-
PDF
- Split View
-
Views
-
Cite
Cite
M Kolari, J Nuutinen, F A Rainey, M S Salkinoja-Salonen, Colored moderately thermophilic bacteria in paper-machine biofilms, Journal of Industrial Microbiology and Biotechnology, Volume 30, Issue 4, 1 April 2003, Pages 225–238, https://doi.org/10.1007/s10295-003-0047-z
- Share Icon Share
Abstract
Biofilms cause several problems in papermaking. This report describes a microbiological survey of colored biofilms in six paper and board machines, including two case studies of outbreaks of colored slimes in which the causative bacteria were found. A total of 95 pink-, red-, orange- or yellow-pigmented strains were isolated. Nearly all (99%) of the strains grew at 52 °C, 72% grew at 56 °C, but only 30% grew at 28 °C, indicating that most of the strains were moderately thermophilic. Biofilm formation potential and biocide susceptibility of the strains were analyzed with a microtiter plate assay. In the presence of 5 ppm of methylene bisthiocyanate or 2,2-dibromo-3-nitrilopropionamide in paper-machine water, 55 strains formed biofims. Moreover, 39 strains increased biofilm production by 5–753% in the presence of biocide, suggesting that biocide concentrations inhibitory to planktonic but not to surface-attached cells may actually promote biofouling. The cells may have inactivated a portion of the biocides, as the cell density in this assay was high, corresponding to the highest cell densities occurring in the circulating waters. Four groups of colored bacteria that were isolated from several mills were identified. Pink-pigmented Deinococcus geothermalis and red-pigmented Meiothermus silvanus occurred as common primary biofilm-formers in paper machines. This report is the first description of the involvement of Meiothermus species in red-slime formation in the paper industry. The third group of bacteria (putative new species related to Roseomonas) contained strains that were not biofilm formers, but which were commonly found in slimes of neutral or alkaline machines. The fourth group contained red-pigmented biofilm-forming strains representing a novel genus of α-Proteobacteria related to Rhodobacter. Many colored paper-machine bacteria are species previously known from microbial mats of hot springs. Some characteristics of the bacterial groups are described here in order to facilitate their recognition in future cases of colored-slime outbreaks in the paper industry.
Introduction
Biofouling of surfaces in the wet end of paper machines can cause several problems for the papermaking process [3]. Slimes (biofilms) detaching from machine surfaces can break the paper web, causing downtime that can cost up to $30,000 per hour in modern machines. The content of live microbes in the paper product may increase to numbers intolerable in food-quality packaging paper and paperboard. Colored slimes can spoil paper products, as colored deposits are visible in white products. Biofouling can also accelerate corrosion of machine surfaces [3]. In spite of the use of biocides to control microbes in the papermaking process [3, 15], sporadic outbreaks of slimes occur. Biofouling is difficult to eradicate, as paper machines are open, large systems that cannot be kept sterile. Furthermore, biocides are less effective against sessile biofilms than against freely floating microbes [6, 7, 29]. Mattila et al. [15] examined biofilms grown in the shower water line of a paper machine, and showed by viability staining that biocide killed only the outermost biofilm layers.
Paper mills monitor total microbe amounts in raw materials, circulating waters, storage tanks, broke, and the paper products by regular counting of viable microbes. A wide range of bacterial species has been isolated from paper machines [3, 4, 5, 9, 12, 17, 18, 20, 26, 27], whereas in the paper products the major contaminants belong to three species of spore-forming bacteria: Bacillus, Brevibacillus and Paenibacillus [19, 20, 25, 27]. Slimes on the machine surfaces are more occasionally inspected, even though the surfaces can carry marked amounts of biomass. Biofilms with over 7×106 CFU cm−2 can grow in white water within 1 day [12]. Many paper-machine isolates produce slime in laboratory media [3, 5, 9, 26], but little is known about the species actually initiating the biofouling of the machines [3, 12, 27]. Concerning colored biofilms, attempts to identify the causative agents of pink slimes were made in the mid-1900s but, as stated previously [17], since then there have been significant changes in paper-making technology. Recently, Oppong et al. [17] described pink-pigmented isolates of Deinococcus grandis, Flectobacillus sp., Micrococcus sp., Methylobacterium zatmanii and Roseomonas sp. from colored slimes, but the ability of these bacteria to generate biofilms was not studied. Väisänen et al. [27] thoroughly examined the microbial communities in one printing-paper machine operating at pH 5. They isolated pigmented bacteria including pink strains of Acinetobacter radioresistens and Deinococcus geothermalis and yellow Aureobacterium testaceum from slimes; pink Methylobacterium mesophilicum from kaolin; yellow Ralstonia sp. from circulating water; and yellow Pantoea agglomerans from starch slurry. Only D. geothermalis formed biofilms in pure culture. Indeed, D. geothermalis is the only colored species described so far that is capable of primary biofilm formation in modern paper machines [12, 27].
This study focuses on colored slimes in the paper industry. We examined colored deposits occurring on stainless-steel surfaces in six mills. A total of 95 colored bacterial strains were isolated, characterized, and grouped, and their relevance for the papermaking process was investigated by testing their potential for biofilm formation and tolerance of two biocides. In addition, the causative bacteria of intensive formation of colored slimes in two mills are described.
Materials and methods
Isolation of colored bacteria from paper machines
The sources of the bacterial strains are shown in Table 1. Biofilm strains were isolated from sterilized stainless-steel test coupons immersed for 4–13 days in the process lines, or from slimes scraped off the machine chest. Surface temperatures of the sampling sites were measured with a portable Raynger ST (Raytek, Santa Cruz, Calif., USA) non-contact infrared thermometer with laser sighting. To enhance the yield of biofilm formers, the slime samples were washed before plating. Each sample was suspended in 10–50 ml sterilized tap water and vigorously shaken for 1 min. The slime was allowed to settle, the upper phase discarded, and the procedure repeated two to five times. Strains were isolated from the remaining, compact pellets of slime at 45 or 50 °C (2–7 days) on agar plates adjusted to pH 5, 7 or 8, reflecting the pH of the source machine. The following media were used: R2A agar (BBL; Becton Dickinson, Sparks, Md., USA), tryptic soy agar (TSA; Becton Dickinson), Castenholz trypticase yeast extract agar (CTYE; prepared as described in [1]), white water agar (WWA; [12]), clear filtrate agar (CFA; clear filtrate amended with yeast extract 0.1 g l−1, starch 1 g l−1, agar 15 g l−1), and pulp agar (PA; chemical pulp 2 g l−1, yeast extract 1 g l−1, peptone 0.5 g l−1, agar 15 g l−1). Strain codes (e.g. D-wire-R2A5-4) display information on the isolation source (wire section of machine D), the isolation medium (R2A pH 5), and the strain (4). D. geothermalis E50051 was described previously [27]. Reference strain Roseomonas gilardii ATCC49956T [22] was a gift from Victor L. Yu (University of Pittsburgh, Penn., USA). Meiothermus silvanus DSM9946T, M. ruber DSM1279T and Porphyrobacter neustonensis DSM9434T were obtained from the Deutsche Sammlung von Mikroorganismen und Zellkulturen, Braunschweig, Germany.
Origins of the paper-machine slimes and other samples. CP Chemical pulp, PGW pressurized groundwood, TMP thermomechanical pulp, CTMP chemithermomechanical pulp
Mill . | Machine type . | Wet-end temperature and pH . | Raw materials . | Main product . | Samples . |
---|---|---|---|---|---|
A | Fine paper machine | 45 °C, pH 8 | CP | Uncoated printing paper | Biofilms grown on stainless steel in a side-stream biofilm device (volume: 1 m3) fed with clear filtrate (conductivity 50 mS m−1) from machine A; CP; starch; fillers; chemically cleaned water; retention and sizing agents; other additives |
B | Fine paper machine | 53 °C, pH 5 | CP | Coated paper | Red slimes from the wire section; CP; raw water; retention and sizing agents; other additives |
C | Pulp dryer | 52–62 °C, pH 5.5 | CP | Dried sheets of CP | Slimes with various colors from wire section, water lines and wells; virgin pulp; talcum; cooling water; circulating water; machine felts; dried pulp sheets |
D | Newsprint machine | 52 °C, pH 5.3 | PGW+TMP+CP | Uncoated newsprint | Red slimes from the wire section |
E | Magazine paper machine | 52 °C, pH 7.4 | PGW+CP | Coated magazine paper | Slimes with various colors from the wire section, beginning of the press section, the disk-filter unit and the circulation water lines |
F | Board machine | 52 °C, pH 9.5 | CP+CTMP | Food-quality packaging board | Red slimes from splash areas in the wire section |
Mill . | Machine type . | Wet-end temperature and pH . | Raw materials . | Main product . | Samples . |
---|---|---|---|---|---|
A | Fine paper machine | 45 °C, pH 8 | CP | Uncoated printing paper | Biofilms grown on stainless steel in a side-stream biofilm device (volume: 1 m3) fed with clear filtrate (conductivity 50 mS m−1) from machine A; CP; starch; fillers; chemically cleaned water; retention and sizing agents; other additives |
B | Fine paper machine | 53 °C, pH 5 | CP | Coated paper | Red slimes from the wire section; CP; raw water; retention and sizing agents; other additives |
C | Pulp dryer | 52–62 °C, pH 5.5 | CP | Dried sheets of CP | Slimes with various colors from wire section, water lines and wells; virgin pulp; talcum; cooling water; circulating water; machine felts; dried pulp sheets |
D | Newsprint machine | 52 °C, pH 5.3 | PGW+TMP+CP | Uncoated newsprint | Red slimes from the wire section |
E | Magazine paper machine | 52 °C, pH 7.4 | PGW+CP | Coated magazine paper | Slimes with various colors from the wire section, beginning of the press section, the disk-filter unit and the circulation water lines |
F | Board machine | 52 °C, pH 9.5 | CP+CTMP | Food-quality packaging board | Red slimes from splash areas in the wire section |
Origins of the paper-machine slimes and other samples. CP Chemical pulp, PGW pressurized groundwood, TMP thermomechanical pulp, CTMP chemithermomechanical pulp
Mill . | Machine type . | Wet-end temperature and pH . | Raw materials . | Main product . | Samples . |
---|---|---|---|---|---|
A | Fine paper machine | 45 °C, pH 8 | CP | Uncoated printing paper | Biofilms grown on stainless steel in a side-stream biofilm device (volume: 1 m3) fed with clear filtrate (conductivity 50 mS m−1) from machine A; CP; starch; fillers; chemically cleaned water; retention and sizing agents; other additives |
B | Fine paper machine | 53 °C, pH 5 | CP | Coated paper | Red slimes from the wire section; CP; raw water; retention and sizing agents; other additives |
C | Pulp dryer | 52–62 °C, pH 5.5 | CP | Dried sheets of CP | Slimes with various colors from wire section, water lines and wells; virgin pulp; talcum; cooling water; circulating water; machine felts; dried pulp sheets |
D | Newsprint machine | 52 °C, pH 5.3 | PGW+TMP+CP | Uncoated newsprint | Red slimes from the wire section |
E | Magazine paper machine | 52 °C, pH 7.4 | PGW+CP | Coated magazine paper | Slimes with various colors from the wire section, beginning of the press section, the disk-filter unit and the circulation water lines |
F | Board machine | 52 °C, pH 9.5 | CP+CTMP | Food-quality packaging board | Red slimes from splash areas in the wire section |
Mill . | Machine type . | Wet-end temperature and pH . | Raw materials . | Main product . | Samples . |
---|---|---|---|---|---|
A | Fine paper machine | 45 °C, pH 8 | CP | Uncoated printing paper | Biofilms grown on stainless steel in a side-stream biofilm device (volume: 1 m3) fed with clear filtrate (conductivity 50 mS m−1) from machine A; CP; starch; fillers; chemically cleaned water; retention and sizing agents; other additives |
B | Fine paper machine | 53 °C, pH 5 | CP | Coated paper | Red slimes from the wire section; CP; raw water; retention and sizing agents; other additives |
C | Pulp dryer | 52–62 °C, pH 5.5 | CP | Dried sheets of CP | Slimes with various colors from wire section, water lines and wells; virgin pulp; talcum; cooling water; circulating water; machine felts; dried pulp sheets |
D | Newsprint machine | 52 °C, pH 5.3 | PGW+TMP+CP | Uncoated newsprint | Red slimes from the wire section |
E | Magazine paper machine | 52 °C, pH 7.4 | PGW+CP | Coated magazine paper | Slimes with various colors from the wire section, beginning of the press section, the disk-filter unit and the circulation water lines |
F | Board machine | 52 °C, pH 9.5 | CP+CTMP | Food-quality packaging board | Red slimes from splash areas in the wire section |
Microscopic analysis of slimes
Unstained slimes were examined using a stereomicroscope (Wild M10; Leica, Wetzlar, Germany) and photographed using color print films of 800 ASA. Slimes were stained with ethidium bromide (EtBr; 40 mg l−1; Sigma E-1510) in tap water for 15 min with shaking. Unbound stain was removed by repeated washing as described above. Some of the slimes were also stained with green fluorescent latex beads as described previously [11]. Epifluorescence microscopy (excitation filter BP450–490 nm; emission filter LP520 nm) and confocal laser scanning microscopy (CLSM; excitation at 488 nm and detection with emission filters BP506–538 and EFLP 585 nm) were done as described previously [12].
Phenotypic characterization of strains
Whole-cell fatty acids were analyzed in cultures grown for 24 or 48 h (for slow-growing strains 3–5 days) on R2A plates at 45 °C, or on trypticase soy broth agar (TSBA; Becton Dickinson) plates at 28 or 45 °C (some non-pigmented strains of mill A only). Fatty acids were extracted, methylated, analyzed and compared to the fatty-acid library (version 3.9) of the MIDI Microbial Identification System (Microbial ID, Newark, Del., USA) as described previously [26]. Maximum growth temperatures (T max) were determined by incubating the strains on R2A or CTYE plates in a biological incubator (Stuart Scientific S101D, Bibby Sterilin, Staffordshire, UK) with gradual temperature increments in steps of 3 °C. Each strain was grown for 2 days, after which growth was assessed and a new agar plate inoculated prior the next temperature increment. The actual temperature inside the incubator was recorded with an accuracy of ±0.2 °C by a Tinytag model G Temperature Datalogger (Gemini Data Loggers, Chichester, UK). Gram reaction and catalase activity were measured as described by Smibert and Krieg [23] on plate cultures grown for 2 days at 45 °C. Hydrolysis of starch, casein and lipids (Tween 80, oleic acid ester) was tested by plate assays [23]. Detection of the hydrolysis of Tween 80, yielding crystals of calcium soaps visible as opaque halo forms around the colonies, was aided by amending the media with 30 mg l−1 of Victoria Blue B as a contrasting stain. A dendrogram based on these phenotypic data was generated using BioNumerics version 2.0 (neighbor-joining tree-building method, Pearson's correlation, standardized characters) (Applied Maths, Kortrijk, Belgium).
For analysis of bacteriochlorophyll a, cultures were grown aerobically in the dark in PE medium [8] for 3 days. Cells were collected by centrifugation and extracted with acetone:methanol (3:1). The 400–900 nm absorption spectra of the extracts were recorded with a Perkin-Elmer Lambda Bio spectrometer. An extract of P. neustonensis DSM9434T served as a positive reference.
16S rRNA gene sequence analysis
The partial and nearly full (~450 and ~1,450 nucleotides) 16S rRNA gene sequences were determined by direct sequencing of PCR-amplified 16S rDNA as described previously [21]. The sequence data were compared with those of prokaryotic 16S rDNA sequences in the EMBL [24], EMBL New (release 11 September, 2002) and RDP databases [14]. Similarity values were calculated by pairwise comparison of the aligned sequences.
Microtiter plate assay for biofilm formation
Biofilm formation was assayed in 96-well plates of tissue-culture-treated polystyrene (NunclonT 167008; Nalge Nunc International, Roskilde, Denmark). Three media were used: R2-broth (pH 7) and paper-machine circulating water (white water, WW) medium at pH 5 and at pH 7. R2-broth contained casein peptone 0.75 g l−1, soluble starch 0.5 g l−1, yeast extract 0.5 g l−1, glucose 0.5 g l−1, K2HPO4 0.3 g l−1, sodium pyruvate 0.3 g l−1, meat extract 0.25 g l−1 and MgSO4 0.024 g l−1. To prepare WW media, white water (conductivity 100–120 mS m−1) was collected from publication paper machines operating at pH 5 and pH 7, amended with 200 mg yeast extract l−1 and 1.5 g soluble starch l−1, filtered through a metal sieve with a pore size of 74 µm (to remove fibers but not process fines and fillers), sterilized (121 °C, 15 min) and adjusted to pH 4.9 and pH 7.2 with 2 M HCl.
In the BF assay, each of the microtiter plate wells was filled with 250 µl sterile medium and 0, 5 or 15 ppm (concentration of effective substances) methylene bisthiocyanate (MBT; Fennosan M9) or 2,2-dibromo-3-nitrilopropionamide (DBNPA; synonym 2,2-dibromo-2-cyanoacetamide; Fennosan R20 V; Kemira Chemicals, Finland). Each well was inoculated with 10 µl of a culture grown in R2-broth for 2 days at 45 °C. The plates were placed on a rotary shaker (160 rpm, 45 °C) for 2 days. Planktonic growth in the wells was visually scored based on the turbidity and color of the wells. The wells were emptied, stained with 330 µl crystal violet (4 g l−1 in 20% (v/v) methanol) for 3 min, washed three times with running water to remove planktonic cells, and allowed to air-dry. Stain retained by the biofilm was dissolved in ethanol (330 µl per well, 1 h) and A 595 measured with an iEMS Plate Reader (Labsystems, Helsinki, Finland). Between 0 and 4.0 the A 595 values correlate linearly with CFU in the biofilm [12]. The threshold for evident biofilm formation was set to an A 595 value of 0.3 corresponding to ca. 4×104 CFU cm−2 [12]. The significance of differences was calculated with Student's t test.
Strain and nucleic acid sequence accession numbers
The strains M. silvanus B-R2A5-50-4, M. silvanus C-wire-CTYE-1, unknown α-Proteobacteria A-col-CFA-6, D. geothermalis C-wire-CTYE-3 and D. geothermalis C-CTYE-9a were deposited in HAMBI (the Culture Collection of the Faculty of Agriculture and Forestry, University of Helsinki) with the HAMBI numbers 2477, 2478, 2479, 2480 and 2481. The 16S rDNA sequences of the α-Proteobacteria A-col-CFA-6, A-col-R2A-4 and A-cf-CFA-6 were submitted to EMBL and assigned accession numbers AJ505839, AJ505840 and AJ512828.
Results
Characterization of colored biofilms in paper machine A
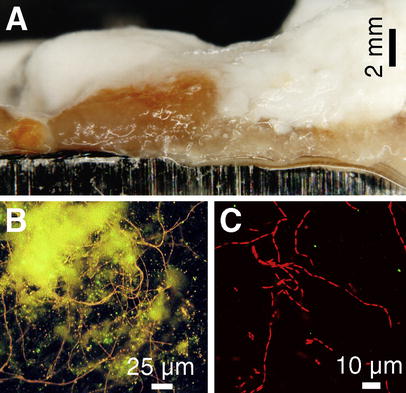
Photomicrographs of biofilms grown on stainless-steel test coupons within 17 days of immersion in clear filtrate of paper machine A (Table 1). A Stereomicroscopy of the unstained biofilm revealed a layered structure with an orange-colored, slimy, cohesive layer closest to the steel surface. Epifluorescence photomicrographs from the steel surface after scraping off most of the orange biofilm layer. B A network of adherent filamentous bacteria (stained with the nucleic acid stain ethidium bromide (EtBr), orange fluorescence) and yellowish autofluorescence from remains of the slime matrix. C Confocal laser scanning microscopy (CLSM) image is an optical thin-section (z=1.5 µm) from the interior of the orange slime, showing thin rod-shaped bacteria of variable lengths growing as filaments. The slime matrix around the filaments was non-fluorescent under single wavelength excitation (488 nm) of CLSM
Identifying orange-pigmented biofilm formers in machine A
From the biofilm and the clear filtrate, 67 bacterial cultures were isolated at 45 °C (Table 2). Eleven of these isolates were red-, pink- or orange-pigmented and came from R2A or CFA-plates inoculated with the orange layer or the clear filtrate (Table 2). When half-strength TSA was used, no colored isolate was found (Table 2). R2A and CFA contain less peptone and have a lower osmolarity than TSA, implying that low-nutrient isolation media better reveal the presence of colored paper-machine bacteria.
Bacterial isolates from paper mill A grouped on the basis of colony color and named on the basis of Gram reaction, morphology and whole-cell fatty acid composition. CFA Clear filtrate agar, 1/2 TSA tryptic soy agar in half-strength and amended with 1 g starch l−1
. | Number of strains isolated at 45 °C from . | ||||||||
---|---|---|---|---|---|---|---|---|---|
Isolates . | Orange slime layer . | White slime layer . | Process water (clear filtrate) . | ||||||
. | Isolation medium (pH 8.0) . | ||||||||
CFA | R2A | 1/2 TSA | CFA | R2A | 1/2 TSA | CFA | R2A | 1/2 TSA | |
Colored bacteria: | |||||||||
Orange, α-Proteobacteriaa | 1 | 3 | |||||||
Red or pink, Roseomonas-likeb | 1 | 3 | 2 | 1 | |||||
Other bacteria: | |||||||||
Thermomonas spp.c | 3 | 2 | 2 | 1 | 2 | 3 | 1 | ||
Pseudomonas stutzeri | 2 | 1 | |||||||
Unidentified gram-negative rods | 2 | 2 | 4 | 2 | 3 | 3 | 1 | 3 | |
Unidentified filamentous bacteria | 2 | 1 | |||||||
Bacillus and Paenibacillus spp. (P. macerans, B. atrophaeus, B. laterosporus+other) | 3 | 2 | 3 | 1 | 1 | ||||
Unidentified gram-positive cocci | 3 | 1 | 1 | 1 | |||||
No. of isolates (all summed=67): | 10 | 13 | 13 | 5 | 5 | 4 | 6 | 6 | 5 |
. | Number of strains isolated at 45 °C from . | ||||||||
---|---|---|---|---|---|---|---|---|---|
Isolates . | Orange slime layer . | White slime layer . | Process water (clear filtrate) . | ||||||
. | Isolation medium (pH 8.0) . | ||||||||
CFA | R2A | 1/2 TSA | CFA | R2A | 1/2 TSA | CFA | R2A | 1/2 TSA | |
Colored bacteria: | |||||||||
Orange, α-Proteobacteriaa | 1 | 3 | |||||||
Red or pink, Roseomonas-likeb | 1 | 3 | 2 | 1 | |||||
Other bacteria: | |||||||||
Thermomonas spp.c | 3 | 2 | 2 | 1 | 2 | 3 | 1 | ||
Pseudomonas stutzeri | 2 | 1 | |||||||
Unidentified gram-negative rods | 2 | 2 | 4 | 2 | 3 | 3 | 1 | 3 | |
Unidentified filamentous bacteria | 2 | 1 | |||||||
Bacillus and Paenibacillus spp. (P. macerans, B. atrophaeus, B. laterosporus+other) | 3 | 2 | 3 | 1 | 1 | ||||
Unidentified gram-positive cocci | 3 | 1 | 1 | 1 | |||||
No. of isolates (all summed=67): | 10 | 13 | 13 | 5 | 5 | 4 | 6 | 6 | 5 |
aThe main fatty acids were: 18:1 ω7cis (60–66%), 17:1 ω6cis (12–13%), 16:0 (8–12%), 14:0 2OH (4%) and 15:0 (1–3%) and 15:0 2OH (1%)
bBased on fatty-acid composition resembling that of Roseomonas gilardii [28]. The main fatty acids were: 18:1 ω7cis (48–67%), 19:0 cyclo ω8cis (2–17%), 16:0 (12–18%), 18:1 2OH (6–14%), 19:0 cyclo 2OH (3–8%) and 18:0 3OH (1–3%)
cBased on morphology (thin rods 2–5 μm length), growth at 45 °C, and presence of branched-chain fatty-acid patterns resembling that of Thermomonas haemolytica [4]. The major fatty acid was 15:0 iso (41–62%). Other main fatty acids were 16:0 iso (8–12%), 17:1 iso ω9cis (2–14%), 17:0 iso (2–9%), 15:0 anteiso (3–8%), 11:0 iso (4–6%) and 11:0 iso 3OH (4–6%)
Bacterial isolates from paper mill A grouped on the basis of colony color and named on the basis of Gram reaction, morphology and whole-cell fatty acid composition. CFA Clear filtrate agar, 1/2 TSA tryptic soy agar in half-strength and amended with 1 g starch l−1
. | Number of strains isolated at 45 °C from . | ||||||||
---|---|---|---|---|---|---|---|---|---|
Isolates . | Orange slime layer . | White slime layer . | Process water (clear filtrate) . | ||||||
. | Isolation medium (pH 8.0) . | ||||||||
CFA | R2A | 1/2 TSA | CFA | R2A | 1/2 TSA | CFA | R2A | 1/2 TSA | |
Colored bacteria: | |||||||||
Orange, α-Proteobacteriaa | 1 | 3 | |||||||
Red or pink, Roseomonas-likeb | 1 | 3 | 2 | 1 | |||||
Other bacteria: | |||||||||
Thermomonas spp.c | 3 | 2 | 2 | 1 | 2 | 3 | 1 | ||
Pseudomonas stutzeri | 2 | 1 | |||||||
Unidentified gram-negative rods | 2 | 2 | 4 | 2 | 3 | 3 | 1 | 3 | |
Unidentified filamentous bacteria | 2 | 1 | |||||||
Bacillus and Paenibacillus spp. (P. macerans, B. atrophaeus, B. laterosporus+other) | 3 | 2 | 3 | 1 | 1 | ||||
Unidentified gram-positive cocci | 3 | 1 | 1 | 1 | |||||
No. of isolates (all summed=67): | 10 | 13 | 13 | 5 | 5 | 4 | 6 | 6 | 5 |
. | Number of strains isolated at 45 °C from . | ||||||||
---|---|---|---|---|---|---|---|---|---|
Isolates . | Orange slime layer . | White slime layer . | Process water (clear filtrate) . | ||||||
. | Isolation medium (pH 8.0) . | ||||||||
CFA | R2A | 1/2 TSA | CFA | R2A | 1/2 TSA | CFA | R2A | 1/2 TSA | |
Colored bacteria: | |||||||||
Orange, α-Proteobacteriaa | 1 | 3 | |||||||
Red or pink, Roseomonas-likeb | 1 | 3 | 2 | 1 | |||||
Other bacteria: | |||||||||
Thermomonas spp.c | 3 | 2 | 2 | 1 | 2 | 3 | 1 | ||
Pseudomonas stutzeri | 2 | 1 | |||||||
Unidentified gram-negative rods | 2 | 2 | 4 | 2 | 3 | 3 | 1 | 3 | |
Unidentified filamentous bacteria | 2 | 1 | |||||||
Bacillus and Paenibacillus spp. (P. macerans, B. atrophaeus, B. laterosporus+other) | 3 | 2 | 3 | 1 | 1 | ||||
Unidentified gram-positive cocci | 3 | 1 | 1 | 1 | |||||
No. of isolates (all summed=67): | 10 | 13 | 13 | 5 | 5 | 4 | 6 | 6 | 5 |
aThe main fatty acids were: 18:1 ω7cis (60–66%), 17:1 ω6cis (12–13%), 16:0 (8–12%), 14:0 2OH (4%) and 15:0 (1–3%) and 15:0 2OH (1%)
bBased on fatty-acid composition resembling that of Roseomonas gilardii [28]. The main fatty acids were: 18:1 ω7cis (48–67%), 19:0 cyclo ω8cis (2–17%), 16:0 (12–18%), 18:1 2OH (6–14%), 19:0 cyclo 2OH (3–8%) and 18:0 3OH (1–3%)
cBased on morphology (thin rods 2–5 μm length), growth at 45 °C, and presence of branched-chain fatty-acid patterns resembling that of Thermomonas haemolytica [4]. The major fatty acid was 15:0 iso (41–62%). Other main fatty acids were 16:0 iso (8–12%), 17:1 iso ω9cis (2–14%), 17:0 iso (2–9%), 15:0 anteiso (3–8%), 11:0 iso (4–6%) and 11:0 iso 3OH (4–6%)
The majority (70%) of the 67 isolates grew poorly or not at all at 28 °C, indicating a moderately thermophilic nature. Of the 22 isolates obtained at 45 °C using half-strength TSA-plates, 11 failed to grow on TSBA, also at 45 °C, indicating toxicity of TSBA (= full-strength TSA+2.5 g dextrose l−1). At 28°C TSBA-plates supported growth of only 8 isolates. Thus, standard conditions (TSBA, 28 °C) used to grow biomass for whole-cell fatty-acid methyl esters (FAME) analysis cannot be used to identify most of these isolates. For this reason, the isolates were cultivated for FAME analysis on R2A or TSBA-plates at 45 °C instead of under standard conditions. Table 2 shows that most of the 67 isolates were gram-negative, rod-shaped Proteobacteria. Thermomonas spp. were the most frequent (21%). In the FAME analysis, there was no match between the 32 isolates (48%) from machine A and the species in the commercial fatty-acid library (MIDI version 3.9).
To identify the bacteria causing the orange slime, the 11 colored isolates were evaluated for their ability to form biofilms as pure cultures. In a microtiter plate assay (BF assay) isolates A-col-CFA-6, A-col-R2A-9d1, A-col-R2A-9d2 and A-col-R2A-9d3 formed thick biofilms in R2-broth (A 595nm 2.1–4.2) and in neutral paper-machine white water (A 595nm 0.9–1.0). The same four isolates also formed evident biofilms when grown in sterilized clear filtrate at pH 8 in glass tubes. These four orange-pigmented rod-shaped bacteria grew as filaments that were microscopically identical to those seen in the orange slime (Fig. 1C). It is thus plausible that these bacteria were responsible for producing the orange-slime in machine A. Their whole-cell fatty acid composition (Table 2) was typical for α-Proteobacteria, but no acceptable matches were found in the FAME library. The 16S rDNA sequences of the isolates A-col-CFA-6 (1,462 nucleotides) and A-col-R2A-9d1 (430 nucleotides) were determined to be identical, and 99.7% similar to the 16S rDNA sequence AF407713 sampled from microbial communities from an Australian groundwater basin with temperature of 52 °C. When compared to sequences from cultured bacteria, the highest similarities were (similarities of 97.2–98.6%) to aerobic phototrophic bacteria of the genera Porphyrobacter, Erythrobacter and Erythromicrobium. In this group P. tepidarius is the only known species capable of growth at 50 °C. The sequences of paper-machine isolates were 98.3% similar to that of P. tepidarius OK5APO (AY048657), isolated from a microbial mat in a geothermal hot spring in New Zealand; however, our four isolates differed from the P. tepidarius in morphology (filaments instead of ovoid to short rods) and contained no bacteriochlorophyll a. These "Porphyrobacter-like" isolates may represent a novel species within the α4-subgroup of the Proteobacteria. They may be of importance in the formation of orange slimes in alkaline paper-machine process water.
To reveal the origins of the orange-slime formers, water, raw materials and additives (Table 1) used by mill A were plated on R2A at 45 °C. Colored bacteria were found in two fillers: 11% (1100 CFU ml−1) of the colonies growing from ground calcium carbonate were red, and from bentonite (a clay mineral) slurry 94% (6.7×104 CFU ml−1) of the colonies were yellow. Red-pigmented "Roseomonas-like" isolates (A-gcc-R2A-1 and A-gcc-R2A-2) and a yellow Novosphingobium capsulatum-like isolate (A-bento-R2A-1) were found, but when tested for biofilm formation, they were negative (BF assay, A 595nm≤0.1). Orange "Porphyrobacter-like" isolates were not found, but the high number of colored bacteria in bentonite suggests it as a potential source for the contamination.
Red slimes in mill B
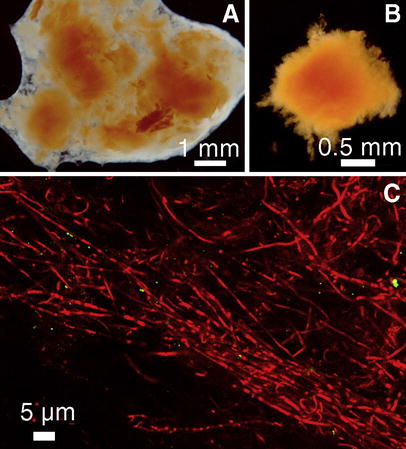
Analysis of the outbreak of red slimes in mill B. A Stereomicroscope image of unstained slime from the wire section shows compact red slime deposits surrounded with translucent slime. B The translucent slime suspended easily in contrast to the coherent red deposits causing the quality problem in mill B. C CLSM image (z=3.0 µm) from the interior of a red deposit shows mainly rod-shaped bacteria (EtBr stained, red fluorescence) growing in filaments
The cohesiveness of the red pellets, an injurious character considering the papermaking process, was exploited in an attempt to isolate the causative microbe. The red slimes were repeatedly washed with sterile water under vigorous shaking to remove the loose translucent slime and to enrich the red-colored biomass (Fig. 2B), then plated on R2A pH 5, R2A pH 7, CTYE and TSA agars, and grown 7 days at 45 or 50 °C. Nineteen red or pink-orange bacteria were isolated, of which 14 were tentatively identified as Meiothermus silvanus and four as Deinococcus geothermalis based on morphology and FAME analysis. The M. silvanus strains were identical in color and morphology to the filaments of the rod-shaped bacteria observed in the red slime.
To reveal the origin of the red slime contamination in mill B, all raw materials and additives (Table 1) were cultured on R2A at 45 °C. Intensive growth of colored bacteria was observed from dried sheets of chemical pulp and from pulper samples (wet pulp made of these sheets). Six pink-orange-pigmented strains were identified as D. geothermalis. Dry pulp sheets from the storage house of the pulp-sheet manufacturer (mill C) were also cultured on R2A and pink D. geothermalis strains were isolated. There was no growth in the chemical pulp from towers of mill C. The pulp-drying machine of mill C was then inspected, and 40 pink-, red- or orange-pigmented bacteria were isolated from the colored slimes observed in the wire section and in wells, from circulating water as well as from pieces cut off the press cylinder felts. The majority (73%) of the strains were identified as D. geothermalis or as M. silvanus, similar to those isolated from paper mill B (see groups I and II in Fig. 4). It was concluded that the clean pulp became contaminated with D. geothermalis and M. silvanus in the pulp-drying machine of the pulp mill, further contaminating the paper machine of mill B where this pulp was used.
Characteristics of colored strains from paper industry biofilms
Colored slimes were collected from two more paper mills and one board mill (D–F, Table 1) and colored bacteria were isolated using R2A and WWA agars. A total of 95 strains were obtained from the six mills included in this survey (A–F, Table 1), of which 61% were gram-negative bacteria. The majority (57%) of the strains had pink pigmentation; others were red (28%), orange (12%) or yellow (3%). Independent of the mill, all 30 red- or yellow-pigmented strains were gram-negative rod-shaped bacteria, many with a variable cell length from 2-10 µm, which often grew as filaments. Among the pink- and orange-pigmented strains, many morphotypes were present, but large gram-positive or gram-variable cocci were most frequent (36 strains). Forty-nine percent of the strains hydrolyzed starch, 56% lipids (Tween 80) and 61% a protein (casein). Hydrolysis of starch and lipids was strain-specific, showing no mill-related trends, but all 15 strains from mill A were caseinase-negative. Catalase was produced by 94% of the strains.
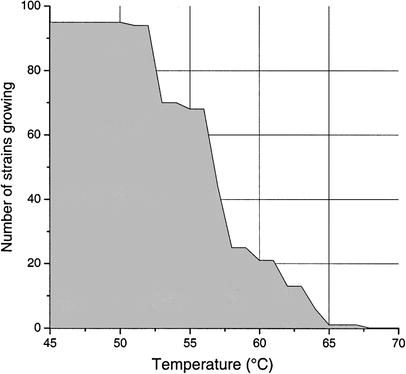
Maximum growth temperatures of the 95 colored strains. The strains were grown on R2A or CTYE-agar plates for 2 days, after which growth was assessed. The strains were then transferred to fresh agar plate, and reincubated at a temperature +3 °C higher than the previous one. The graph shows the highest temperature permitting evident growth in any of the triplicate assays for each strain
Diversity of colored paper-machine bacteria
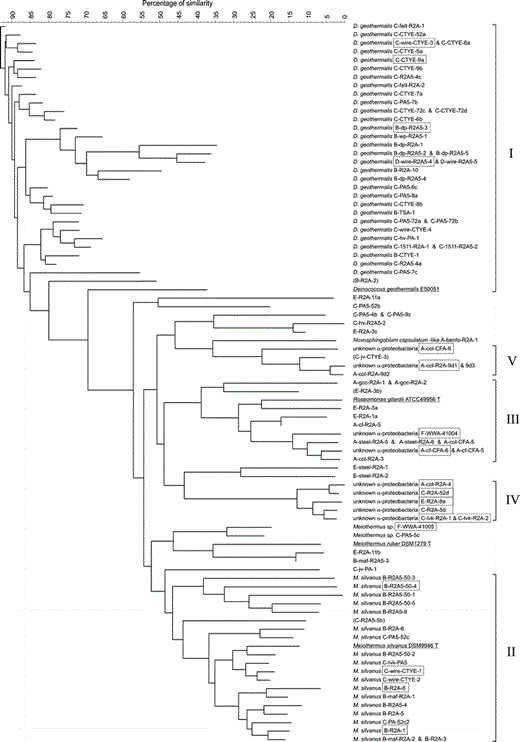
Analysis of the diversity of the 95 pigmented bacteria from the paper industry and four reference strains. The similarity tree was calculated with the neighbor-joining method from a composite data set containing results from analysis of whole-cell fatty-acid composition, T max, morphology, Gram-reaction, pigmentation, biofilm formation, catalase and selected hydrolytic activities (hydrolysis of starch, casein and lipids). The strains were named, when possible, based on the same composite data set. Strains marked with boxes were also analyzed for partial or full 16S rDNA sequences. Strains are shown in the same line if the cluster analysis showed less than 3% difference. The analysis revealed five bacterial groups (I–V). Strains whose group assignment was questionable because of one atypical phenotypic property are shown in parentheses
Group II consisted of 19 red-pigmented, rarely pink, Meiothermus silvanus strains (Fig. 4). The partial 16S rDNA sequences of four strains (B-R2A-1, B-R2A-8, B-R2A5-50-4 and C-wire-CTYE-1) were identical, and 99.8% similar to that of the M. silvanus type strain (DSM9946). This indicates phylogenetic coherency of group II. The gram-negative rod-shaped cells (0.5–0.8 µm×2.5 µm up to filaments) had a T max of 59–63 °C, except for one strain with a T max as high as 67 °C. The predominant fatty acids were iso- and anteiso-branched (≥77% of the total amount), 15:0 anteiso (31–42%) as the major component. Typical signature fatty acids in minor amounts (1–7%) were 17:0 iso 2OH, 17:0 anteiso 2OH, 17:1 ω6cis, 13:0 iso 3OH and an unknown compound with equivalent chain length (ECL) of 16.09. All strains were unable to hydrolyze lipids or starch and all showed catalase activity, unlike the type strain of M. silvanus, which may indicate adaptation to a hostile environment with occasional usage of oxidizing biocides such as peracetic acid. The M. silvanus strains of group II were isolated from two mills in connection with a red-slime outbreak, and one related strain, Meiothermus sp. F-WWA-41005, was isolated from red slime from a board machine, suggesting that Meiothermus species may play a role in outbreaks of red slimes in the paper industry.
Group III consisted of 12 strains (Fig. 4) of pink- or red-pigmented gram-negative bacteria. The cells were coccoid rods, but some strains were also able to grow as longer rods in filaments. The major fatty acids of these strains were 19:0 cyclo ω8cis, 18:1 ω7cis (together 51–70% of the total fatty acids), 16:0 (12–33%), 18:1 2OH (4–14%), the signature fatty acid 19:0 cyclo 2OH (3–16%) and 18:0 3OH (1–4%). All strains produced catalase, and did not hydrolyze starch or casein. Based on these biochemical results the strains resembled the species Roseomonas gilardii. The T max of the strains was 52–57 °C, clearly higher than the T max of 47 °C of the type strain of R. gilardii (ATCC49956). The partial 16S rDNA sequences of strains F-WWA-41004 and A-cf-CFA-6 from two different mills were 99.8% identical. The highest similarity was only 94.9–95.1% to R. genomospecies 4, followed by 93.6% to Teichococcus ludipueritiae [10] and 93.2–93.4% to R. gilardii. Thus, the strains may represent a new genus of α-Proteobacteria related to Roseomonas. Strains of group III were present in slimes and circulating water of three different types of machines (A, E and F in Table 1; pH 7–9) using different raw materials. In mill A, these bacteria were also found in the calcium carbonate filler. The results suggest that these "Roseomonas-like" bacteria are frequently present in the slimes and process water of neutral and alkaline machines.
Group IV consisted of six strains of α-Proteobacteria (Fig. 4) with red-pigmented rod-shaped cells (0.6–0.8 µm×2.0–4.0 µm) having a T max of 56–57 °C. All strains were unable to hydrolyze starch, casein or Tween 80. The major fatty acids were 19:0 cyclo ω8cis (39–44%), 18:0 (21–24%), 16:0 (21–23%), and 18:1 ω7cis (4–7%), whereas minor signature fatty acids were an unknown ECL 11.799 (2–3%), 11 methyl 18:1 ω7cis (1%) and 10:0 3OH (1%). The partial 16S rDNA sequences of the six strains were identical. The full 16S rDNA sequence of strain A-col-R2A-4 had highest similarities of only 93.5% to phototrophic Rhodobacter blasticus ATCC33485T and 93.1% to colorless Paracoccus kocurii JCM7684T. Therefore, our strains likely represent a new genus within the Rhodobacteraceae family of α-Proteobacteria. They were isolated from slimes in two paper machines (mills A and E) and a pulp dryer (mill C) in different seasons, suggesting that these bacteria are regular inhabitants of colored slimes.
Group V consisted of the "Porphyrobacter-like" strains from mill A and an orange-pigmented isolate C-jv-CTYE-3 from the cooling water of mill C. However, isolate C-jv-CTYE-3 had coccoidal cells in contrast to the filamentous morphology of the other four strains; thus its membership of this group remained unclear.
Analysis of biofilm formation potential
Biofilm formation (BF) of pigmented paper-industry strains at 45 °C in 2 days and the effects of methylene bisthiocyanate (MBT) and 2,2-dibromo-3-nitrilopropionamide (DBNPA) biocides. BF was measured in wells of microtiter plates. Bars Average A 595±SD of quadruplicate wells containing crystal-violet-stained biofilms after dissolution of the stain in ethanol. For the MBT and DBNPA tests, the length of each shaded section in a bar corresponds to the absorbance value for either 5 ppm or 15 ppm of the biocide, and is not a cumulative value for 15 ppm. Error bars Biocide concentration of 15 ppm. Strains are shown in the same line if they had similar biofilm behavior
Figure 5A shows that 56% of the colored strains formed evident biofilm (A 595nm ≥0.3) as pure cultures in WW medium at pH 7. Eleven strains formed significantly (p<0.05) more biofilm than D. geothermalis E50051, a known paper-machine primary biofilm former. The most effective biofilm former (A 595nm 2.1) was M. silvanus strain B-R2A5-50-4 (group II) isolated from the wire section of machine B during the red-slime outbreak.
Figure 5B shows that only 16% of the strains produced biofilm (A 595nm ≥0.3) in WW medium at pH 5, indicating that the acidic white water supported less biofilm growth than neutral white water. At pH 5, the most effective biofilm former (A 595nm 0.6) was D. geothermalis strain C-PA5-6c (group I), originating from pink slime in the wire section of the pulp dryer of mill C. The nine most effective biofilm formers at pH 5 were all identified as D. geothermalis.
Figure 5B also shows that all colored strains originating from machines with pH ≥7 (A-, E- and F-coded strains) formed no evident biofilm in WW at pH 5. This implies that the colored biofilm-formers in paper machines operating under neutral or alkaline conditions are different from those in acidic machines. Comparing the ten best biofilm-forming strains both at pH 5 and at pH 7 supported this view, as only three strains (D. geothermalis B-R2A-10, C-PA5-7c and C-CTYE-9a) behaved the same at the two pH values.
In R2-broth (right column in Fig. 5B), 66% of the strains produced biofilms (10% more strains than in WW medium), indicating that the majority of the colored strains were biofilm formers in the laboratory. In general, the biofilms grew thicker (A 595nm up to 4.3) in R2-broth than in WW medium, but some strains formed thick biofilms in WW medium only, e.g. D. geothermalis B-R2A-10 at pH 7, and not in R2-broth. This shows that the strains varied with respect to conditions optimal for biofilm growth, and weak biofilm formation in R2-broth does not necessarily imply the inability of a strain to form biofilm under machine conditions.
Effects of biocides on formation of colored biofilms
Figure 5A shows that 5 mg MBT (methylene bisthiocyanate) per l WW medium at pH 7 prevented (>90% reduction of A 595nm) the biofilm formation of only seven strains (e.g. C-R2A-5d, group IV) and increased the biofilm formation of 34 strains by 5–753% (e.g. A-col-CFA-5, group III). This indicates that not only was 5 ppm MBT insufficient to prevent biofilm formation in WW medium at pH 7, but the biocide in fact stimulated bacterial production of biofilms, possibly by making the free-swimming mode of growth unfavorable. Fifteen ppm MBT prevented biofilm growth of more strains than 5 ppm (e.g. A-col-R2A-4, group IV), but 22 strains still formed biofilms (42% of the biofilm formers in WW medium, pH 7, with no biocides). Twelve of these 22 strains increased their biofilm formation by 3–746% (e.g. D. geothermalis C-PA5–8a). The thickest biofilms in WW medium at pH 7 in the presence of 15 ppm MBT were formed by three strains of D. geothermalis (B-R2A-10, C-CTYE-52a and C-wire-CTYE-3). Five and 15 ppm MBT prevented (>90% reduction of A 595nm) biofilms of none of the strains in WW medium at pH 5 (Fig.5 B). In contrast, there were more biofilm-forming strains in the presence of 15 ppm MBT (20 strains) than in the absence of MBT (15 strains only) at pH 5. As an example M. silvanus B-R2A50-4 (group II) increased biofilm formation by 67%. The results indicate that MBT may have been effective against free-swimming bacteria but did not prevent biofilm formation by many colored bacteria of paper industrial origin.
Figure 5A shows that 5 and 15 ppm DBNPA (2,2-dibromo-3-nitrilopropionamide) in WW medium at pH 7 prevented (>90% reduction of A 595nm) biofilm formation in none of the colored strains. In fact, 15 ppm DBNPA increased biofilm formation of 39 strains by 2–54%. Therefore, DBNPA was less effective than MBT in preventing biofilm growth at pH 7. The thickest biofilms were formed by M. silvanus B-R2A50-4. At pH 5, DBNPA also did not prevent biofilms (Fig. 5B), but 15 ppm caused >50% reduction in biofilm growth of seven strains, indicating that DBNPA was slightly more effective at this pH than MBT.
In conclusion, in neutral white water 15 ppm MBT prevented biofilms better than DBNPA, but in acidic white water the situation reversed. For many strains, however, the presence of 5 and 15 ppm of the biocides probably made the free-swimming mode of growth unfavorable, but did not prevent the growth of bacteria as a biofilm, thus initiating or stimulating the biofilm mode of growth.
Based on the results in Fig. 5A & B, bacteria of groups I and II (D. geothermalis and M. silvanus) were primary biofilm formers both in neutral and acidic paper-machine water. In WW medium at pH 7, over 80% of the D. geothermalis strains and 50% of the M. silvanus strains formed biofilms that, in general, were reduced by 15 ppm of MBT, but not by DBNPA. The Roseomonas-like strains (group III) formed no thick biofilms in the absence of biocides, but MBT stimulated biofilm formation of some strains, e.g. A-col-CFA-5, in WW medium at pH 7. The Roseomonas-like strains seem not to belong to the primary-colonizers of machine surfaces. The Rhodobacter-related strains (group IV) and the "Porphyrobacter-like" strains (group V) formed thick biofilms in neutral, but not in acidic paper-machine water. Their biofilm formation was prevented by MBT but not by DBNPA.
Discussion
This report presents a microbiological survey of colored bacteria in slimes in six paper and board machines. Case studies from mills A and B showed that media low in nutrients (R2A or process-water-based agars), isolation temperatures of 45–50 °C and pre-washing of the slime samples are an efficient combination when searching for the causative bacteria of colored biofilms. In this way, we established a collection of 95 pigmented paper-machine strains, including putative new species, many of which were unable to grow on TSA or TSBA. We reported earlier [12] that the use of nutrient-rich cultivation media such as TSA or plate count agar, typically used for hygienic process-control purposes, might have caused overestimation of the role of Bacillus species in the paper-machine slimes, as these fast-growing bacteria can override others in laboratory cultures. Here we demonstrate that use of rich media may mask those species primarily responsible for the formation of colored slimes.
We show here that D. geothermalis is a common pink-slime-former in the paper industry rather than an accidental contaminant as was assumed [2]. It was the most frequent colored species isolated from acidic paper machines, and in the BF assay D. geothermalis strains were the most effective primary biofilm formers in acidic paper-machine water. Excepting some strains of D. geothermalis that formed biofilms both in neutral and acidic paper-machine water, our results also suggest that paper machines operating under neutral and acidic conditions contain different bacteria as primary biofilm formers.
The case study of mill B showed that biofouling of a pulp dryer can contaminate clean chemical pulp, that the contaminant D. geothermalis survived the pulp drying, and that carry-over of the colored bacteria likely triggered the outbreak of red slimes in the recipient paper machine. M. silvanus and D. geothermalis were both shown to be present in the slimes of the pulp dryer and machine B, but only D. geothermalis was detected from the dry sheets of pulp. We assume that both species were transferred within the dry pulp, but D. geothermalis was easier to detect, as bacteria of the genus Deinococcus are highly resistant towards desiccation [2].
Species of the genus Meiothermus are slightly thermophilic and have been found in several hydrothermal areas and also in hot fermentors fed with wastewater [16]. This is the first report describing the presence of Meiothermus species in paper mills, showing the capability of M. silvanus for biofilm formation, and suggesting an important role for it in outbreaks of red slimes. The ability of M. silvanus and D. geothermalis to actively form biofilm is understandable in the perspective of their natural habitat: the colored microbial mats (biofilms) in geothermal hot springs [2, 16]. Similarly, the "Porphyrobacter-like" orange-slime formers from mill A were closely related to aerobic phototrophic bacteria that have hot springs as their natural habitat [30]. The warm paper machines seem to offer a man-made ecological niche for many bacteria of the normal flora of hot springs.
The "Roseomonas-like" bacteria, putatively a new species, were commonly found in colored slimes although these isolates were incapable of primary colonization of clean surfaces. Bacteria of the genus Roseomonas are described as pathogenic for humans, causing bacteremia and other infections [22], but we do not know whether the Roseomonas-like bacteria in the paper machines have pathogenic potential. The biohazard may be low, as these bacteria do not form thermoresistant spores and will likely be killed by heat in the drying section of the paper machines. Oppong et al. [17] reported nine pink-pigmented isolates from paper machine slimes including also one Roseomonas sp. isolate from a white water filter. The other isolates were different from the isolates in our study, which is not surprising because Oppong et al. [17] used isolation temperatures as low as 25–30 °C. The majority of the pigmented strains in our study were moderately thermophilic, 22% of the strains were able to grow even at 61 °C. Most strains had a T max 5–15 °C higher than the temperatures on the biofouled surfaces from which they were isolated. The trend of increasing the operating temperatures of paper machines is likely to continue in the future. Our results demonstrate that, in the machines investigated, temperature increments of less than 10 °C will not prevent colored biofouling.
In addition to the colored strains, we isolated 14 strains of Thermomonas spp. from mill A. Prior to this we isolated 11 Thermomonas spp. strains from biofilms in two paper machines [12]. The type strain of T. haemolytica also originated from kaolin slurry used for paper production [4]. These results imply that Thermomonas spp. are common in paper machines.
Increasing evidence exists that many bacteria possess two differentiated growth modes, the planktonic and the biofilm mode [29], and that the cells of the sessile biofilm phenotype have reduced susceptibility to killing by biocides and antibiotics [6, 7]. When killing times for biofilm and planktonic cells were compared, Pseudomonas aeruginosa biofilms had resistance factor 29 for DBNPA, 34 for glutaraldehyde, 120 for chlorine and 1,900 for an alkyl dimethyl benzyl ammonium compound [7]. This indicates that data on antimicrobial efficacy obtained with planktonic bacteria are not reliable indicators of performance against biofilms [7]. MBT and DBNPA are commonly used biocides in the paper industry, but little is known about their effectiveness against paper-machine biofilms. We used the microtiter plate assay, the relevance of which has been shown previously [12, 13], to study the effects of MBT and DBNPA on the formation of biofilm by the colored strains. In general, colored biofilms of groups I, II, IV and V were better prevented by MBT than by DBNPA, when applied in concentrations used in the industry. But it is worth noting that the cell density in paper-machine circulating water is not always as high as that required for the BF assay (after inoculation up to 5×107 CFU ml−1). The high cell density may have increased biocide inactivation by the cells, making this BF assay a sensitive tool to disclose biocide tolerant strains. Instead of eradicating biofilms, MBT or DBNPA actually increased biofilm formation by many strains. It is likely that the biocides prevented planktonic growth but failed to prevent growth as a biofilm, thus favoring the latter mode of growth. Similarly, Das et al. [6] reported that changes in biocide susceptibility of Staphylococcus epidermidis and Escherichia coli occurred immediately upon attachment, and growth of attached cells persisted in biocide concentrations that inhibited growth of planktonic cells. Our results imply that suboptimal biocide doses in paper machines may cause the bacteria to seek protection by shifting to growth in biofilms and thus increase biofouling of the process. This is in agreement with the results of Grobe et al. [7], who reported that improved killing of P. aeruginosa biofilms required a concentrated dose of biocide rather than prolonged doses of a lower concentration.
Acknowledgements
The Viikki Graduate School in Biosciences of Helsinki University, Kemira Chemicals Oy, National Technology Agency (TEKES) and Finnish paper industry supported this study. We acknowledge the use of the Helsinki University CLSM facilities at the Institute of Biotechnology. We thank Justus Reunanen and Inka Harju for technical assistance. Edward Moore and Annette Krüger from GBF (Braunschweig, Germany) and DSMZ Identification Service (Braunschweig) are acknowledged for the 16S rDNA sequence determinations.
References
Battista JR, FA Rainey (2001) Genus Deinococcus. In: Boone DR, Castenholz RW and GM Garrity (eds) Bergey's manual of systematic bacteriology, 2nd edn. Springer, Berlin Heidelberg New York, pp 396–403
Nobre MF, MS DaCosta (2001) Genus Meiothermus. In: Boone DR, Castenholz RW and GM Garrity (eds) Bergey's manual of systematic bacteriology, 2nd edn. Springer, Berlin Heidelberg New York, pp 414–420
Smibert RM, NR Krieg (1994) Phenotypic characterization. In: Gerhardt P, RG Murray, W Wood et al (eds) Methods for general and molecular bacteriology. American Society for Microbiology, Washington DC, pp 607–654
Stoesser G, W Baker, A van den Broek, E Camon, M Garcia-Pastor, C Kanz, T Kulikova, R Leinonen, Q Lin, V Lombard, R Lopez, N Redaschi, P Stoehr, M Tuli, K Tzouvara, R Vaughan (2002) The EMBL Nucleotide Sequence Database. Nucleic Acids Res 30:21–26. http://www.ebi.ac.uk/embl/