-
PDF
- Split View
-
Views
-
Cite
Cite
Oksik Choi, Cheng-Zhu Wu, Sun Young Kang, Jong Seog Ahn, Tai-Boong Uhm, Young-Soo Hong, Biosynthesis of plant-specific phenylpropanoids by construction of an artificial biosynthetic pathway in Escherichia coli, Journal of Industrial Microbiology and Biotechnology, Volume 38, Issue 10, 1 October 2011, Pages 1657–1665, https://doi.org/10.1007/s10295-011-0954-3
- Share Icon Share
Abstract
Biological synthesis of plant secondary metabolites has attracted increasing attention due to their proven or assumed beneficial properties and health-promoting effects. Phenylpropanoids are the precursors to a range of important plant metabolites such as the secondary metabolites belonging to the flavonoid/stilbenoid class of compounds. In this study, engineered Escherichia coli containing artificial phenylpropanoid biosynthetic pathways utilizing tyrosine as the initial precursor were established for production of plant-specific metabolites such as ferulic acid, naringenin, and resveratrol. The construction of the artificial pathway utilized tyrosine ammonia lyase and 4-coumarate 3-hydroxylase from Saccharothrix espanaensis, cinnamate/4-coumarate:coenzyme A ligase from Streptomyces coelicolor, caffeic acid O-methyltransferase and chalcone synthase from Arabidopsis thaliana, and stilbene synthase from Arachis hypogaea.
Electronic supplementary material
The online version of this article (doi:10.1007/s10295-011-0954-3) contains supplementary material, which is available to authorized users.
Introduction
Phenylpropanoids include several important natural products classes, for example, flavonoids, stilbenoids, lignins, and coumarins. These phenylpropanoid-derived substances have a range of recorded effects on human health, involving antioxidant, antiallergic, antiinflammatory, antithrombotic, and antioncogenic activities [9, 22]. These phenylpropanoid biosynthetic enzymes are, therefore, attractive targets for metabolic engineering and synthetic biology to enhance or initiate the production of economically desirable traits or compounds [7, 10, 23]. Recent advances in the biochemistry of specific enzymes and enzyme complexes have created strategies for the enhancement of phenylpropanoid compounds by modifying pathways [15, 17, 20, 24, 27].
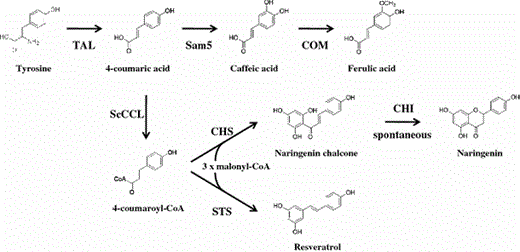
Engineered biosynthetic pathway in E. coli. TAL and Sam5 from S. espanaensis, COMT and CHS from A. thaliana, ScCCL from S. coelicolor, and STS from A. hypogaea
Our strategy to harness microorganisms for the production of naringenin and resveratrol was to design and express artificial phenylpropanoid pathways with bacterial tyrosine ammonia-lyase (TAL). This was accomplished by assembling TAL from the actinomycete Saccharothrix espanaensis, cinnamate/4-coumarate:CoA ligase (ScCCL) from the actinomycete Streptomyces coelicolor, CHS from the plant Arabidopsis thaliana, and STS from the plant Arachis hypogaea on a single plasmid in Escherichia coli. In addition, phenylpropioinic acids, such as 4-coumaric acid, caffeic acid, and ferulic acid, can be efficiently produced by recombinant E. coli cells expressing the actinomycete TAL, 4-coumarate 3-hydroxylase (Sam5), and plant caffeic acid O-methyltransferase (COMT) from A. thaliana.
Materials and methods
Strains, plasmids, and chemicals
E. coli DH5α and E. coli C41 (DE3) [19] were used for general DNA manipulation and expression of biosynthetic genes, respectively. A pCR®-TOPO vector (Invitrogen, Carlsbad, CA) was used for polymerase chain reaction (PCR) cloning. pET-28a(+) harboring the kanamycin resistance gene was purchased from Novagen (San Diego, CA). 4-Coumaric acid, cinnamic acid, caffeic acid, ferulic acid, naringenin, and resveratrol were purchased from Sigma–Aldrich (St. Louis, MO).
DNA manipulation
Restriction enzymes, Taq DNA polymerase, and other DNA-modifying enzymes were purchased from TaKaRa Biochemicals (Shiga, Japan). DNA ligation was performed using AccuPower® Ligation PreMix and AccuRapidTM DNA ligation kit (Bioneer, Korea). TAL and Sam5 genes from S. espanaensis KCTC9392, COMT (GenBank # AY062837) and CHS gene (GenBank # AF112086) from A. thaliana, and the ScCCL gene from S. coelicolor A3(2) M130 (GenBank # NP628552) were amplified from the genomic DNA or complementary DNA (cDNA) library using the described primer set [1, 3, 14, 25]. The STS gene from Arachis hypogaea (GenBank # AB027606) was codon-optimized and synthesized by Codon Devices (Cambridge, MA) [1, 3, 14, 25]. After the DNA manipulation, the absence of undesired alterations during PCR was checked by nucleotide sequencing on an automated nucleotide sequencer.
Construction of pET-T5 M
Primers used in this study
Name . | Sequence (5′–3′) . | Restriction enzyme site . |
---|---|---|
TAL-N | AGATCTACGCAGGTCGTGGAACGT | BglII |
TAL-C | AAGCTTGTGTGCTCATCCGAAATCCTT | HindIII |
Sam5-F | CATATGACCATCACGTCACCTGCGCCGG | NdeI |
Sam5-R | AAGCTTCAGGTGCCGGGGTTGATCAGGTCGG | HindIII |
COM-F | CATATGGGTTCAACGGCAGAGAC | NdeI |
COM-R | AAGCTTAGAGCTTCTTGAGTAACTC | HindIII |
CCL-F | CATATGTTCCGCAGCGAGTACGCA | NdeI |
CCL-R | AAGCTTCATCGCGGCTCCCTGAGC | HindIII |
CHS-N | AGATCTGGTGCTTCTTCTTTGGATGAG | NdeI |
CHS-C | CTCGAGTTAGAGAGCAACGCTGTG | XhoI |
kS-R | CTCGAGTTAAATAGCCATCGAGCGCAGGACC | XhoI |
CPac | TTAATTAATGCGCCGCTACAGGGCGCGTCC | PacI |
NPac | TTAATTAATCGCCGCGACAATTTGCGACGG | PacI |
Cspe | ACTAGTTCCTCCTTTCAGCAAAAAACCCCTC | SpeI |
Nspe | ACTAGTAGGTTGAGGCCGTTGAGCACCGCC | SpeI |
Name . | Sequence (5′–3′) . | Restriction enzyme site . |
---|---|---|
TAL-N | AGATCTACGCAGGTCGTGGAACGT | BglII |
TAL-C | AAGCTTGTGTGCTCATCCGAAATCCTT | HindIII |
Sam5-F | CATATGACCATCACGTCACCTGCGCCGG | NdeI |
Sam5-R | AAGCTTCAGGTGCCGGGGTTGATCAGGTCGG | HindIII |
COM-F | CATATGGGTTCAACGGCAGAGAC | NdeI |
COM-R | AAGCTTAGAGCTTCTTGAGTAACTC | HindIII |
CCL-F | CATATGTTCCGCAGCGAGTACGCA | NdeI |
CCL-R | AAGCTTCATCGCGGCTCCCTGAGC | HindIII |
CHS-N | AGATCTGGTGCTTCTTCTTTGGATGAG | NdeI |
CHS-C | CTCGAGTTAGAGAGCAACGCTGTG | XhoI |
kS-R | CTCGAGTTAAATAGCCATCGAGCGCAGGACC | XhoI |
CPac | TTAATTAATGCGCCGCTACAGGGCGCGTCC | PacI |
NPac | TTAATTAATCGCCGCGACAATTTGCGACGG | PacI |
Cspe | ACTAGTTCCTCCTTTCAGCAAAAAACCCCTC | SpeI |
Nspe | ACTAGTAGGTTGAGGCCGTTGAGCACCGCC | SpeI |
Primers used in this study
Name . | Sequence (5′–3′) . | Restriction enzyme site . |
---|---|---|
TAL-N | AGATCTACGCAGGTCGTGGAACGT | BglII |
TAL-C | AAGCTTGTGTGCTCATCCGAAATCCTT | HindIII |
Sam5-F | CATATGACCATCACGTCACCTGCGCCGG | NdeI |
Sam5-R | AAGCTTCAGGTGCCGGGGTTGATCAGGTCGG | HindIII |
COM-F | CATATGGGTTCAACGGCAGAGAC | NdeI |
COM-R | AAGCTTAGAGCTTCTTGAGTAACTC | HindIII |
CCL-F | CATATGTTCCGCAGCGAGTACGCA | NdeI |
CCL-R | AAGCTTCATCGCGGCTCCCTGAGC | HindIII |
CHS-N | AGATCTGGTGCTTCTTCTTTGGATGAG | NdeI |
CHS-C | CTCGAGTTAGAGAGCAACGCTGTG | XhoI |
kS-R | CTCGAGTTAAATAGCCATCGAGCGCAGGACC | XhoI |
CPac | TTAATTAATGCGCCGCTACAGGGCGCGTCC | PacI |
NPac | TTAATTAATCGCCGCGACAATTTGCGACGG | PacI |
Cspe | ACTAGTTCCTCCTTTCAGCAAAAAACCCCTC | SpeI |
Nspe | ACTAGTAGGTTGAGGCCGTTGAGCACCGCC | SpeI |
Name . | Sequence (5′–3′) . | Restriction enzyme site . |
---|---|---|
TAL-N | AGATCTACGCAGGTCGTGGAACGT | BglII |
TAL-C | AAGCTTGTGTGCTCATCCGAAATCCTT | HindIII |
Sam5-F | CATATGACCATCACGTCACCTGCGCCGG | NdeI |
Sam5-R | AAGCTTCAGGTGCCGGGGTTGATCAGGTCGG | HindIII |
COM-F | CATATGGGTTCAACGGCAGAGAC | NdeI |
COM-R | AAGCTTAGAGCTTCTTGAGTAACTC | HindIII |
CCL-F | CATATGTTCCGCAGCGAGTACGCA | NdeI |
CCL-R | AAGCTTCATCGCGGCTCCCTGAGC | HindIII |
CHS-N | AGATCTGGTGCTTCTTCTTTGGATGAG | NdeI |
CHS-C | CTCGAGTTAGAGAGCAACGCTGTG | XhoI |
kS-R | CTCGAGTTAAATAGCCATCGAGCGCAGGACC | XhoI |
CPac | TTAATTAATGCGCCGCTACAGGGCGCGTCC | PacI |
NPac | TTAATTAATCGCCGCGACAATTTGCGACGG | PacI |
Cspe | ACTAGTTCCTCCTTTCAGCAAAAAACCCCTC | SpeI |
Nspe | ACTAGTAGGTTGAGGCCGTTGAGCACCGCC | SpeI |
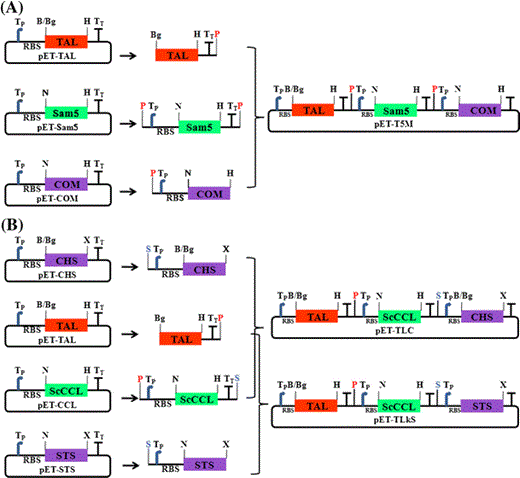
Organization of the artificial gene clusters used for production of plant-specific compounds in E. coli. All three genes contained their own T7 promoter and RBS. Schematic representation of the strategies used for construction of pET-T5 M (a), pET-TLC, and pET-TLkS (b). All constructs contained the T7 promoter and the RBS in front of each gene, and the T7 terminator located in the rear of each gene. The following abbreviations are used: B, BamHI; Bg, BglII; H, HindIII; N, NdeI; X, XhoI; P, PacI; S, SpeI; Tp, T7 promoter; TT, T7 terminator; RBS, ribosomal binding site
Construction of pET-TLC and pET-TLkS
Using S. coelicolor genomic DNA as a template, the ScCCL gene fragment was PCR-amplified with the primer pairs CCL-F and CCL-R (Table 1), and was cloned into pCR®-TOPO, resulting in pCR-CCL. To construct the expression plasmid, the NdeI-HindIII fragment was excised from pCR-CCL and cloned between the NdeI and HindIII sites of pET-28a(+), resulting in pET-CCL (Fig. 2). Using A. thaliana cDNA as a template, the CHS gene fragment was PCR-amplified with the primer pairs CHS-N and CHS-C (Table 1), and cloned into pCR®-TOPO, resulting in pCR-CHS. The BglII-XhoI fragment was excised from pCR-CHS and cloned between the BamHI and XhoI sites of pET-28a(+), resulting in pET-CHS (Fig. 2). To construct the plasmid containing the artificial naringenin biosynthetic pathway, the previous pET-T5 M cloning method was used. The 2.53-kb DNA fragment containing the ScCCL coding region was PCR-amplified using the pET-CCL as a template with primers NPac and CSpe (the sequence is located downstream of the T7 terminator region of the pET vector and contains the designed SpeI site; Table 1). Using pET-CHS as a template, the 1.63-kb DNA fragment containing the CHS coding region was PCR-amplified with primers NSpe (the sequence is located upstream of the T7 promoter region of the pET vector and contains the designed SpeI site; Table 1) and CHS-C. The amplified fragments were digested with each restriction enzymes and cloned between BamHI- and XhoI-digested pET-28a(+) by a four-fragment ligation, resulting in pET-TLC (Fig. 2). The gap between the previous terminator and the next promoter is 696 bp (TAL-CCL) and 269 bp (CCL-CHS), respectively.
In addition, the STS gene of A. hypogaea was synthesized and cloned into pUC19. A NdeI-XhoI fragment of STS (1-kb) was cloned between the corresponding sites of pET-28a(+), resulting in pET-STS (Fig. 2). To construct the plasmid containing the resveratrol biosynthetic pathway, we used the previous cloning method using the PacI and SpeI sites. Using pET-STS as a template, the 1.58-kb DNA fragment containing the STS coding region was PCR-amplified with primers NSpe and KS-R. The amplified fragments were digested with each restriction enzyme and cloned between BamHI- and XhoI-digested pET-28a(+) by a four-fragment ligation, resulting in pET-TLkS (Fig. 2).
Culture conditions for production
Recombinant E. coli C41 (DE3) strains harboring plasmid were precultured overnight at 37°C in Luria–Bertani (LB) medium containing 50 μg/mL kanamycin. The overnight culture was inoculated (1.5%) into fresh LB medium supplemented with the same concentration of kanamycin. The culture was grown at 37°C to optical density at 600 nm (OD600) of 0.6. Isopropyl β-d-thiogalactopyranoside (IPTG) was added at final concentration of 1 mM, and the culture was incubated for 5 h. The cells were harvested by centrifugation, suspended, and incubated at 26°C for 36 h in modified M9 medium (M9 medium supplemented with 40 g/L glucose and 25 g/L CaCO3, pH 6.8–7.0) containing 50 μg/mL kanamycin and 1 mM IPTG [21]. At the end of the incubation, the broth cultures had a pH ranging from 5.8 to 6.0. For feeding experiments, cultures were induced with IPTG, supplemented with 4-coumaric acid, cinnamic acid, caffeic acid, and ferulic acid (final concentration, 2 mM), and allowed to grow for an additional 36 h before harvest.
Extraction and analysis of product
Twenty-five milliliters of culture was extracted with an equal volume of ethyl acetate. In particular, the E. coli broth cultures containing pET-TLC plasmid were adjusted to pH 9.0 with NaOH [11]. The ethyl acetate was dried under vacuum, and the dried residue was resuspended in 500 μL methanol. Twenty microliters of extract was applied to a J’sphere ODS-H80 column (4.6 × 150 mm i.d., 5 μm; YMC, Kyoto, Japan) using a high-pressure liquid chromatography (HPLC) system [CH3CN–H2O (0.05% trifluoroacetic acid) 20:80 to 60:40 over 25 min followed to 100:0 by 1 min, 1 mL/min; Dionex, Sunnyvale, CA, USA] equipped with a photodiode array detector. The retention times of naringenin and resveratrol were 14.6 and 10.5 min, respectively. Compounds were identified by comparing observed retention time, ultraviolet spectra, and mass chromatogram with those of authentic standards. Liquid chromatography-mass spectrometry (LC–MS) was performed using a Finnigan LCQ mass spectrophotometer (Thermo Electron, Pittsburgh, PA, USA) equipped with an electrospray ionization (ESI) source. HPLC separations were performed using a Finnigan Surveyor HPLC System unit (Thermo Electron) using a hydrosphere C18 column (50 × 2.0 mm, 5 μm; YMC, Kyoto, Japan). The system was operated using a previously described procedure with minor modification [16]. Data-dependent tandem mass spectrometry experiments were controlled using menu-driven software provided with the Xcalibur system. Single-charged radicals for standard compounds were 4-coumaric acid (m/z 163.1), caffeic acid (m/z 179.1), ferulic acid (m/z 193.1), naringenin (m/z 271.2), and resveratrol (m/z 227.2).
Results and discussion
Production of 4-coumaric acid, caffeic acid, and ferulic acid by recombinant E. coli
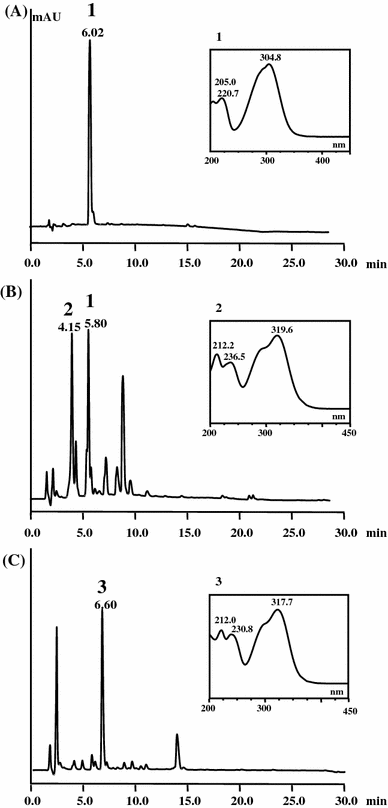
HPLC analysis of extracts from supernatants of cultures containing E. coli cells expressing TAL alone or together with Sam5 and COM. The cells were cultured in modified M9 medium and analyzed after 24 h induction. a E. coli harboring pET-TAL plasmid. b E. coli harboring pET-Sam5 fed with 4-coumaric acid. c E. coli harboring pET-T5 M coexpressing TAL, Sam5, and COM proteins. Absorbance was monitored at 285 nm. The insets show the UV/Vis spectra of the compounds with the indicated HPLC peaks. Peaks indicate 4-coumaric acid (1), caffeic acid (2), and ferulic acid (3)
Production of naringenin in E. coli through an artificial biosynthetic pathway
Naringenin is a gateway compound for a large number of flavonoid-derived plant natural products [9]. To produce naringenin in E. coli, an artificial gene cluster containing three genes of heterologous origins (TAL from S. espanaensis, ScCCL from S. coelicolor, and CHS from A. thaliana) was constructed. The use of the bacterial TAL enzyme would bypass the hydroxylation step of C4H for the production of naringenin chalcone from phenylalanine. ScCCL in the Gram-positive filamentous bacterium S. coelicolor A3(2) can activate 4-coumaric acid to 4-coumaroyl-CoA, as well as cinnamic acid to cinnamoyl-CoA [14]. CHS is a well-studied ubiquitous plant-specific type III polyketide synthase that catalyzes sequential decarboxylative condensation of 4-coumaroyl-CoA with three molecules of malonyl-CoA to produce a new aromatic ring system, naringenin chalcone, the key intermediate in the biosynthesis of flavonoids [1]. Naringenin chalcone was thought to be rapidly converted into naringenin by nonenzymatic isomerization under alkaline conditions. We cloned the CHS gene from A. thaliana, and the obtained sequence was not consistent with the published sequences from A. thaliana (GenBank # AF112086). However, these nucleotide changes caused silent mutations in the amino acid sequence. Expression of recombinant CHS was determined by sodium dodecyl sulfate–polyacrylamide gel electrophoresis (SDS–PAGE). The purified protein displayed the expected molecular weight of near 46.2 kDa (Supplementary Fig. 1). We designed an artificial gene cluster in the pET-TLC plasmid so that tyrosine was converted into naringenin chalcone by the successive actions of TAL, ScCCL, and CHS (Fig. 2). The pET-TLC plasmid consisting of a T7 promoter and RBS upstream of each gene produced the expected efficient transcription and translation of the three genes in E. coli [13]. The recombination cells were cultured at 26°C to avoid formation of inclusion bodies of the proteins, since SDS–PAGE analysis of the total proteins showed that, when grown at 37°C, a large amount of 46.2-kDa CHS was evident in both the insoluble and soluble fractions (Supplementary Fig. 1). Even when culturing at 26°C, a considerable amount of the 46.2-kDa CHS was still recovered in the insoluble fraction. On the other hand, no SDS–PAGE-separated proteins with molecular masses of 57.4 and 57.5 kDa, representing TAL and ScCCL, respectively, were detected (Supplementary Fig. 1).
![HPLC and LC–MS analysis of naringenin produced by engineered E. coli harboring plasmid pET-TLC. a HPLC analysis of extracts from E. coli harboring pET-TLC cultures produced naringenin. The insets show the UV/Vis spectra of the naringenin peak. Peaks indicate 4-coumaric acid (1) and naringenin acid (2). b Selected ion chromatogram of naringenin (m/z 271.26 [M-H]−) produced by E. coli harboring pET-TLC. c Selected ion chromatogram of pinocembrin at m/z 255.29 [M-H]− produced by E. coli harboring pET-TLC. See “Materials and methods” for details of the HPLC and LC–MS protocols](https://oup.silverchair-cdn.com/oup/backfile/Content_public/Journal/jimb/38/10/10.1007_s10295-011-0954-3/2/m_10295_2011_954_fig4_html.gif?Expires=1750230303&Signature=fo2kcHKYruzoRlQQe~w9w7fj~51IAzetK8Jk-aLwYQ8ZnsndpXQn1eWtDc88TLcu-Lo8jc05JAJVhZ9afjsYf~9AWmIsXtZ7PgSQcPr8PoyBii9Up8xv6sp0fDdtQNMlJsadfuVnRGXDOKtW3IFXjZpD2yICDo~QslpuZHepx2t1rv04WZZfl3u7tW9rP6GL7CKcsaS89HFxNP9L6miGsL~Xr5qtiNHfvA10TjnPjvf5u1vfs~hpoPfLS56A7OXbkuBdP4Bb1FgxG~6qGD2wiSwt5-tXkNKzAO0RbYolzlXTW1iGzmEGTQPALqSeFSjQ7PR2NmtzsgiCzcyQ5Rp7oQ__&Key-Pair-Id=APKAIE5G5CRDK6RD3PGA)
HPLC and LC–MS analysis of naringenin produced by engineered E. coli harboring plasmid pET-TLC. a HPLC analysis of extracts from E. coli harboring pET-TLC cultures produced naringenin. The insets show the UV/Vis spectra of the naringenin peak. Peaks indicate 4-coumaric acid (1) and naringenin acid (2). b Selected ion chromatogram of naringenin (m/z 271.26 [M-H]−) produced by E. coli harboring pET-TLC. c Selected ion chromatogram of pinocembrin at m/z 255.29 [M-H]− produced by E. coli harboring pET-TLC. See “Materials and methods” for details of the HPLC and LC–MS protocols
Production of resveratrol in E. coli through an artificial biosynthetic pathway
Resveratrol, a representative stilbene found in grapes and other food products, has attracted increasing interest due to its inhibitory activity, both in vitro and in vivo, of the initiation, promotion, and progression of carcinogenesis [12]. Several attempts to produce resveratrol by recombinant microorganisms have been reported [2, 25]. Stilbenes are biosynthesized from tyrosine, which are converted to 4-coumaric acid. This acid is then activated to CoA esters, which becomes the substrate of STS, and condenses with three molecules of malonyl-CoA, resulting in resveratrol (Fig. 1).
![HPLC and LC–MS analysis of resveratrol produced by engineered E. coli harboring pET-TLkS vector. a HPLC analysis of extracts from E. coli harboring pET-TLkS cultures produced resveratrol. E. coli harboring pET28 (empty vector, negative control) did not produce resveratrol. The peak at 6 min represents 4-coumaric acid, and the peak at 10.5 min represents resveratrol. b Selected ion chromatogram of resveratrol (m/z 227.23 [M-H]−) produced by E. coli harboring pET-TLkS](https://oup.silverchair-cdn.com/oup/backfile/Content_public/Journal/jimb/38/10/10.1007_s10295-011-0954-3/2/m_10295_2011_954_fig5_html.gif?Expires=1750230303&Signature=t6V~IRiWo4NgFAw2QEgRhVr-NGqm-rQjia1gjWYva1X60~Ix3XxCz1P0oCNI7TsV~Yj3K77Nt5lmDK6o69un6wWrPaE4BmOioWyNi8qBnFGAOIN81EpUBHyfkQo6s7uCY7BoE2oA-05N1xKP8pX28ohQTp-cbYYJkWrwcD70l6H17L6GiShs0Km4ecypcQKCin7tKjJRplrRb~2qNPwUPfYQ~tgbal3jPFg95PZSS8biPQtQwKGLDBskv7b1QoG-OW6iKUMqSsh3ifLyKXyb77bMDkkMlbNhJa3tMJcvQE4XjWSjUlfoSwhSHot~O5o40kZekTN0fgrNYMnN1~tL0Q__&Key-Pair-Id=APKAIE5G5CRDK6RD3PGA)
HPLC and LC–MS analysis of resveratrol produced by engineered E. coli harboring pET-TLkS vector. a HPLC analysis of extracts from E. coli harboring pET-TLkS cultures produced resveratrol. E. coli harboring pET28 (empty vector, negative control) did not produce resveratrol. The peak at 6 min represents 4-coumaric acid, and the peak at 10.5 min represents resveratrol. b Selected ion chromatogram of resveratrol (m/z 227.23 [M-H]−) produced by E. coli harboring pET-TLkS
We have successfully expressed all the biosynthetic genes and engineered the artificial pathway leading to the synthesis of the phenylpropionic acids, naringenin, and resveratrol in E. coli, starting from tyrosine. The production of ferulic acid, naringenin, and resveratrol yielded 7.1 ± 1.3 mg/L, 5.3 ± 1.3 mg/L, and 1.4 ± 0.2 mg/L, respectively. It is important to note, however, that the observed phenylpropanoids production was a basal level from the synthetic gene cluster without any metabolic engineering and/or precursor feeding in the fermentation process. However, the yields of phenylpropanoids were very low. We expect that an increase in the amount of malonyl-CoA in E. coli under normal culture conditions by overexpression of the acetyl-CoA carboxylase gene would lead to yield enhancement [4, 17, 18]. In addition, use of a tyrosine-fermenting strains would obviate the need to provide amino acids to the culture and elevate the yields of the final product.
To achieve efficient artificial biosynthetic platforms of phenylpropanoids in general, optimizing the functional expression of the biosynthetic enzymes used during the biosynthetic process is a concern. Many of the early studies resorted to more practical approaches of protein expression to improve the effective intercellular concentrations of the functional enzymes in an effort to increase production levels. In this approach, metabolic channeling typically involves the organization of intracellular enzymes so that active sites for consecutive reactions are brought into close proximity. This proximity prevents loss of intermediates by either diffusion or competition with other pathways, and can effectively increase catalytic efficiency by up to two orders of magnitude. In one study, use of synthetic protein scaffolds recruited metabolic enzymes to an extent that markedly improved the production of mevalonate while lowering the overall cellular metabolic load [6]. Based on an understanding of such organization, we also will try new strategies for efficiently assembling metabolic enzymes into structures that yield high product titers.
Acknowledgments
This work was supported in part by the 21C Frontier Microbial Genomics and Application Center, Basic Science Research Program, and Global R&D Center program, the Ministry of Science and Technology, Republic of Korea, and by a grant from KRIBB Research Initiative Program. The authors would also like to thank Joon-Tae Park for assistance in the LC–MS experiment.
References
Electronic supplementary material
Supplementary material 1 (doc 27 kb)
Supplementary material 2 (pptx 603 kb)