-
PDF
- Split View
-
Views
-
Cite
Cite
Rongliang Wang, Ling Zhang, Dongmei Wang, Xiaolian Gao, Jiong Hong, Identification of a xylulokinase catalyzing xylulose phosphorylation in the xylose metabolic pathway of Kluyveromyces marxianus NBRC1777, Journal of Industrial Microbiology and Biotechnology, Volume 38, Issue 10, 1 October 2011, Pages 1739–1746, https://doi.org/10.1007/s10295-011-0963-2
- Share Icon Share
Abstract
Xylulokinase is one of the key enzymes in xylose metabolism and fermentation, and fine-tuned expression of xylulokinase can improve xylose fermentation in yeast. To improve the efficiency of xylose fermentation in Kluyveromyces marxianus, the gene KmXYL3, which encodes a d-xylulokinase (E.C. 2.7.1.17), was isolated from K. marxianus NBRC1777. KmXYL3 was expressed in Escherichia coli BL21 (DE3) cells, and the specific activity of the resulting recombinant purified xylulokinase was 23.5 mU/mg. Disruption of KmXYL3 resulted in both loss of xylitol utilization and marked decrease in xylose utilization, proving that KmXYL3 encodes a xylulokinase that catalyzes the reaction from xylulose to xylulose 5-phosphate in the xylose metabolic pathway. The slow assimilation of xylose observed in the KmXYL3-disrupted strain indicates that KmXYL3 is critical for xylose and xylitol utilization; however, K. marxianus utilizes a bypass pathway for xylose assimilation, and this pathway does not involve xylitol or xylulose.
Introduction
Lignocellulose has attracted much attention owing to its sustainability as a source for the production of biofuel and other chemicals. Hemicellulose, which is one of the main components of lignocellulose, can be easily hydrolyzed by acid or enzymes to produce xylose (85–90%) [1]. In addition to glucose, xylose is another major lignocellulose hydrolysis product and is the second most abundant sugar in nature [16]. Xylose fermentation is one of the commonly used processes for converting renewable biomass into energy sources such as ethanol; however, the yield of ethanol production through this process is low.
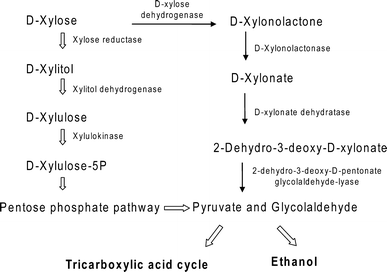
The normal d-xylose metabolic pathway (wide arrow) and the presumed bypass pathway of d-xylose through d-xylonolactone and d-xylonate (line arrow) in yeast
The yeast K. marxianus is considered as a generally regarded as safe (GRAS) microorganism and has advantages such as short generation time and high growth rate at elevated temperatures (0.86–0.99 h−1 at 40°C), with an upper growth limit of 52°C [4, 7]. K. marxianus also has great ethanol-producing capacity at elevated temperatures [4, 19, 26] and can thus potentially be applied in simultaneous saccharification and fermentation (SSF) of lignocellulose and other biomass at temperature higher than 40°C [2, 12], a characteristic that would be useful in the ethanol biofuel industry.
Although K. marxianus can assimilate xylose and grow at high temperatures [4], its ability to ferment xylose is poor [4, 5, 24, 33]. Elucidation of the limiting step of xylose fermentation in K. marxianus will help to optimize the process of constructing a highly efficient, high-temperature fermentation strain for biomass conversion.
Xylulokinase plays a critical role in xylose metabolism in all natural and engineered microorganisms. The expression level of the enzyme greatly affects xylose fermentation in yeast, and it is reported that fine-tuned overexpression of xylulokinase can improve xylose fermentation to ethanol [14, 22, 32]. Although approximately 30 xylulokinase or potential xylulokinase genes from yeast can be found in GenBank, most of them are hypothetical proteins or theoretically predicted as xylulokinase. Only the xylulokinases from Saccharomyces cerevisiae, Pichia stipitis, and Candida maltosa [13, 21, 27] were cloned, expressed, and characterized. The gene encoding xylulokinase from Pachysolen tannophilus was cloned and characterized [30], but the sequence is currently unavailable in GenBank.
In this study, a xylulokinase gene, KmXYL3, was cloned from K. marxianus. The recombinant enzyme KmXK was expressed in E. coli and purified for further experiments. KmXYL3 gene disruption and KmXK activity characterization confirmed that KmXYL3 codes for a xylulokinase that catalyzes conversion of xylulose to xylulose 5-P in K. marxianus. In addition, the KmXYL3 disruption experiment indicated the presence of a bypass metabolic pathway that does not involve xylitol and xylulose (Fig. 1). These findings provide important information for future genetic engineering advances to improve xylose fermentation efficiency in K. marxianus.
Materials and methods
Chemical and microorganism strains
All chemicals used were of reagent or higher grade and were purchased from Sangon Biotech Co. (Shanghai, China) unless otherwise noted. Yeast extract, peptone, and tryptone were purchased from OXOID (Cambridge, UK). Restriction endonucleases and T4 DNA ligase were from Fermentas China (Shenzhen, China). Xylulose was from Sigma (St. Louis, MO, USA). PrimeStar HS DNA polymerase from TAKARA (Dalian, China) was used for polymerase chain reaction (PCR). E. coli DH5 was used as a host cell for all DNA manipulation. E. coli BL21 (DE3) (Invitrogen, Carlsbad, CA, USA) containing a protein expression plasmid was used to express the recombinant KmXK. Luria–Bertani (LB; tryptone 10 g, yeast extract 5 g, and NaCl 10 g/l, pH 7.0) medium was used for E. coli growth and protein expression with 100 μg/ml ampicillin. K. marxianus NBRC1777 (NBRC, Japan) and K. marxianus YHJ010 (ΔKmura3::Kanr ΔKmleu2::hisG, ΔKmtrp1::hisG, auxotroph strain derived from K. marxianus NBRC1777) (Table 1) [19] were grown on solid or liquid YPD medium (peptone 20 g, yeast extract 10 g, and glucose 20 g/l). Synthetic dropout (SD) medium (yeast nitrogen base 6.7 g; glucose, xylose, or xylitol 20 g/l with appropriate amino acids) was used to screen and confirm gene disruption.
Yeast strains used in the study
Strain . | Genotype . | Phenotype . | Source or reference . |
---|---|---|---|
K. marxianus | |||
NBRC1777 | Wild type | XK wild type | NBRC |
YHJ010 | ΔKmura3::Kanr | XK wild type | [19] |
ΔKmleu2::hisG | URA3, LEU2, TRP1 | ||
ΔKmtrp1::hisG | Auxotroph | This study | |
YWRL001 | ΔKmleu2::Kanr | XK, URA3, LEU2 | |
ΔKmleu2::his G | Auxotroph | ||
ΔKmtrp1::hisG KmXYL33::Sc | |||
TRP 1 |
Strain . | Genotype . | Phenotype . | Source or reference . |
---|---|---|---|
K. marxianus | |||
NBRC1777 | Wild type | XK wild type | NBRC |
YHJ010 | ΔKmura3::Kanr | XK wild type | [19] |
ΔKmleu2::hisG | URA3, LEU2, TRP1 | ||
ΔKmtrp1::hisG | Auxotroph | This study | |
YWRL001 | ΔKmleu2::Kanr | XK, URA3, LEU2 | |
ΔKmleu2::his G | Auxotroph | ||
ΔKmtrp1::hisG KmXYL33::Sc | |||
TRP 1 |
Yeast strains used in the study
Strain . | Genotype . | Phenotype . | Source or reference . |
---|---|---|---|
K. marxianus | |||
NBRC1777 | Wild type | XK wild type | NBRC |
YHJ010 | ΔKmura3::Kanr | XK wild type | [19] |
ΔKmleu2::hisG | URA3, LEU2, TRP1 | ||
ΔKmtrp1::hisG | Auxotroph | This study | |
YWRL001 | ΔKmleu2::Kanr | XK, URA3, LEU2 | |
ΔKmleu2::his G | Auxotroph | ||
ΔKmtrp1::hisG KmXYL33::Sc | |||
TRP 1 |
Strain . | Genotype . | Phenotype . | Source or reference . |
---|---|---|---|
K. marxianus | |||
NBRC1777 | Wild type | XK wild type | NBRC |
YHJ010 | ΔKmura3::Kanr | XK wild type | [19] |
ΔKmleu2::hisG | URA3, LEU2, TRP1 | ||
ΔKmtrp1::hisG | Auxotroph | This study | |
YWRL001 | ΔKmleu2::Kanr | XK, URA3, LEU2 | |
ΔKmleu2::his G | Auxotroph | ||
ΔKmtrp1::hisG KmXYL33::Sc | |||
TRP 1 |
Cloning of XK gene KmXYL3 from K. marxianus
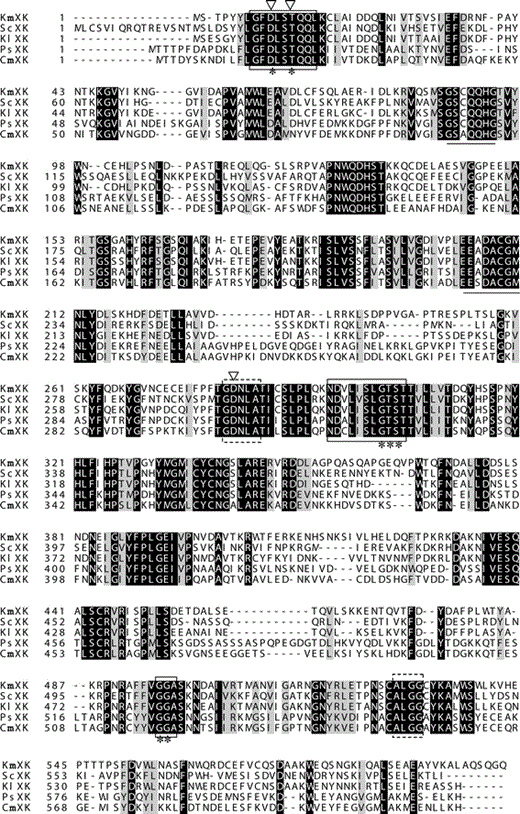
Sequence alignment of KmXK with other XKs from various microorganisms. The XKs aligned were derived from K. marxianus (KmXK, GenBank accession no. GU586191, in this study), S. cerevisiae (ScXK, GenBank accession no. EEU06528), K. lactis (KlXK, GenBank accession no. XP_454390), P. stipitis (PsXK, GenBank accession no. EAZ63302), and C. maltosa (CmXK, GenBank accession no. AAY87404). Highly conserved motifs of phosphate 1, phosphate 2, and adenosine are enclosed in boxes. Connect 1 and connect 2 are enclosed in dashed line boxes. D10, T13, G302, T303, S304, G496, and G497, indicated by asterisks, are amino acids that are common to all sugar kinases. D10, T13, and D282 indicated by inverted triangles are catalytic residues and are conserved across the ATPase superfamily
After the DNA fragment of the partial KmXYL3 gene was obtained and sequenced, the unknown flanking sequences were amplified by thermal asymmetric interlaced PCR (TAIL-PCR) [23]. The specific 3′ and 5′ primers for the flanking sequence, which were designed on the basis of the cloned DNA fragment of KmXYL3, were XK-FS1, FS2, FS3 and XK-RS1, RS2, RS3 (Table 2). The arbitrary degenerate (AD) primers were AD1, AD2, AD3, and AD4. The arbitrary primer for the second and third round of TAIL-PCR was AC1. The PCR cycle conditions were the same as described by Liu [23]. The amplified flanking sequences were cloned into pMD18-T, and the sequences were validated.
Primers used in this study
Primer name . | Sequence (5′→3′) . |
---|---|
XK-F1 | GGKTCYTGYCARCARCACGG |
XK-R1 | TTCATWCCACAVGCATCDGC |
XK-EcoRI-F | ACGTGAATTCAGATGTCTACCCCATACTATTTAGGTTTCG |
XK-HindIII-R | GGGTTCGAAGACTGGCCCTGGCTCTGG |
XK-knock-F | TCTACCCCATACTATTTAGGTTTCGATC |
XK-knock-R | GACTGGCCCTGGCTCTGGGCCAGG |
TRP1-EcoRI-F | AGTCGAATTCTTATCCCATTCCATGCGGGGTATC |
TRP1-EcoRI-R | AGTCGAATTCACTCTTCCTTTTTCAATATTATTG |
XK-FS2 | CAGTGCGATGAGTTGGCAGAAAGCGTTGGA |
XK-FS3 | GACCGAGCCCGAGGTTTACG |
XK-FS1 | GCGAACACTTGCCCTCAAACCTGG |
XK-RS1 | TCGTAAACCTCGGGCTCGGTCTCGTGGATC |
XK-RS2 | CCAACGCTTTCTGCCAACTC |
XK-RS3 | CTTCGCGAACAGTTGCAAGG |
AD1 | ACGATGGACTCCAGAGAVNVNNNGGAA |
AD2 | ACGATGGACTCCAGAGABNBNNNGGTT |
AD3 | ACGATGGACTCCAGAGVVNVNNNCCAA |
AD4 | ACGATGGACTCCAGAGBBNBNNNCGGT |
AC1 | ACGATGGACTCCAGAG |
Primer name . | Sequence (5′→3′) . |
---|---|
XK-F1 | GGKTCYTGYCARCARCACGG |
XK-R1 | TTCATWCCACAVGCATCDGC |
XK-EcoRI-F | ACGTGAATTCAGATGTCTACCCCATACTATTTAGGTTTCG |
XK-HindIII-R | GGGTTCGAAGACTGGCCCTGGCTCTGG |
XK-knock-F | TCTACCCCATACTATTTAGGTTTCGATC |
XK-knock-R | GACTGGCCCTGGCTCTGGGCCAGG |
TRP1-EcoRI-F | AGTCGAATTCTTATCCCATTCCATGCGGGGTATC |
TRP1-EcoRI-R | AGTCGAATTCACTCTTCCTTTTTCAATATTATTG |
XK-FS2 | CAGTGCGATGAGTTGGCAGAAAGCGTTGGA |
XK-FS3 | GACCGAGCCCGAGGTTTACG |
XK-FS1 | GCGAACACTTGCCCTCAAACCTGG |
XK-RS1 | TCGTAAACCTCGGGCTCGGTCTCGTGGATC |
XK-RS2 | CCAACGCTTTCTGCCAACTC |
XK-RS3 | CTTCGCGAACAGTTGCAAGG |
AD1 | ACGATGGACTCCAGAGAVNVNNNGGAA |
AD2 | ACGATGGACTCCAGAGABNBNNNGGTT |
AD3 | ACGATGGACTCCAGAGVVNVNNNCCAA |
AD4 | ACGATGGACTCCAGAGBBNBNNNCGGT |
AC1 | ACGATGGACTCCAGAG |
* Restriction sites are underlined
** Codes for degenerate bases in primers: K (G or T), Y(C or T), R (A or G), W (A or T), B (C, G, or T), V (A, C, or G), D (A, G, or T), N (A, C, G, or T)
Primers used in this study
Primer name . | Sequence (5′→3′) . |
---|---|
XK-F1 | GGKTCYTGYCARCARCACGG |
XK-R1 | TTCATWCCACAVGCATCDGC |
XK-EcoRI-F | ACGTGAATTCAGATGTCTACCCCATACTATTTAGGTTTCG |
XK-HindIII-R | GGGTTCGAAGACTGGCCCTGGCTCTGG |
XK-knock-F | TCTACCCCATACTATTTAGGTTTCGATC |
XK-knock-R | GACTGGCCCTGGCTCTGGGCCAGG |
TRP1-EcoRI-F | AGTCGAATTCTTATCCCATTCCATGCGGGGTATC |
TRP1-EcoRI-R | AGTCGAATTCACTCTTCCTTTTTCAATATTATTG |
XK-FS2 | CAGTGCGATGAGTTGGCAGAAAGCGTTGGA |
XK-FS3 | GACCGAGCCCGAGGTTTACG |
XK-FS1 | GCGAACACTTGCCCTCAAACCTGG |
XK-RS1 | TCGTAAACCTCGGGCTCGGTCTCGTGGATC |
XK-RS2 | CCAACGCTTTCTGCCAACTC |
XK-RS3 | CTTCGCGAACAGTTGCAAGG |
AD1 | ACGATGGACTCCAGAGAVNVNNNGGAA |
AD2 | ACGATGGACTCCAGAGABNBNNNGGTT |
AD3 | ACGATGGACTCCAGAGVVNVNNNCCAA |
AD4 | ACGATGGACTCCAGAGBBNBNNNCGGT |
AC1 | ACGATGGACTCCAGAG |
Primer name . | Sequence (5′→3′) . |
---|---|
XK-F1 | GGKTCYTGYCARCARCACGG |
XK-R1 | TTCATWCCACAVGCATCDGC |
XK-EcoRI-F | ACGTGAATTCAGATGTCTACCCCATACTATTTAGGTTTCG |
XK-HindIII-R | GGGTTCGAAGACTGGCCCTGGCTCTGG |
XK-knock-F | TCTACCCCATACTATTTAGGTTTCGATC |
XK-knock-R | GACTGGCCCTGGCTCTGGGCCAGG |
TRP1-EcoRI-F | AGTCGAATTCTTATCCCATTCCATGCGGGGTATC |
TRP1-EcoRI-R | AGTCGAATTCACTCTTCCTTTTTCAATATTATTG |
XK-FS2 | CAGTGCGATGAGTTGGCAGAAAGCGTTGGA |
XK-FS3 | GACCGAGCCCGAGGTTTACG |
XK-FS1 | GCGAACACTTGCCCTCAAACCTGG |
XK-RS1 | TCGTAAACCTCGGGCTCGGTCTCGTGGATC |
XK-RS2 | CCAACGCTTTCTGCCAACTC |
XK-RS3 | CTTCGCGAACAGTTGCAAGG |
AD1 | ACGATGGACTCCAGAGAVNVNNNGGAA |
AD2 | ACGATGGACTCCAGAGABNBNNNGGTT |
AD3 | ACGATGGACTCCAGAGVVNVNNNCCAA |
AD4 | ACGATGGACTCCAGAGBBNBNNNCGGT |
AC1 | ACGATGGACTCCAGAG |
* Restriction sites are underlined
** Codes for degenerate bases in primers: K (G or T), Y(C or T), R (A or G), W (A or T), B (C, G, or T), V (A, C, or G), D (A, G, or T), N (A, C, G, or T)
After assembling the flanking sequences with the sequence of the cloned DNA fragment based on the conserved region, the full-length sequence of KmXYL3 was obtained. The sequence included a complete open reading frame (ORF) and was deposited in GenBank with accession no. GU586191. The DNA sequence of KmXYL3 was analyzed and aligned with other XK genes using BLAST.
KmXYL3 expression vector construction
The ORF of KmXYL3 was amplified from the genomic DNA of K. marxianus NBRC1777 by PCR using primers XK-EcoRI-F and XK-HindIII-R. The PCR products were then digested with EcoRI and HindIII and inserted into a pET-21c plasmid to construct the expression vector pET-21c-Kmxyl3.
Expression and purification of recombinant KmXK
The pET-21c-Kmxyl3 plasmid was transformed into E. coli BL21 (DE3) cells. After obtaining single colonies harboring the pET21c-Kmxyl3 plasmid, one colony was inoculated into 5 ml LB and incubated at 37°C overnight. Thereafter, 4 ml of the preculture was transferred into 400 ml LB medium in a 1-l Erlenmeyer flask and incubated at 37°C. Subsequently, 0.2 mM isopropyl β-d-1-thiogalactopyranoside (IPTG) (final concentration) was added when the OD600 was 0.4–0.8, and the incubation was continued for a further 12 h at 16°C. The cells were harvested by centrifugation at 4°C (10 min at 7,000 × g) and resuspended in 20 ml buffer A (50 mM Tris–HCl, 25 mM NaCl, pH 8.0). After the cells were lysed by sonication and centrifuged at 4°C (15 min at 15,000 × g), the supernatant was loaded onto a Ni–NTA affinity column (Qiagen, The Netherlands). The column was washed with buffer A, containing 5 mM imidazole, and the recombinant KmXK was then eluted using 250 mM imidazole. The recovered KmXK was purified further on a Sephacryl S200 column provided by AKTA UPC10. The purified protein was then analyzed by sodium dodecyl sulfate-polyacrylamide gel electrophoresis (SDS-PAGE).
d-Xylulokinase assay
d-Xylulokinase activity was measured as described by Shamanna and Sanderson [28] with some modifications. Xylulokinase activity was assayed in a 50 mM Tris–HCl (pH 7.5) buffered mixture containing glycine (50 mM), KCl (50 mM), ethylenediamine tetraacetic acid (EDTA) (1 mM), NaF (10 mM), phosphoenolpyruvate, tricyclohexylammonium (PEP-Tri) (1 mM), MgCl2 (5 mM), adenosine triphosphate (ATP) (0.5 mM), nicotinamide adenine dinucleotide (NADH) (0.3 mM), lactate dehydrogenase (LDH, E.C. 1.1.1.27, 25 U), pyruvate kinase (E.C. 2.7.1.40, 25 U), prepared xylulokinase (100 μl), and xylulose (1 mM), and adjusted to a volume of 500 μl with H2O. The reaction was initiated by adding 1 mM xylulose, and activity was determined by monitoring the decrease of absorbance at 340 nm using a spectrophotometer. NaF inhibited ATPase and NADH oxidase activities but did not affect the activity of d-xylulokinase. One unit of d-xylulokinase was defined as the amount of enzyme that phosphorylates 1 μmol d-xylulose/min. An NADH standard curve was obtained without xylulokinase. The protein concentration of the crude cell extract was measured using the method described by Bradford [8].
Disruption of KmXYL3 in K. marxianus
ScTRP1 was amplified from YEGAP [17] by PCR using TRP-EcoRI-F and TRP-EcoRI-R primers (Table 2) and digested with EcoRI. The pET-21c-Kmxyl3 plasmid was digested with MfeI to remove a 338-bp DNA fragment from the KmXYL3 gene and then ligated with the EcoRI-digested ScTRP1 fragment to construct a new plasmid, pET-21c-Kmxyl3-Trp. The KmXYL3 disruption cassette was amplified from pET-21c-Kmxyl3-Trp by PCR with primers XK-knock-F and XK-knock-R (Table 2), and transformed by electroporation into K. marxianus YHJ010 to disrupt the KmXYL3 gene. The KmXYL3 disruptants were selected on SD/-Trp dropout medium, and gene disruption was confirmed by PCR with primers XK-EcoRI-F and XK-RS2 (Table 2). The function of KmXYL3 was validated through carbon utilization on solid or liquid SD medium. KmXYL3-disrupted (YWRL001) and wild-type KmXYL3 strains (NBRC1777 and YHJ010) were inoculated into 5 ml YPD and cultured overnight at 37°C. The cells were then harvested and washed with sterilized water. Finally, the cells were inoculated into 50 ml liquid SD medium with xylose or xylitol as carbon source. The growth of these three strains was measured by monitoring OD600 beginning at 0.1.
Results and discussion
Cloning of the KmXYL3 gene from K. marxianus
A 1,968-bp sequence was obtained by assembling the sequence amplified by TAIL-PCR and the sequence amplified on the basis of a conserved region of XKs. The BLAST result showed that it contained a 1,809-bp ORF sequence encoding a 602-amino-acid protein. The putative molecular weight of the protein was estimated to be 68 kDa. The encoded protein was highly homologous to XKs from other microorganisms and belonged to the FGGY family of carbohydrate kinases. The alignment of cloned KmXK and XKs from other microorganisms is shown in Fig. 2. All five sequence motifs [phosphate 1 (residues 10–15), connect 1 (residues 281–287), phosphate 2 (residues 300–304), adenosine (residues 496–498), and connect 2 (residues 529–530)], which are conserved in the ATP-binding regions of the known members of the sugar kinases, actin, and hsp70 class [6], were found in the amino acid sequence of KmXK. In particular, Asp10, Thr13, Gly302, Thr303, Ser304, Gly496, and Gly497 were common to all sugar kinases. Among them, Asp10, Thr13, and Asp282 were catalytic residues and were conserved in the ATPase superfamily (Fig. 2) [10].
Expression and purification of recombinant KmXK in E. coli
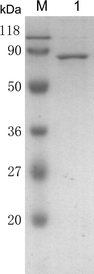
Purification of xylulokinase. 1 Purified xylulokinase, M prestained protein molecular weight marker (Fermentas)
Characterization of recombinant KmXK
KmXK showed enzyme activity with d-xylulose and d-fructose as substrates, and no activity with d-xylose. The specific activity of d-xylulose was 23.5 and 46.0 mU/mg with d-fructose.
Disruption of KmXYL3 in K. marxianus
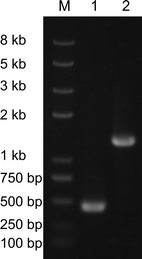
PCR to identify the disruption of KmXYL3. Lane 1 K. marxianus YHJ010, lane 2: K. marxianus YWRL001 (KmXYL3 disrupted)
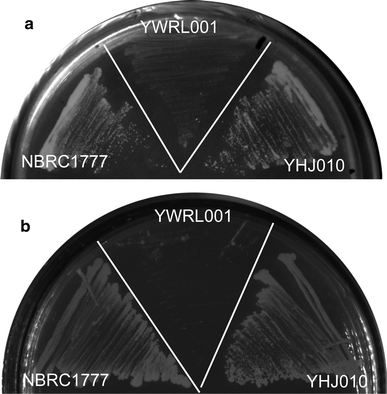
Growth of KmXYL3-disrupted strain K. marxianus YWRL001 on SD medium with xylose (a) or xylitol (b) as sole carbon source
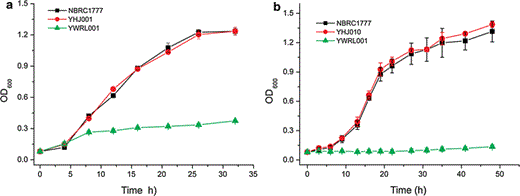
Growth of KmXYL3-disrupted strain YWRL001 in liquid SD medium with xylose (a) or xylitol (b) as sole carbon source
The disruption of KmXYL3 did not completely block the growth on plates containing xylose but did block growth on those containing xylitol. This indicates that there is an additional metabolic pathway, which can utilize xylose without xylulokinase. Jin et al. suggested a pathway from xylulose to arabinitol, which bypasses the step catalyzed by xylulokinase in P. stipitis [21]; however, in this study, the KmXYL3-disrupted strain was no longer able to utilize xylitol. Our other studies showed that a K. marxianus strain with disrupted xylose reductase (XR) gene KmXYL1 grew poorly in xylose medium, and a strain with the xylitol dehydrogenase (XDH) gene KmXYL2 disrupted was unable to utilize xylitol for growth, but showed weak growth in SD medium containing xylose (data not shown). All these results further proved the presence of a bypass pathway starting from xylose, not xylulose, as reported by Jin et al. By using the MRSD program (metabolic route search and design, http://www.bioinfo.ustc.edu.cn/softwares/mrsd/), a route was found which starts from d-xylose, then to d-xylonolactone, d-xylonate, 2-dehydro-3-deoxy-d-xylonate, and finally to pyruvate (Fig. 1). This route had previously been found in bacteria [25, 29], where the step from d-xylose to d-xylonolactone was catalyzed by d-xylose dehydrogenase (KEGG reaction K14273); d-xylonolactone was converted to d-xylonate by d-xylonolactonase (KEGG reaction K14274); d-xylonate was then converted to 2-dehydro-3-deoxy-d-xylonate by d-xylonate dehydratase (KEGG reaction K14275); and finally, 2-dehydro-3-deoxy-d-xylonate was converted to pyruvate and glycolaldehyde by 2-dehydro-3-deoxy-d-pentonate glycolaldehyde-lyase (KEGG reaction R01782) (Fig. 1) [29]. Although there is no known corresponding enzyme in yeast, several enzymes homologous to bacterial enzymes have been found in yeast. A peroxisomal multifunctional and oxidation protein encoded by the Fox2 gene in yeast is homologous to d-xylose dehydrogenase [15], and the gluconolactonase (YBR053C) in yeast [9, 20] is homologous to xylonolactonase. Dihydroxy-acid dehydratase gene (Ilv3p, GenBank accession no. EEU04815) in yeast is homologous to d-xylonate dehydratase [3]. Although these enzymes exist in yeast and are not specific for xylose metabolism, it is still possible that xylose was metabolized using these enzymes and resulted in the weak growth of this yeast.
Conclusions
A novel KmXYL3 gene encoding xylulokinase (KmXK) from K. marxianus was cloned. Gene disruption and recombinant enzyme activity both confirmed that KmXK catalyzes xylulose to xylulose 5-P in the xylose metabolic pathway. Disruption of KmXYL3 hindered assimilation of xylose and xylitol but could not block utilization of xylose completely. This suggests the presence of a bypass pathway starting from xylose. The cloning and disruption of the KmXYL3 gene provides a foundation for metabolic flux research on xylose and modification of the xylose pathway in K. marxianus in the future.
Acknowledgments
We thank Professor Tamaki Hisanori from Kagoshima University and Kumagai Hidehiko from Ishikawa Prefectural University for providing us K. marxianus YHJ010 and plasmid YEGAP. This work was supported by a grant-in-aid from the National Natural Science Foundation of China (grant no. 31070028), and the project was also sponsored by the Scientific Research Foundation for the Returned Overseas Chinese Scholars, State Education Ministry and Specialized Research Fund for the Doctoral Program of Higher Education of China (grant no. 20093402120027) and the Fundamental Research Funds for the Central Universities (grant no. WK2070000007).