 ΔtyrR::FRT | This study |
W3110_TIR | W3110 transformed with pJLBaroG fbr tktA and pTrctyrCpheA CM | This study |
VH33_TIR | VH33 transformed with pJLBaroG fbr tktA and pTrctyrCpheA CM | This study |
W3110ΔtyrR_TIR | W3110ΔtyrR transformed with pJLBaroG fbr tktA and pTrctyrCpheA CM | This study |
VH33ΔtyrR_TIR | VH33ΔtyrR transformed with pJLBaroG fbr tktA and pTrctyrCpheA CM | This study |
W3110ΔtyrR_DOPA | W3110ΔtyrR transformed with pJLBaroG fbr tktA and pTrchpaBCTrctyrCpheA CM | This study |
VH33ΔtyrR_DOPA | VH33ΔtyrR transformed with pJLBaroG fbr tktA and pTrchpaBCTrctyrCpheA CM | This study |
pTrc99A | Cloning vector, carries bla and lacI q genes and trc promoter | [1] |
pJLBaroG fbr tktA | aroG fbr under control of the lacUV5 promoter; and tktA under its native promoter, carries lacI q and tet genes | [3] |
pTrctyrCpheA CM | tyrC and pheA CM under control of the trc promoter | [4] |
pTrchpaBC | hpaBC under control of the trc promoter | This study |
pTrchpaBCTrctyrCpheA CM | hpaBC, tyrC and pheA CM under control of the trc promoter | This study |
E. coli strains and plasmids used in this study
Strain or plasmid . | Relevant features . | Reference or source . |
---|---|---|
W3110 | Escherichia coli K-12 F- λ- | ATCC27325 |
VH33 | W3110 ΔptsI, ptsH, crr::kan ΔlacI, lacZ::loxP, PgalP::Ptrc | [6] |
E. coli W | hpaBC operon in chromosome | [16] |
W3110ΔtyrR | W3110 ΔtyrR::FRT | This study |
VH33ΔtyrR | VH33 ΔtyrR::FRT | This study |
W3110_TIR | W3110 transformed with pJLBaroG fbr tktA and pTrctyrCpheA CM | This study |
VH33_TIR | VH33 transformed with pJLBaroG fbr tktA and pTrctyrCpheA CM | This study |
W3110ΔtyrR_TIR | W3110ΔtyrR transformed with pJLBaroG fbr tktA and pTrctyrCpheA CM | This study |
VH33ΔtyrR_TIR | VH33ΔtyrR transformed with pJLBaroG fbr tktA and pTrctyrCpheA CM | This study |
W3110ΔtyrR_DOPA | W3110ΔtyrR transformed with pJLBaroG fbr tktA and pTrchpaBCTrctyrCpheA CM | This study |
VH33ΔtyrR_DOPA | VH33ΔtyrR transformed with pJLBaroG fbr tktA and pTrchpaBCTrctyrCpheA CM | This study |
pTrc99A | Cloning vector, carries bla and lacI q genes and trc promoter | [1] |
pJLBaroG fbr tktA | aroG fbr under control of the lacUV5 promoter; and tktA under its native promoter, carries lacI q and tet genes | [3] |
pTrctyrCpheA CM | tyrC and pheA CM under control of the trc promoter | [4] |
pTrchpaBC | hpaBC under control of the trc promoter | This study |
pTrchpaBCTrctyrCpheA CM | hpaBC, tyrC and pheA CM under control of the trc promoter | This study |
Strain or plasmid . | Relevant features . | Reference or source . |
---|---|---|
W3110 | Escherichia coli K-12 F- λ- | ATCC27325 |
VH33 | W3110 ΔptsI, ptsH, crr::kan ΔlacI, lacZ::loxP, PgalP::Ptrc | [6] |
E. coli W | hpaBC operon in chromosome | [16] |
W3110ΔtyrR | W3110 ΔtyrR::FRT | This study |
VH33ΔtyrR | VH33 ΔtyrR::FRT | This study |
W3110_TIR | W3110 transformed with pJLBaroG fbr tktA and pTrctyrCpheA CM | This study |
VH33_TIR | VH33 transformed with pJLBaroG fbr tktA and pTrctyrCpheA CM | This study |
W3110ΔtyrR_TIR | W3110ΔtyrR transformed with pJLBaroG fbr tktA and pTrctyrCpheA CM | This study |
VH33ΔtyrR_TIR | VH33ΔtyrR transformed with pJLBaroG fbr tktA and pTrctyrCpheA CM | This study |
W3110ΔtyrR_DOPA | W3110ΔtyrR transformed with pJLBaroG fbr tktA and pTrchpaBCTrctyrCpheA CM | This study |
VH33ΔtyrR_DOPA | VH33ΔtyrR transformed with pJLBaroG fbr tktA and pTrchpaBCTrctyrCpheA CM | This study |
pTrc99A | Cloning vector, carries bla and lacI q genes and trc promoter | [1] |
pJLBaroG fbr tktA | aroG fbr under control of the lacUV5 promoter; and tktA under its native promoter, carries lacI q and tet genes | [3] |
pTrctyrCpheA CM | tyrC and pheA CM under control of the trc promoter | [4] |
pTrchpaBC | hpaBC under control of the trc promoter | This study |
pTrchpaBCTrctyrCpheA CM | hpaBC, tyrC and pheA CM under control of the trc promoter | This study |
Deletion of gene tyrR
The tyrR gene was inactivated by employing the method described by Datsenko and Wanner [5]. The following oligonucleotides were employed: TyrR_Fw 5′-GTGTCATATCATCATATTAATTGTTCTTTTTTCAGGTGAAGGTTCCCATGGTGTAGGCTGGAGCTGCTTCG-3′ and TyrR_Rv 5′-TGGTGTTGCACCATCAGGCATATTCGCGCTTACTCTTCGTTCTTCTTCTGCATATGAATATCCTCCTTAG-3′. Plasmid pKD3 was employed as a template to amplify a 1,241-bp PCR product containing a gene conferring chloramphenicol resistance and 50-bp regions of homology to the 5′ and 3′ ends of the tyrR gene. The PCR product was purified and electroporated in electrocompetent strains W3110 and VH33 that contained plasmid pKD46. Transformant cells were selected in solid LB medium containing Cm (30 μg/ml). Candidate tyrR mutants were screened by performing PCR employing the following oligonucleotides that bind outside of the deleted region and in the Cm cassette: TyrR5 5′-GGTGCTGACCGGATATCTTTACG-3′, TyrR3 5′-GGTGTGGCAATTGCTGATGG-3′ and pKD3_Cm 5′-GGTGGTATATCCAGTGATTTTTTTCCAT-3′. The Cm cassette was deleted from tyrR− mutant strains by transforming them with plasmid pCP20 and following the method described by Datsenko and Wanner [5]. The resultant strains having inactive tyrR and lacking the Cm cassette were named W3110ΔtyrR and VH33ΔtyrR.
Construction of plasmid pTrchpaBCTrctyrCpheACM
Genes hpaBC were amplified by PCR from chromosomal DNA of E. coli W employing oligonucleotides EcoRI_HpaBC 5′-GGAATTCCTGTAGAGGTCGACATGAAACC-3′ and BamHI_HpaBC 5′-GCTAAATTCGAGGTCGGGGAAGCCTAGGC-3′ [16]. The 2,127 PCR product and plasmid pTrc99A were digested with EcoRI and BamHI and ligated. The resulting plasmid, pTrchpaBC, contains the hpaBC genes under the transcriptional control of the trc promoter. To construct plasmid pTrchpaBCTrctyrCpheA CM, the region of plasmid pTrctyrCpheA CM, containing the trc promoter and the tyrC and pheA CM genes was obtained by double digestion with PvuII and ScaI and ligated to pTrchpaBC linearized with PvuII. Strains W3110ΔtyrR and VH33ΔtyrR were transformed with plasmids pJLBaroG fbr tktA and pTrchpaBCTrctyrCpheA CM to generate W3110ΔtyrR_DOPA and VH33ΔtyrR_DOPA, respectively.
Growth media, inoculum preparation, and culture conditions
Cells were routinely grown in Luria–Bertani (LB) broth or LB agar plates [20]. M9 mineral medium contained 10 g/l glucose, 6 g/l Na2HPO4, 0.5 g/l NaCl, 3 g/l KH2PO4, 1 g/l NH4Cl, 246.5 mg/l, MgSO4, 14.7 mg/l CaCl2, and 10 μg/ml vitamin B1. Antibiotics were added to the corresponding cultures at a final concentration of 100 μg/ml carbenicillin (Cb), 30 μg/ml tetracycline (Tc), and 30 μg/ml chloramphenicol (Cm) during selection, propagation and fermentation stages. In resting cells cultures, 50 μg/ml Cm was added to arrest cell growth. IPTG was added as an inducer in all cultures to a final concentration of 0.1 mM. Cultures for l-Tyr production were performed in 250-ml baffled shake flasks containing 50 ml of M9 medium supplemented with 10 g/l glucose, Cb, Tc, and IPTG. Flasks were inoculated at an initial optical density at 600 nm of 0.1 and incubated at 37°C with 300 rpm shaking. Resting cell cultures for l-DOPA synthesis from glucose were performed as follows: cells from plate colonies of strains W3110ΔtyrR_DOPA or VH33ΔtyrR_DOPA were used to inoculate at an initial optical density at 600 nm of 0.1, 250-ml baffled shake flasks containing 100 ml of LB medium supplemented with 10 g/l glucose, Cb, and Tc. Shake flasks were incubated at 37°C with 120 rpm shaking, after 1.5 h, IPTG was added and after 3 h cells were centrifuged, washed twice with M9 medium, and resuspended at a dry cell weight (DCW) concentration of 1 ± 0.2 g/l in 250-ml baffled shake flasks containing 35 ml of M9 medium supplemented with glucose 10 g/l and Cm to arrest cell growth. Flasks were incubated at 37°C with 120 rpm shaking. Ascorbic acid is reported to reduce l-DOPA oxidation; therefore, the effect of its addition at 0.45 g/l was also evaluated [12]. Fermentor cultures were performed in 1-l stirred tank bioreactors model ADI 1010 (Applikon, The Netherlands), using a working volume of 500 ml. Culture medium LB was supplemented with 50 g/l glucose, 0.203 g/l MgSO4, 0.0147 g/l CaCl2, thiamine 0.0001 g/l, and 1 ml/l of trace elements. This solution contains 27 g/l FeCl3, 2 g/l ZnCl3, CoCl2·6H2O, 2 g/l Na2MoO4·2H2O, 2 g/l CaCl2·2H2O, 0.5 g/l H3BO3 and 100 ml/l HCl. Cultures were inoculated at an initial OD600nm of 0.1. pH was maintained at 6.0 by automatic addition of 2.9% (v/v) NH4OH and 3.0 N H3PO4. Temperature was controlled at 37°C. Airflow was set to 1 vvm. Dissolved oxygen tension was measured with a polarographic oxygen electrode (Applisens, Applikon) and maintained above 20% air saturation during all cultivation period by modifying the impeller speed. Inducer IPTG was added at a DCW of 6.75 g/l. Results presented from flask and fermentor cultures are the average of at least three independent experiments.
Analytical methods
Growth was monitored by measuring the OD600, and the results were converted to dry weight of cells (1 OD600 unit is equivalent to 0.37 g/l) [11]. Metabolites in the supernatants were analyzed using a Waters HPLC system: 600E quaternary pump, 717 automatic injector, 2410 refraction index, and 996 photodiode array detectors. Measurement of glucose and acetic, SHIK and dehydroshikimic (DHS) acids was performed on an Aminex HPX-87H column (300 × 7.8 mm; 9 mm Bio-Rad, Hercules, CA). Running conditions were: mobile phase, 5 mM H2SO4; flow, 0.5 ml/min and temperature, 50° C. Under these conditions, glucose was detected by refraction index. The other metabolites were identified by a photodiode array at 210 nm. l-Tyr and l-DOPA in culture supernatants were quantified using a Phenomenex Synergy Hydro RP18 column (150 by 4.6 mm; 4 μm) attached to an Agilent 1100 HPLC system (Agilent Technologies, Palo Alto, CA). Running conditions were: mobile phase, 0.2% TFA in 40% methanol; flow, 0.5 ml/min. Detection was performed by photodiode array at 280 nm.
Results
Increasing flux to l-Tyr synthesis by inactivation of tyrR
To quantify the extent of carbon flux increase to l-Tyr synthesis by elimination of TyrR repression, gene tyrR was deleted in PTS+ strain W3110 and PTS− gluc+ strain VH33 to generate strains W3110ΔtyrR and VH33ΔtyrR, respectively. These four strains were transformed with plasmids pJLBaroG fbr tktA and pTrctyrCpheA CM to generate the l-Tyr production strains W3110_TIR, VH33_TIR, W3110ΔtyrR_TIR, and VH33ΔtyrR_TIR (Table 1). These strains were grown in shake flasks containing minimal medium supplemented with 10 g/l glucose. Table 2 shows the parameters calculated from these experiments. Inactivation of tyrR had a negative impact on the specific growth rate (μ) and the specific glucose consumption rate (q glucose) only in the PTS− gluc+ strain VH33ΔtyrR_TIR. The overflow metabolite acetic acid accumulated in cultures of strains W3110_TIR and W3110ΔtyrR_TIR at 1.4 g/l. In contrast, acetic acid reached a low final concentration of 0.05 g/l in cultures of both PTS− gluc+ strains. Low acetate production has been observed in other PTS− strains generated for the production of anthranilate or recombinant proteins [3, 6]. This effect is the result of lower glucose import rate and a lower rate of PYR production as a result of PTS inactivation.
Comparison of kinetic and stoichiometric parameters of l-Tyr production strains in shake flask experiments
Strain . | μ (h−1) . | q glucose (g/g/h) . | YBiom/Glc (g/g) . | q l-Tyr (mg/g/h) . | Yl-Tyr/Glc (mg/g) . | Final l-tyr titer (mg/l) . |
---|---|---|---|---|---|---|
W3110_TIR | 0.53 ± 0.02 | 0.37 ± 0.02 | 0.36 ± 0.01 | 12 ± 2 | 32 ± 2 | 150 ± 20 |
W3110ΔtyrR_TIR | 0.40 ± 0.02 | 0.47 ± 0.02 | 0.24 ± 0.02 | 20 ± 2 | 42 ± 2 | 220 ± 30 |
VH33_TIR | 0.21 ± 0.02 | 0.28 ± 0.02 | 0.54 ± 0.02 | 33 ± 3.4 | 118 ± 20 | 220 ± 20 |
VH33ΔtyrR_TIR | 0.10 ± 0.01 | 0.18 ± 0.03 | 0.14 ± 0.02 | 65 ± 4 | 361 ± 30 | 150 ± 30 |
Strain . | μ (h−1) . | q glucose (g/g/h) . | YBiom/Glc (g/g) . | q l-Tyr (mg/g/h) . | Yl-Tyr/Glc (mg/g) . | Final l-tyr titer (mg/l) . |
---|---|---|---|---|---|---|
W3110_TIR | 0.53 ± 0.02 | 0.37 ± 0.02 | 0.36 ± 0.01 | 12 ± 2 | 32 ± 2 | 150 ± 20 |
W3110ΔtyrR_TIR | 0.40 ± 0.02 | 0.47 ± 0.02 | 0.24 ± 0.02 | 20 ± 2 | 42 ± 2 | 220 ± 30 |
VH33_TIR | 0.21 ± 0.02 | 0.28 ± 0.02 | 0.54 ± 0.02 | 33 ± 3.4 | 118 ± 20 | 220 ± 20 |
VH33ΔtyrR_TIR | 0.10 ± 0.01 | 0.18 ± 0.03 | 0.14 ± 0.02 | 65 ± 4 | 361 ± 30 | 150 ± 30 |
Specific growth rate (μ), specific glucose consumption rate (q glucose), biomass yield from total glucose consumed (YBiom/Glc), specific rate of l-Tyr production (q l-Tyr), l-Tyr yield from total glucose consumed (Yl-Tyr/Glc). Values presented are the average of at least three independent experiments
Comparison of kinetic and stoichiometric parameters of l-Tyr production strains in shake flask experiments
Strain . | μ (h−1) . | q glucose (g/g/h) . | YBiom/Glc (g/g) . | q l-Tyr (mg/g/h) . | Yl-Tyr/Glc (mg/g) . | Final l-tyr titer (mg/l) . |
---|---|---|---|---|---|---|
W3110_TIR | 0.53 ± 0.02 | 0.37 ± 0.02 | 0.36 ± 0.01 | 12 ± 2 | 32 ± 2 | 150 ± 20 |
W3110ΔtyrR_TIR | 0.40 ± 0.02 | 0.47 ± 0.02 | 0.24 ± 0.02 | 20 ± 2 | 42 ± 2 | 220 ± 30 |
VH33_TIR | 0.21 ± 0.02 | 0.28 ± 0.02 | 0.54 ± 0.02 | 33 ± 3.4 | 118 ± 20 | 220 ± 20 |
VH33ΔtyrR_TIR | 0.10 ± 0.01 | 0.18 ± 0.03 | 0.14 ± 0.02 | 65 ± 4 | 361 ± 30 | 150 ± 30 |
Strain . | μ (h−1) . | q glucose (g/g/h) . | YBiom/Glc (g/g) . | q l-Tyr (mg/g/h) . | Yl-Tyr/Glc (mg/g) . | Final l-tyr titer (mg/l) . |
---|---|---|---|---|---|---|
W3110_TIR | 0.53 ± 0.02 | 0.37 ± 0.02 | 0.36 ± 0.01 | 12 ± 2 | 32 ± 2 | 150 ± 20 |
W3110ΔtyrR_TIR | 0.40 ± 0.02 | 0.47 ± 0.02 | 0.24 ± 0.02 | 20 ± 2 | 42 ± 2 | 220 ± 30 |
VH33_TIR | 0.21 ± 0.02 | 0.28 ± 0.02 | 0.54 ± 0.02 | 33 ± 3.4 | 118 ± 20 | 220 ± 20 |
VH33ΔtyrR_TIR | 0.10 ± 0.01 | 0.18 ± 0.03 | 0.14 ± 0.02 | 65 ± 4 | 361 ± 30 | 150 ± 30 |
Specific growth rate (μ), specific glucose consumption rate (q glucose), biomass yield from total glucose consumed (YBiom/Glc), specific rate of l-Tyr production (q l-Tyr), l-Tyr yield from total glucose consumed (Yl-Tyr/Glc). Values presented are the average of at least three independent experiments
The PTS+ strain W3110_TIR showed a specific rate of l-Tyr production (q L-Tyr) of 12 ± 2 mg/g/h, whereas in the isogenic tyrR − strain W3110ΔtyrR_TIR, this value was 1.7 fold higher. The PTS− gluc+ strains VH33_TIR and VH33ΔtyrR_TIR showed q l-Tyr values of 33 ± 3.4 and 65 ± 4 mg/g/h, respectively. Strains W3110_TIR and W3110ΔtyrR_TIR showed l-Tyr yields from total glucose consumed (Yl-Tyr/Glc) of 32 ± 2 and 42 ± 2 mg/g, respectively. In strains VH33_TIR and VH33ΔtyrR_TIR, Yl-Tyr/Glc was higher, corresponding to 118 ± 20 and 361 ± 30 mg/g, respectively. The intermediates of the SHIK pathway, DHS and SHIK were detected only in cultures with strain VH33_TIR, were they accumulated to a final concentration of 134 ± 4 and 340 ± 11 mg/l, respectively.
Synthesis of l-DOPA in strains expressing 4-hydroxyphenylacetate 3-hydroxylase
Genes hpaBC encoding the enzyme 4-hydroxyphenylacetate 3-hydroxylase from E. coli W were amplified by PCR and employed to generate plasmid pTrchpaBCTrctyrCpheA CM. This plasmid was used to generate strains W3110ΔtyrR_DOPA and VH33ΔtyrR_DOPA (Table 1). These two strains displayed a brown color after 20 h incubation at 37°C, a phenotype resulting from hpaBC overexpression, as reported previously [24]. The capacity of these strains to produce l-DOPA from glucose was assayed in resting cells shake flask cultures. Under these conditions, q glucose values were similar for the four cultures (Table 3). In the resting cell cultures lacking ascorbic acid, strains W3110ΔtyrR_DOPA and VH33ΔtyrR_DOPA displayed specific rates of l-DOPA production (q L-DOPA) of 12 ± 1 and 14 ± 1 mg/g/h, respectively. No significant difference in this parameter was observed when comparing with cultures where ascorbic acid was added. The calculated l-DOPA yields from glucose (YL-DOPA/Glc) in the absence of ascorbic acid for W3110ΔtyrR_DOPA and VH33ΔtyrR_DOPA corresponded to 35 ± 1 and 54 ± 7 mg/g, respectively. The highest final l-DOPA titers (313 ± 60 and 320 ± 100 mg/l) were observed for cultures with strain VH33ΔtyrR_DOPA.
Comparison of kinetic and fermentation parameters of l-DOPA production strains in resting cells shake flask experiments
Strain . | Ascorbic acid (g/l) . | q glucose (g/g/h) . | q l-DOPA (mg/g/h) . | Yl-DOPA/Glc (mg/g) . | l-DOPA titer (mg/l) . | DHS titer (mg/l) . | SHIK titer (mg/l) . | l-Tyr titer (mg/l) . |
---|---|---|---|---|---|---|---|---|
W3110ΔtyrR_DOPA | 0 | 0.34 ± 0.02 | 12 ± 1 | 35 ± 1 | 235 ± 50 | 0 | 248 ± 12 | 60 ± 11 |
W3110ΔtyrR_DOPA | 0.45 | 0.27 ± 0.01 | 11 ± 1 | 41 ± 4 | 269 ± 150 | 0 | 228 ± 15 | 62 ± 21 |
VH33ΔtyrR_DOPA | 0 | 0.26 ± 0.03 | 14 ± 1 | 54 ± 7 | 313 ± 60 | 225 ± 71 | 226 ± 11 | 51 ± 42 |
VH33ΔtyrR_DOPA | 0.45 | 0.23 ± 0.01 | 12 ± 1 | 54 ± 10 | 320 ± 100 | 312 ± 11 | 271 ± 72 | 22 ± 19 |
Strain . | Ascorbic acid (g/l) . | q glucose (g/g/h) . | q l-DOPA (mg/g/h) . | Yl-DOPA/Glc (mg/g) . | l-DOPA titer (mg/l) . | DHS titer (mg/l) . | SHIK titer (mg/l) . | l-Tyr titer (mg/l) . |
---|---|---|---|---|---|---|---|---|
W3110ΔtyrR_DOPA | 0 | 0.34 ± 0.02 | 12 ± 1 | 35 ± 1 | 235 ± 50 | 0 | 248 ± 12 | 60 ± 11 |
W3110ΔtyrR_DOPA | 0.45 | 0.27 ± 0.01 | 11 ± 1 | 41 ± 4 | 269 ± 150 | 0 | 228 ± 15 | 62 ± 21 |
VH33ΔtyrR_DOPA | 0 | 0.26 ± 0.03 | 14 ± 1 | 54 ± 7 | 313 ± 60 | 225 ± 71 | 226 ± 11 | 51 ± 42 |
VH33ΔtyrR_DOPA | 0.45 | 0.23 ± 0.01 | 12 ± 1 | 54 ± 10 | 320 ± 100 | 312 ± 11 | 271 ± 72 | 22 ± 19 |
Specific glucose consumption rate (q glucose), specific rate of l-DOPA production (q l-DOPA), l-DOPA yield from total glucose consumed (Yl-DOPA/Glc). Values presented are the average of at least three independent experiments
Comparison of kinetic and fermentation parameters of l-DOPA production strains in resting cells shake flask experiments
Strain . | Ascorbic acid (g/l) . | q glucose (g/g/h) . | q l-DOPA (mg/g/h) . | Yl-DOPA/Glc (mg/g) . | l-DOPA titer (mg/l) . | DHS titer (mg/l) . | SHIK titer (mg/l) . | l-Tyr titer (mg/l) . |
---|---|---|---|---|---|---|---|---|
W3110ΔtyrR_DOPA | 0 | 0.34 ± 0.02 | 12 ± 1 | 35 ± 1 | 235 ± 50 | 0 | 248 ± 12 | 60 ± 11 |
W3110ΔtyrR_DOPA | 0.45 | 0.27 ± 0.01 | 11 ± 1 | 41 ± 4 | 269 ± 150 | 0 | 228 ± 15 | 62 ± 21 |
VH33ΔtyrR_DOPA | 0 | 0.26 ± 0.03 | 14 ± 1 | 54 ± 7 | 313 ± 60 | 225 ± 71 | 226 ± 11 | 51 ± 42 |
VH33ΔtyrR_DOPA | 0.45 | 0.23 ± 0.01 | 12 ± 1 | 54 ± 10 | 320 ± 100 | 312 ± 11 | 271 ± 72 | 22 ± 19 |
Strain . | Ascorbic acid (g/l) . | q glucose (g/g/h) . | q l-DOPA (mg/g/h) . | Yl-DOPA/Glc (mg/g) . | l-DOPA titer (mg/l) . | DHS titer (mg/l) . | SHIK titer (mg/l) . | l-Tyr titer (mg/l) . |
---|---|---|---|---|---|---|---|---|
W3110ΔtyrR_DOPA | 0 | 0.34 ± 0.02 | 12 ± 1 | 35 ± 1 | 235 ± 50 | 0 | 248 ± 12 | 60 ± 11 |
W3110ΔtyrR_DOPA | 0.45 | 0.27 ± 0.01 | 11 ± 1 | 41 ± 4 | 269 ± 150 | 0 | 228 ± 15 | 62 ± 21 |
VH33ΔtyrR_DOPA | 0 | 0.26 ± 0.03 | 14 ± 1 | 54 ± 7 | 313 ± 60 | 225 ± 71 | 226 ± 11 | 51 ± 42 |
VH33ΔtyrR_DOPA | 0.45 | 0.23 ± 0.01 | 12 ± 1 | 54 ± 10 | 320 ± 100 | 312 ± 11 | 271 ± 72 | 22 ± 19 |
Specific glucose consumption rate (q glucose), specific rate of l-DOPA production (q l-DOPA), l-DOPA yield from total glucose consumed (Yl-DOPA/Glc). Values presented are the average of at least three independent experiments
The l-DOPA precursors SHIK and l-TYR were detected in the supernatants of cultures with strain W3110ΔtyrR_DOPA (Table 3). In cultures of strain VH33ΔtyrR_DOPA the l-DOPA intermediates DHS, SHIK and l-TYR were detected at a total concentration corresponding to nearly twice the amount of l-DOPA produced (Table 3).
Batch fermentor culture for l-DOPA production
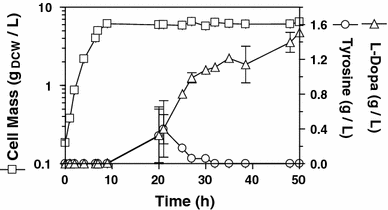
Kinetic behavior trajectories of VH33ΔtyrR_DOPA in batch culture. Open squares, Biomass concentration; open circles, l-Tyr concentration; open triangles, l-DOPA concentration. Results presented are the average of three independent experiments
Discussion
The aim of this work was to generate E. coli strains for the production of l-DOPA from glucose and quantify the effects of PTS functional replacement and TyrR inactivation on l-Tyr and l-DOPA production parameters. PTS impairment in E. coli has been explored as a metabolic engineering strategy for increasing PEP metabolic availability and thus augment carbon flow into aromatic biosynthetic pathways [2, 7]. In this work, a clear positive effect on YL-Tyr/Glc was observed when comparing strains with the PTS− gluc+ and the PTS+ phenotypes. In the tyrR + strains, PTS inactivation caused a 3.7-fold increase in YL-Tyr/Glc, while in the tyrR − mutants, an 8.6-fold increase was observed. The accumulation of the intermediates of the SHIK pathway, DHS and SHIK was detected in the PTS− gluc+ strain VH33_TIR and not in the PTS+ strain W3110_TIR. This result indicates a higher carbon flux into the SHIK pathway in the PTS− gluc+ strain that surpasses the capacity of some of its enzymes, resulting in the accumulation and secretion of intermediates. For production of l-DOPA, strains with the PTS− gluc+ phenotype displayed the best parameters; however, the level of improvement over the PTS+ strains was not as large as in the case for l-Tyr. This result is likely caused by 4-hydroxyphenylacetate 3-hydroxylase limiting activity, since l-Tyr accumulated to a level comparable to l-DOPA (Table 3).
Previous studies have reported the use of strains with an inactive tyrR gene for l-Tyr production [18, 28]. However, in these reports, no comparison was performed with an isogenic tyrR + strain. Therefore, the quantitative performance improvement caused by tyrR disruption was not known. Our study showed that elimination of tyrR leads to 1.7- and 1.9-fold higher q L-Tyr in a PTS+ or a PTS− glucose+ background, respectively. Accumulation of DHS and SHIK was detected in cultures of strain VH33_TIR and not with VH33ΔtyrR_TIR. This result strongly suggests that enhancement of l-Tyr production in the latter strain can be explained in part by the relief of metabolic bottlenecks in the SHIK pathway that was detected in the PTS− glucose+ background as a result of higher PEP availability. TyrR exerts repression on genes aroF, aroL, tyrA and tyrB, encoding l-Tyr feedback inhibited DAHP synthase, shikimate kinase II, chorismate mutase-prephenate dehydrogenase and tyrosine aminotransferase, respectively. These genes are members of the TyrR regulon [23]. The observed positive effect in the tyrR − strain is likely the result of increased transcript level and corresponding specific activity for several of these proteins. Previous works have shown that overexpression of cloned tyrB and/or aroL genes leads to increased aromatics production capacity [19, 27].
Ascorbic acid has been reported to decrease oxidation of l-DOPA under production conditions [12]. In our study, both the YL-DOPA/Glc and the final l-DOPA titers did not change significantly as a result of ascorbic acid addition to the culture medium. These results suggest that under our production conditions and within the time frame of the analysis, no appreciable amount of l-DOPA is lost due to oxidation.
An E. coli strain that converts glucose to l-DOPA has previously been reported [14]. This strain was generated by expressing genes encoding a feedback inhibition insensitive mutant version of DAHP synthase, a feedback-sensitive chorismate mutase-prephenate dehydrogenase and p-hydroxyphenylacetate 3-hydroxylase. In shake flask experiments, this strain produced 30 mg/l of l-DOPA in 24 h. Fed-batch fermentor cultures were also performed with this strain, however, only relative l-DOPA concentrations were provided. The best production strain generated in our study had similar genetic modifications as those present in the strain reported by Kramer et al. [14], but in addition, strain VH33ΔtyrR_DOPA overexpresses tktA, glucose import is PEP-independent and repression by TyrR is absent. These modifications were chosen to increase and avoid limitation of aromatic precursors PEP and E4P, as well as to redirect these two intermediates to the SHIK and l-Tyr pathways by expressing feedback inhibition resistant versions of key enzymes and finally, partially eliminating pathway bottlenecks by inactivation of TyrR. This engineered phenotype allowed the production of 320 mg/l of l-DOPA from glucose in minimal medium and 1.51 g/l in complex medium.
The results obtained in this study enable the identification of targets for further improvement of l-DOPA production strains. Even though repression on certain genes from the SHIK and l-Tyr pathways was eliminated by inactivating tyrR; accumulation of DHS and SHIK was still detected. This result suggests that overexpression of some genes from these pathways has the potential for increasing l-Tyr and l-DOPA production. Combinatorial pathway analysis of l-Tyr production strains has identified genes aroK and ydiB, coding for SHIK kinase and SHIK dehydrogenase, respectively, as specific targets for increasing flux in the common aromatic pathway [19]. In addition, it was found that not all the l-Tyr was converted to l-DOPA. This result indicates that the rate of l-Tyr synthesis surpasses the rate of hydroxylation by the enzyme 4-hydroxyphenylacetate 3-hydroxylase. Therefore, higher expression of hpaBC genes and/or the expression of an enzyme with higher catalytic efficiency have the potential for improving l-Tyr to l-DOPA transformation.
Acknowledgments
This work was supported by CONACyT grants 83039 and 126793. AJM was supported by a fellowship from CONACyT. We thank Luz María Martínez, Mercedes Enzaldo, Juan Manuel Hurtado, Mario Trejo and Martín Patiño for technical assistance.
References
Kramer M, Kremer-Muschen S, Wubbolts MG (2006) Process for the preparation of l-3, 4-dihydroxyphenylalanine by aerobic fermentation of a microorganism. US Patent 2006/0141587A1