-
PDF
- Split View
-
Views
-
Cite
Cite
Albert Juan Fuglsang-Madsen, Nicole Lind Henriksen, Elizabeth Serrano Chávez, Lasse Andersson Kvich, Julie Knippel Melsted Birch, Katrine Top Hartmann, Thomas Eriksen, Thomas Bjarnsholt, Hans Gottlieb, Thomas Lars Andresen, Louise Kruse Jensen, Jonas Rosager Henriksen, Anders Elias Hansen, Eradication of Staphylococcus aureus in Implant-Associated Osteomyelitis by an Injectable In Situ-Forming Depot Antibiotics Delivery System, The Journal of Infectious Diseases, Volume 230, Issue 3, 15 September 2024, Pages 614–623, https://doi.org/10.1093/infdis/jiae139
- Share Icon Share
Abstract
Bone infections with Staphylococcus aureus are notoriously difficult to treat and have high recurrence rates. Local antibiotic delivery systems hold the potential to achieve high in situ antibiotic concentrations, which are otherwise challenging to achieve via systemic administration. Existing solutions have been shown to confer suboptimal drug release and distribution. Here we present and evaluate an injectable in situ-forming depot system termed CarboCell. The CarboCell technology provides sustained and tuneable release of local high-dose antibiotics.
CarboCell formulations of levofloxacin or clindamycin with or without antimicrobial adjuvants cis-2-decenoic acid or cis-11-methyl-2-dodecenoic acid were tested in experimental rodent and porcine implant-associated osteomyelitis models. In the porcine models, debridement and treatment with CarboCell-formulated antibiotics was carried out without systemic antibiotic administration. The bacterial burden was determined by quantitative bacteriology.
CarboCell formulations eliminated S. aureus in infected implant rat models. In the translational implant-associated pig model, surgical debridement and injection of clindamycin-releasing CarboCell formulations resulted in pathogen-free bone tissues and implants in 9 of 12 and full eradication in 5 of 12 pigs.
Sustained release of antimicrobial agents mediated by the CarboCell technology demonstrated promising therapeutic efficacy in challenging translational models and may be beneficial in combination with the current standard of care.
Osteomyelitis is a medically challenging and societally costly disease [1]. Recurrence rates are high, ranging from 5–40 % depending on anatomical location, comorbidities, and presence of foreign bodies [2]. Chronic osteomyelitis is regarded as biofilm infections, and 30–60 % of cases are estimated to be caused by Staphylococcus aureus [3]. Biofilm infections are considered difficult to treat, and the biofilm state is known to increase the embedded bacterias’ tolerance to antibiotics by up to 1000-fold, in large part caused by slow-growing bacteria [4]. While systemically administered antibiotics struggle to reach site-specific biofilm-eradicating concentrations [5], local drug-eluting technologies offer a promising solution to achieve minimum biofilm eradication concentrations (MBEC) in infected bone [6]. This is because achieving adequate site-specific concentrations through systemic administration requires high dosages, which can lead to partitioning into off-target tissues and subsequent toxicity. In contrast, local drug-eluting technologies could achieve MBEC with a lower dose of antibiotics, potentially also reducing the risk of adverse reactions and antimicrobial resistance development.
Current treatment consists mainly of systemic antibiotics, often supplemented with antibiotic-loaded bone cements or void fillers. However, it remains uncertain whether these drug-eluting technologies improve therapeutic outcomes [6–8], and their benefit is debated among clinicians [6, 8]. Consensus statements support the potential of novel or improved local antibiotic-eluting depot technologies for controlling biofilm infections [6]. Therefore, we aimed to design a technology that exhibits sustained high-dose release, which can be strategically injected and distributed in tissues.
To achieve these characteristics, we designed the CarboCell technology, an injectable, in situ-forming depot delivery system based on hydrophobic carbohydrate esters, triglycerides, and organic solvents (examples are shown in Figure 1). The CarboCell technology forms a hydrophobic liquid, which can be used to solubilize hydrophobic or amphiphilic compounds. Once injected into an aqueous environment, the organic solvent leaves the matrix, forming a drug depot that releases antibiotics over time. Importantly, the CarboCell technology is not restricted to open surgical sites but can be injected strategically into cancellous bone, cavities, and soft tissue using thin needles, and releases antimicrobials where infection resides (conceptualization; Figure 1A–C). The drug release period and viscosity of CarboCells can be tailored by modifying the composition (Figure 1D) [9].
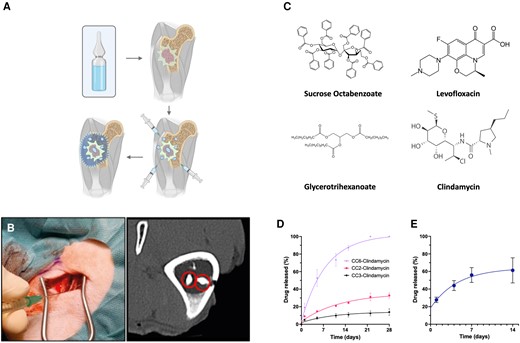
The concept of CarboCells technology, practical applicability, constituents used in this study, and in vitro and in vivo release of selected formulations of clindamycin. A, CarboCells (light blue) can be injected strategically across infected lesions and areas to optimize antibiotic distribution (outlined area). B, In vivo injection can be performed using standard thin needles; depots can comprise contrast agents visible in bone tissue and exhibits locoregional stability over time. C, Molecular structures of the hydrophobic sugar ester, triglyceride, and lead antibiotics utilized in this study. D, Examples of in vitro release curves of clindamycin from compositions (left; formulations CC6, CC2, and CC3; see Table 1 for formulation details), or from in vivo formulation CC3 (right). Release data are shown as the mean value ± SEM. Methods for release quantification and high-pressure liquid chromatography can be found in “Methods” section and Supplementary Methods, respectively.
In this article, we investigate the antimicrobial efficacy of the CarboCell technology loaded with antibiotics or in combinations with potentiating agents in experimental rodent- and porcine implant-associated infection models. For the experimental implant-associated osteomyelitis porcine model, it is known that an inoculum of 104 colony-forming units (CFU) does not resolve naturally [7, 10–16]. Furthermore, debridement with administration of a gentamicin-eluting bone cement does not lead to infection clearance (n = 6 [7]), and a study by Vittrup et al in 2024, using the exact same experimental protocol as in this study, showed that immunohistochemical (IHC) staining for S. aureus in 4 of 8 pigs that received intravenous rifampicin + moxifloxacin were positive postmortem, and likewise for 3 of 7 pigs that received moxifloxacin alone [16]. They also showed that in all the pigs, the neutrophil count fulfilled the confirmatory infection criteria, demonstrating the difficulty of eradicating infection in the model.
For the chosen adjuvants in this study, cis-2-decenoic acid (C2DA) and diffusible signal factor (DSF, cis-11-2-methyl-dodecenoic acid), the intention was to exploit their reported biofilm-dispersing activity to reduce the required killing concentration of the primary antibiotic [17–20]. This might extend the effective radius around each CarboCell depot for achieving staphylocidal concentrations.
METHODS
Selection of Active Pharmaceuticals
Compounds with expected activity against S. aureus and/or its biofilm were selected, and included combinations of levofloxacin, C2DA, DSF, gentamicin, linezolid, vancomycin, and clindamycin [17, 21–27].
Formulations of CarboCell
Formulations were made by weighing the components sucrose- octabenzoate, glycerotrihexanoate, ethanol, or dimethyl sulfoxide, followed by sonication at 75 °C until homogenous and translucent. Antimicrobials were dissolved by adding the desired mass of CarboCell liquid. CarboCell compositions used in this work are listed in Table 1. The CarboCell composition changes conducted throughout the study resulted from iterative optimization to improve release kinetics and antibiotic solubility.
CarboCell Designation . | SuBen, w/w, % . | GTH, w/w, % . | EtOH, w/w, % . | DMSO, w/w, % . | SuBen/GTH Ratio . |
---|---|---|---|---|---|
CC1 | 60 | 15 | 25 | … | 4 |
CC2 | 60 | 20 | 20 | … | 3 |
CC3 | 64 | 16 | 20 | … | 4 |
CC4 | 60 | 15 | … | 25 | 4 |
CC5 | 64 | 16 | … | 20 | 4 |
CC6 | 55 | 25 | … | 20 | 2.2 |
CarboCell Designation . | SuBen, w/w, % . | GTH, w/w, % . | EtOH, w/w, % . | DMSO, w/w, % . | SuBen/GTH Ratio . |
---|---|---|---|---|---|
CC1 | 60 | 15 | 25 | … | 4 |
CC2 | 60 | 20 | 20 | … | 3 |
CC3 | 64 | 16 | 20 | … | 4 |
CC4 | 60 | 15 | … | 25 | 4 |
CC5 | 64 | 16 | … | 20 | 4 |
CC6 | 55 | 25 | … | 20 | 2.2 |
The table provides an overview of all CarboCell compositions of this study and their chemical composition.
Abbreviations: CC, CarboCell; DMSO, dimethyl sulfoxide; EtOH, ethanol; GTH, glycerotrihexanoate; SuBen, sucrose octabenzoate.
CarboCell Designation . | SuBen, w/w, % . | GTH, w/w, % . | EtOH, w/w, % . | DMSO, w/w, % . | SuBen/GTH Ratio . |
---|---|---|---|---|---|
CC1 | 60 | 15 | 25 | … | 4 |
CC2 | 60 | 20 | 20 | … | 3 |
CC3 | 64 | 16 | 20 | … | 4 |
CC4 | 60 | 15 | … | 25 | 4 |
CC5 | 64 | 16 | … | 20 | 4 |
CC6 | 55 | 25 | … | 20 | 2.2 |
CarboCell Designation . | SuBen, w/w, % . | GTH, w/w, % . | EtOH, w/w, % . | DMSO, w/w, % . | SuBen/GTH Ratio . |
---|---|---|---|---|---|
CC1 | 60 | 15 | 25 | … | 4 |
CC2 | 60 | 20 | 20 | … | 3 |
CC3 | 64 | 16 | 20 | … | 4 |
CC4 | 60 | 15 | … | 25 | 4 |
CC5 | 64 | 16 | … | 20 | 4 |
CC6 | 55 | 25 | … | 20 | 2.2 |
The table provides an overview of all CarboCell compositions of this study and their chemical composition.
Abbreviations: CC, CarboCell; DMSO, dimethyl sulfoxide; EtOH, ethanol; GTH, glycerotrihexanoate; SuBen, sucrose octabenzoate.
In Vitro and In Vivo Release Studies
In vitro release was investigated by injecting antibiotic-loaded CarboCell into phosphate-buffered saline (PBS) followed by incubation at 37°C. At specified time points, samples were retrieved, and high-pressure liquid chromatography (HPLC; Supplementary Methods) analyses were carried out to determine the release of antibiotics as a function of time. In vivo release was quantified by sampling depots postmortem and quantifying the released amounts by HPLC analysis (Supplementary Methods).
In Vivo Experiments
Infected Implant Rat Model
Fifty-nine male Wistar rats (400–500 g; Janvier, France) were gas-anesthetized using 2.5 % volume/volume isoflurane, received subcutaneous (S.C.) injections of 0.01 mg/kg buprenorphine (Indivior Europe) and 2 mg/kg meloxicam (Boehringer Ingelheim), and prepared for surgery. A 1–2 cm incision was made along the femur diaphysis to expose the femoral bone. A 1.8 mm diameter mid-diaphyseal hole was drilled, followed by the insertion of a 2–4 mm stainless-steel pin (1 mm diameter), which was coated with an in vitro-matured S. aureus S54F9 biofilm (a highly virulent strain [28, 29]) by incubation at 37°C/180 rpm for 4 days. The inoculum dosage was 6.4 ± 1.0 log CFU/cm2. Bone wax (Mediq) was applied to close the hole, and 200 µL antibiotic-loaded CarboCell was divided between S.C. tissue at the bone surface and more superficially. Control animals that did not receive CarboCell (or received unloaded CarboCell) were included in each experiment. Animals were completely randomized to treatment groups, and surgeons were blinded to the treatment groups. Daily per oral administration of buprenorphine in Nutella (0.2 mg/g) at 2 g/kg/24 hours was provided for 72 hours postsurgery. Rats were euthanized 7 days postsurgery, implants, whole femurs, and 3 × 0.5–1 g S.C. samples were collected for microbiological analysis. The femurs were crushed using sterilized implant cutters before homogenization and sonication as described. All experiments were approved by the Danish Animal Inspectorate (2020-15-0201-00713) and no rats were euthanized prematurely.
Implant-Associated Porcine Model
Fifteen female SPF pigs (30–35 kg) were acclimatized for 1 week. Two were included in a prophylaxis study (Supplementary Materials). As previously described, implant-associated osteomyelitis was induced by drilling a 4 × 20 mm hole in the right proximal tibia, followed by inoculation of 104 CFU S. aureus S54F9 in 10 μL saline and insertion of a 2 × 15 mm steel implant [7, 11, 12, 30]. After 7 days, revision surgery was conducted [7]. Briefly, soft tissue abscesses were drained, implants removed, and necrotic bone tissue debrided, followed by irrigation using 1 L 0.9% saline. Thus, the protocol mimics a clinical 1-stage revision. S.C. tissue, bone tissue, and implants were collected for microbiological analysis during debridement. Pigs were completely randomized to receive approximately 8 mL local treatment with 80 mg/g clindamycin-loaded CC3 (n = 5), 80 mg/g clindamycin-loaded CC2 (n = 5), or 80 mg/g clindamycin + 5 mg/g DSF-loaded CC3 (n = 3). Antibiotic-loaded CarboCells were injected into the bone void and surrounding cancellous bone and S.C. tissue. A stainless-steel implant (approximately 2 × 30 mm) was fixed in the bone void by carefully driving it into cancellous bone, and the wound was closed in 2 layers. Pigs received a single intramuscular injection of buprenorphine (Salfarm Danmark) and daily per oral meloxicam throughout the study. Pigs were euthanized 7 days after treatment (Euthanimal, ScanVet). One pig (CC2-clindamycin 80 mg/g) was euthanized after 4 days due to lameness. The cause of lameness was not identified by necropsy. Necropsy and macroscopic assessment of the wound and bone were carried out for all pigs. Implants, bone tissue (3–5 samples/pig), and S.C. tissue (3 samples/pig) were collected for microbiological and histological analysis. Experimental animal work was approved by the Danish Animal Inspectorate (2017-15-0201-01356).
Microbiological Assays
Autoclaved stainless-steel balls were added to samples, followed by homogenization (20 minutes, 1500 rpm at room temperature; Retsch MM 400). Cold PBS was added, and bacteria and tissue were pelleted by centrifugation (4 °C, 5000 g for 10 minutes). The supernatant was removed, and 1 mL of PBS was added. All samples were sonicated (10 minutes, 4 °C; Branson 1510) to detach bacteria and disperse biofilms. Samples were serially diluted and plated on lysogeny broth (LB) agar plates. Plates were incubated at 37°C for 18–24 hours before counting CFUs. If no colonies were observed, the entire sample volume was plated to confirm pathogen eradication or, in the case of rodent studies, elimination of in vitro-matured biofilm. Logarithmic-transformed CFUs per gram of sample + 1 (logCFU/g + 1), reported as logCFU/g throughout the article, is the average across multiple sampling sites or pooled prior to dilution and plating. CFUs were tested by an agglutination test according to the manufacturer's protocol (ThermoScientific) to validate the identification of S. aureus. If CFUs were not readily identifiable, matrix-assisted laser desorption ionization time-of-flight mass spectrometry (MALDI-TOF MS) analysis was conducted. Samples were randomized prior to sample preparation and unblinded after CFU counting.
Histopathology
Sectioned tibiae were placed in 4 % neutral-buffered formaldehyde (1 week), followed by decalcification in 22 % formic acid (4 weeks). Decalcified specimens were paraffin-embedded and sectioned (4–5 μm). Sections were stained with hematoxylin and eosin and by immunohistochemistry based on an S. aureus-specific antibody, as previously described, and evaluated blindly [31, 32]. Neutrophilic granulocytes were counted in 10 high-power fields (40 × magnification) in the pathological bone area, defined as the perpendicular distance from the bone void border to the appearance of normal trabecular bone [7, 11]. A cutoff of 5 neutrophilic granulocytes in ≥ 5 high-power fields was used to define infection [33]. CarboCell depots were identified as white rounded structures with a larger diameter than bone marrow adipocytes.
Statistical Analyses
One-way ANOVA was performed for rodent studies where untreated control groups were included, and multiple comparisons were corrected by Dunnett’s test. Statistical analyses were performed using GraphPad Prism version 9.4.1. P values are reported as *P ≤ .05, **P ≤ .01, ***P ≤ .001, and ****P ≤ .0001. Data are presented as mean ± SEM.
RESULTS
Selected Antimicrobials Were Active and Soluble in the CarboCell System
Levofloxacin, clindamycin, and C2DA were soluble in CarboCells (Supplementary Table 1), and all exhibited bactericidal activity against S. aureus S54F9 by disk diffusion (Supplementary Figure 1). Furthermore, in an implant-associated mouse model, cases with complete elimination of in vitro-matured S. aureus biofilm were observed by CarboCell-mediated treatment with clindamycin alone or in combination with C2DA (Supplementary Figure 2 and Supplementary Table 2). Moreover, clindamycin remained stable in CarboCells over time (Supplementary Table 3 and Supplementary Figure 3).
CarboCell-Formulated Antibiotics Can Eliminate In Vitro-Matured S. aureus Biofilm in an Implant Rat Model
CarboCell formulations of clindamycin or levofloxacin in combination with C2DA were evaluated in a biofilm-infected implant rat model. A significant reduction of bacterial burden in S.C. tissue, bone, and implants was observed for both combinations, but with no difference between treatments. In total, 7 of 12 CarboCell-treated animals displayed complete elimination of in vitro-matured S. aureus biofilm (Figure 2).
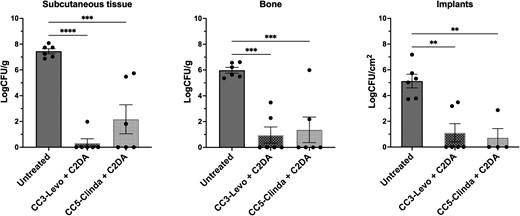
Quantitative microbiological results from treating an infected implant rat model with clindamycin or levofloxacin co-formulated with C2DA in CarboCells, without systemic antibiotic administration. The bacterial load is shown as logCFU/g or logCFU/cm2 in subcutaneous tissue, bone, and implants after 7 days of treatment with levofloxacin (20 mg/g) + C2DA (5 mg/g) formulated in CC3) or clindamycin·HCl (20 mg/g) + C2DA (5 mg/g) formulated in CC5. See formulation details in Table 1. Each circle represents an individual animal. A 1-way ANOVA analysis was conducted. Data are shown as mean ± SEM. **P ≤ .01, ***P ≤ .001, ****P ≤ .0001. Release data for each formulation can be found in Supplementary Table 5. Abbreviations: C2DA, cis-2-decenoic acid; CC, CarboCell; CFU, colony-forming unit; Clinda, clindamycin; Levo, levofloxacin.
Next, it was tested if adding the biofilm-dispersing agent, DSF [27, 34], or an additional antibiotic (linezolid + clindamycin), would increase therapeutic efficacy. Clindamycin + DSF was the only combination that significantly reduced the bacterial burden in S.C. tissue, bone, and implants (Figure 3A). Based on this observation, it was tested if co-formulations of DSF and clindamycin were beneficial when the duration of treatment was 1 or 2 weeks. Although in vitro-matured S. aureus biofilm was eliminated in several animals, there was no difference between 1 or 2 weeks (Figure 3B). No added benefit of biofilm-dispersing agents at the tested dose was observed, despite previous studies reporting promising in vitro activity [17–20]. Furthermore, when comparing 30 to 80 mg/g clindamycin in the implant-associated rat model, there was no significant benefit of increasing the concentration (Supplementary Figure 4). However, considering the larger volume of distribution in larger animals, the 80 mg/g clindamycin concentration was advanced to experimental porcine models. To obtain a complete overview of all animal experiments included in this experiment, see Supplementary Table 4.
![Treatment of infected implant rat models by CarboCell-formulated clindamycin alone or in combination with DSF. A, Bacterial burden (reported as log[CFU/g or CFU/cm2 of the implant]) after 7 days of treatment comparing CarboCell formulation CC5 loaded with 30 mg/g clindamycin + 5 mg/g C2DA, 30 mg/g clindamycin + 5 mg/g DSF, or 25 mg/g clindamycin + 25 mg/g linezolid. B, Bacterial burden (in log[CFU/g or CFU/cm2 of the implant]). Tested formulations were CC3 with 80 mg/g clindamycin after 7 days or 14 days of treatment, or CC3 with 80 mg/g clindamycin + 5 mg/g DSF after 7 days or 14 days of treatment. See composition details in Table 1. Data were analyzed by 1-way ANOVA and are shown as mean ± SEM. *P ≤ .05, **P ≤ .01. Release data for antimicrobials can be found in Supplementary Table 7. Abbreviations: C2DA, cis-2-decenoic acid; CC, CarboCell; CFU, colony-forming unit; Clinda, clindamycin; DSF, diffusible signal factor, cis-11-methyl-2-dodecenoic acid; Linez, linezolid.](https://oup.silverchair-cdn.com/oup/backfile/Content_public/Journal/jid/230/3/10.1093_infdis_jiae139/1/m_jiae139f3.jpeg?Expires=1750196218&Signature=MLEBARogKnqDv09TS23OXiTUs6V-M5T7DeACiQ61lJXXeyuUq94vOxak1a6usQ7XkXuukMsYkmjI2~Qu99zkVPA80WyTh5muZVurJYASUFKCZq9Rai3m~Z3VmHmSJwbkxddeUKkynVqHKLv-tLDks4iGLvQRqTJ7GJ-zoION6-TJ4TFYj6FukhySEZTxmXOm1a837qZUv9yTlzFx~Ou~eM1nGvApoFLW2enGtgorAEgAvmO5T2r7w8xlw-u-fRcqc7CoKMvMeznTyk9Fpafns-tA4tcLwVDt4Mnhs-N20Y9CNXEb~q8kZ~1zO2K1nV94DRKUqNhM5j5G854mq32qCQ__&Key-Pair-Id=APKAIE5G5CRDK6RD3PGA)
Treatment of infected implant rat models by CarboCell-formulated clindamycin alone or in combination with DSF. A, Bacterial burden (reported as log[CFU/g or CFU/cm2 of the implant]) after 7 days of treatment comparing CarboCell formulation CC5 loaded with 30 mg/g clindamycin + 5 mg/g C2DA, 30 mg/g clindamycin + 5 mg/g DSF, or 25 mg/g clindamycin + 25 mg/g linezolid. B, Bacterial burden (in log[CFU/g or CFU/cm2 of the implant]). Tested formulations were CC3 with 80 mg/g clindamycin after 7 days or 14 days of treatment, or CC3 with 80 mg/g clindamycin + 5 mg/g DSF after 7 days or 14 days of treatment. See composition details in Table 1. Data were analyzed by 1-way ANOVA and are shown as mean ± SEM. *P ≤ .05, **P ≤ .01. Release data for antimicrobials can be found in Supplementary Table 7. Abbreviations: C2DA, cis-2-decenoic acid; CC, CarboCell; CFU, colony-forming unit; Clinda, clindamycin; DSF, diffusible signal factor, cis-11-methyl-2-dodecenoic acid; Linez, linezolid.
CarboCell-Mediated Antibiotic Treatment Is Effective at Eradicating S. aureus in a Translational Implant-Associated Osteomyelitis Porcine Model
All pigs were found to have established infection in bone, on implants, and in the S.C. tissue at the time of debridement and CarboCell administration (Supplementary Figure 5). Pigs displayed extensive swelling over the lesions, several had exudation of pus from the wound, and abscesses were found in both S.C. tissue and bone. Although antibiotics were not administered systemically in the porcine osteomyelitis model, all tested CarboCell-antibiotic formulations prevented S. aureus colonization of the new implants inserted at revision surgery. Here, the CC3 formulation was designed to release antimicrobials in vivo over 2 weeks and the CC2 formulation slightly faster (Figure 1D). Bone-residing pathogens were cleared for 4 of 5, 2 of 3, and 4 of 4 pigs in the CC3-clindamycin, CC3-clindamycin + DSF, and CC2-clindamycin treatment groups, respectively (Table 2 and Supplementary Figure 5). The CC2-clindamycin formulation furthermore cleared S. aureus in S.C. tissues of 3 of 4 animals (Table 2). It was not possible to distinguish whether there was an added benefit of DSF. Moreover, 2 pigs were treated prophylactically with levofloxacin + C2DA formulated in CC3; implants were protected from colonization and the bacterial burden in bone was reduced by 3 logs (Supplementary Figure 7). However, based on a reduced formation of osteoid from histological assessment, and reported chondrotoxicity of fluoroquinolones [35, 36], levofloxacin was not advanced further. Furthermore, 2 pigs that solely received debridement (without implant insertion at revision surgery and were euthanized 12 days postdebridement) both had positive IHC staining of S. aureus (Supplementary Figure 6 and Supplementary Table 6; unpublished data associated with the study by Blirup-Plum et al [7]).
Summary of Therapeutic Performance as Evaluated by Quantitative Bacteriology and Histopathology
Treatment Designation . | No. . | S. aureus Implants . | S. aureus Bone . | S. aureus Subcutaneous Tissue . | S. aureus IHC . | Neutrophil Counta . |
---|---|---|---|---|---|---|
CC3-clindamycin (ER)b | 5 | N N N N N | N N N N P | P P P N N | N N N N P | 0 0 1 0 10 |
CC2-clindamycin | 4 | N N N N | N N N N | N N P N | Nc N Pd N | 10 3 10 0 |
CC3-clindamycin + DSF (ER) | 3 | N N N | N N P | P P P | N N P | 1 0 3 |
Treatment Designation . | No. . | S. aureus Implants . | S. aureus Bone . | S. aureus Subcutaneous Tissue . | S. aureus IHC . | Neutrophil Counta . |
---|---|---|---|---|---|---|
CC3-clindamycin (ER)b | 5 | N N N N N | N N N N P | P P P N N | N N N N P | 0 0 1 0 10 |
CC2-clindamycin | 4 | N N N N | N N N N | N N P N | Nc N Pd N | 10 3 10 0 |
CC3-clindamycin + DSF (ER) | 3 | N N N | N N P | P P P | N N P | 1 0 3 |
Abbreviations: CC, CarboCell; DSF, diffusible signal factor; ER, extended release; N, negative; P, positive.
aThe neutrophil count indicates how many of 10 high-power fields at 40 × magnification had more than 5 neutrophils.
bER indicates in vivo release is expected to be extended (ie release clindamycin for a longer duration) in comparison to CC2.
cAbsence of Staphylococcus aureus, but Streptococcus dysgalactiae was detected.
dThis pig tested positive for presence of S. aureus, but bacteria were exclusively present in the hematoma of the bone cavity.
Summary of Therapeutic Performance as Evaluated by Quantitative Bacteriology and Histopathology
Treatment Designation . | No. . | S. aureus Implants . | S. aureus Bone . | S. aureus Subcutaneous Tissue . | S. aureus IHC . | Neutrophil Counta . |
---|---|---|---|---|---|---|
CC3-clindamycin (ER)b | 5 | N N N N N | N N N N P | P P P N N | N N N N P | 0 0 1 0 10 |
CC2-clindamycin | 4 | N N N N | N N N N | N N P N | Nc N Pd N | 10 3 10 0 |
CC3-clindamycin + DSF (ER) | 3 | N N N | N N P | P P P | N N P | 1 0 3 |
Treatment Designation . | No. . | S. aureus Implants . | S. aureus Bone . | S. aureus Subcutaneous Tissue . | S. aureus IHC . | Neutrophil Counta . |
---|---|---|---|---|---|---|
CC3-clindamycin (ER)b | 5 | N N N N N | N N N N P | P P P N N | N N N N P | 0 0 1 0 10 |
CC2-clindamycin | 4 | N N N N | N N N N | N N P N | Nc N Pd N | 10 3 10 0 |
CC3-clindamycin + DSF (ER) | 3 | N N N | N N P | P P P | N N P | 1 0 3 |
Abbreviations: CC, CarboCell; DSF, diffusible signal factor; ER, extended release; N, negative; P, positive.
aThe neutrophil count indicates how many of 10 high-power fields at 40 × magnification had more than 5 neutrophils.
bER indicates in vivo release is expected to be extended (ie release clindamycin for a longer duration) in comparison to CC2.
cAbsence of Staphylococcus aureus, but Streptococcus dysgalactiae was detected.
dThis pig tested positive for presence of S. aureus, but bacteria were exclusively present in the hematoma of the bone cavity.
Histopathological Evaluations Confirm Microbiological Findings
The macroscopic assessment showed that bone voids contained a hematoma (Figure 4A), often surrounded by a thin layer of fibrous tissue. Four of the 12 pigs had macroscopic signs of infection (pus and/or sequestrum) in relation to the bone void, which was bordered by a thicker layer of fibrous tissue (Figure 4A). CarboCell depots were identified in S.C. tissues and bone voids (Figure 4).
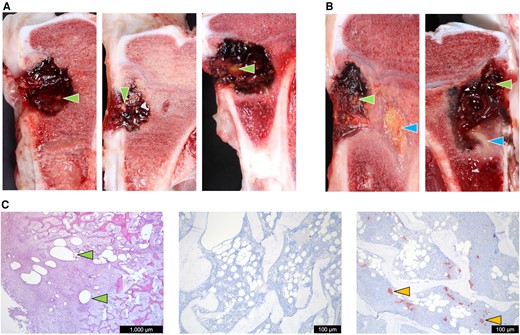
Pathological and histopathological investigations after CarboCell-formulated antibiotic treatment of the implant-associated osteomyelitis porcine model. A, Macropathological photographic examples of cross-sectioned pig tibiae from CC3-clindamycin (left), CC3-clindamycin + DSF (center), and CC2-clindamycin (right). B, Photographic examples of pigs presenting with sequestrate; CC3-clindamycin (left) and CC3-clindamycin + DSF (right). C, Left, an hematoxylin and eosin-stained histological section of the pathological bone area showing CarboCell depots at microscopic levels; center, an example of a Staphylococcus aureus-negative IHC staining of bone; and right, an example of a S. aureus-positive IHC staining of bone. Green arrows point to macroscopic and microscopic examples of CarboCell depots. Blue arrows point to sequestrae. Yellow arrows point to S. aureus aggregates. Release data of antimicrobials in each treatment group are shown in Supplementary Table 8. Quantified bacterial burden in logCFU/g at time of debridement and administration of antibiotic-formulated CarboCell, and the bacterial burden postmortem can be found in Supplementary Figure 5. Abbreviations: CC, CarboCell; CFU, colony-forming unit; DSF, diffusible signal factor, cis-11-methyl-2-dodecenoic acid; IHC, immunohistochemistry.
Histologically, closest to the bone void, the pathological bone area consisted of fibrous tissue of varying thickness intermingled with mononuclear cells, neutrophilic granulocytes, occasionally giant cells, and small amounts of necrotic bone debris. Behind this layer, osteoid and a few osteoclasts were observed. In some cases, osteoid was also present at bone void borders.
In 4 of 5 pigs treated with CC3-clindamycin, neutrophil granulocyte counts were below the infective cutoff value, and IHC for S. aureus was negative (Table 2). In the bones of animals with confirmed presence of pathogens, large amounts of necrotic bone and/or inflammatory cells were observed, sometimes organized as microabscesses. CarboCells were identified within the bone void, pathological bone area, and normal trabecular bone tissue, confirming the strategic placement envisioned in Figure 1A. The inflammatory cellular responses to CarboCells in bone tissue consisted mainly of fibroblasts and macrophages. In a few cases, neutrophilic granulocytes and giant cells were seen.
In general, bacteriological findings and IHC were in agreement regarding identification of S. aureus. In 1 pig from the CC2-clindamycin group, bacteria were identified histologically in bone tissue sections, while microbiological assays identified non-S. aureus colonies, identified as Streptococcus dysgalactiae. For another pig in the CC2-clindamycin group, S. aureus was found in the hematoma of the bone cavity but not in any bone samples; in agreement, IHC identified S. aureus in the bone void cavity but not in the bone. For S. aureus-positive samples, CFUs were isolated, and their sensitivity toward clindamycin was investigated by disk diffusion and compared to the wild type (Supplementary Methods). No colonies were less susceptible toward clindamycin relative to the wild type, suggesting no resistance had emerged (Supplementary Figure 8).
DISCUSSION
This study showed promising results of a new injectable, carbohydrate-based drug delivery system for the treatment of implant-associated osteomyelitis. Formulations of antimicrobials in the CarboCell delivery system were iteratively improved in the in vitro-matured biofilm infected-implant rat models. Lead formulations were then advanced to the 1-stage-revision–like porcine implant-associated osteomyelitis models, for which studies were designed without administration of systemic antibiotics to solely evaluate the therapeutic efficacy and potential of CarboCell formulations. Optimized CarboCell formulations provided complete pathogen eradication in 5 of 12 pigs. However, in an additional 7 pigs CFUs were found only in S.C. tissue, where the co-housing of pigs should potentially be considered when interpreting the microbiological result from soft tissue and fresh wounds. Importantly, for 9 of 12 pigs, all bone tissue was clear of pathogens and pathogen colonization was prevented in all 12 pigs’ implants, without the use of systemic antibiotics. These results suggest a potential to lower the reinfection rate of 1-stage revision strategies, which should be tested in future studies. To put the observed efficacy into perspective, it was previously shown that a commercially available gentamicin-eluting bone cement in the same porcine model could not clear pathogens (of the same strain and inoculum) when combined with debridement in any of the 6 pigs treated, and even allowed biofilm growth directly on the cement [7, 37]. Importantly, debridement alone was insufficient to eradicate S. aureus infection in this model (Supplementary Figure 6 and Supplementary Table 6), and clinical studies have shown that debridement alone often leads to infection recurrence [38].
The high infection control observed in the current study may thus partly be explained by the ability to inject into the trabecular bone by fine needles, which allows the distribution of antibiotics in challenging sites. In 2014, Giers et al showed that antibiotics eluted from bone cements located in a bone void did not distribute into deeper bone tissues [39], and others have shown that MBEC cannot be achieved via systemic recirculation from local delivery systems, as the achieved serum concentrations are insufficient [40–42]. This is problematic as S. aureus is known to spread into deeper bone tissues from the bone void [10]. A related issue may also be that calcium sulphate-based and other cement-based delivery systems are solids that provide an initial drug burst followed by slow drug release [43–45], whereas the in situ-formed CarboCell depots can be injected into deeper bone tissues and offer long-lasting, diffusion-dependent sustained drug release.
Other treatment approaches under development include hydrogels, which also aim to provide sustained local release of antibiotics [46, 47]. However, a loose polymer network in hydrogels makes it challenging to obtain long-term sustained release, which is often solved by complicated cross-linking or covalent binding of drug molecules to the polymer backbone to achieve sustained release [48, 49]. Nevertheless, a recent study demonstrated successful pathogen eradication in a sheep model by a gentamicin + vancomycin-loaded hydrogel, even though the hydrogel exhibited more than 60 % release after 24 hours in vitro [46]. Encouragingly, however, their study found an enhanced therapeutic efficacy relative to the standard of care in a 2-stage revision model with 2 consecutive administrations of the hydrogel (2 × 20 mL) in combination with systemic antibiotics.
The therapeutic efficacy of CarboCell formulations in the current study indirectly supports that effective antibiotic concentrations are achieved in the key locations required for the eradication of infection. However, future studies investigating the precise antibiotic concentration, locally and systemically over time, will be advantageous for optimizing dosing to avoid antimicrobial resistance development and for the clinical advancement of CarboCell formulations. Likewise, the spatial distribution of antibiotics surrounding each CarboCell depot should be investigated along with drug-tissue retention times and in situ clearance rates.
Further development of CarboCell formulations with broader pathogen coverage by combinations of antibiotics is highly attractive to advance treatment towards clinical evaluation. In future studies, combining CarboCell-antibiotic formulations with bone cements could be studied for an optimized osteomyelitis treatment. More investigations into bone regeneration, employing CarboCell technology for the sustained release of osteoinductive factors, or integrating osteoconductive excipients into CarboCell compositions, are also of interest for clinical development. Furthermore, other types of challenging infections, for example, surgical site infection and spondylodiscitis, may also benefit from CarboCell-mediated antimicrobial treatment and should be further investigated. Lastly, this study did not include a direct, parallel evaluation of a placebo control group and/or a systemic antibiotic treatment group, which would be advantageous in future studies. However, we believe that existing preclinical data [7, 10–16], and clinical reports on the challenges and concerns associated with long-term high-dose systemic antibiotics, support the potential for an improved local delivery technology.
Supplementary Data
Supplementary materials are available at The Journal of Infectious Diseases online (http://jid.oxfordjournals.org/). Supplementary materials consist of data provided by the author that are published to benefit the reader. The posted materials are not copyedited. The contents of all supplementary data are the sole responsibility of the authors. Questions or messages regarding errors should be addressed to the author.
Notes
Author contributions. A. F. M., N. L. H., E. S. C., T. L. A., J. R. H., and A. E. H. contributed conceptualization. A. F. M., N. L. H., L. A. K., J. K. M. B., K. T. H., T. E., T. B., H. G., L. K. J., J. R. H., and A. E. H. contributed methodology. A. F. M., N. L. H., E. S. C., J. R. H., and A. E. H. performed validation, formal analysis, investigation, and data curation. T. B., T. L. A., L. K. J., J. R. H., and A. E. H. contributed resources. A. F. M., N. L. H., J. R. H., and A. E. H. wrote the original draft. A. F. M., N. L. H., L. A. K., J. K. M. B., K. T. H., T. E., H. G., T. B., T. L. A., L. K. J., J. R. H., and A. E. H. reviewed and edited the manuscript. A. F. M., J. R. H., and A. E. H. contributed visualization. L. A. K., T. B., T. E., H. G., L. K. J., J. R. H., and A. E. H. contributed supervision. A. F. M., N. L. H., E. S. C., J. R. H., and A. E. H. contributed project administration. T. L. A., J. R. H., and A. E. H. acquired funding.
Acknowledgments. Special thanks go to Associate Professor Emeritus Bent Aalbæk (Copenhagen University), Professor Søren Molin, Janus Haagensen (Technical University of Denmark), and Nicholas Ditzel (University of Southern Denmark) for advice, analysis, methods, and models.
Financial support. This work was supported by the Novo Nordisk Foundation (Distinguished Innovator grant number 0080941 to A. E. H.); and the Innovation Fund Denmark (Innoexplorer grant number 1048-00027B to A. E. H.).
References
Author notes
Potential conflicts of interest. A. E. H., J. R. H., and T. L. A. are coinventors on patents covering the CarboCell technology. All other authors report no potential conflicts.
All authors have submitted the ICMJE Form for Disclosure of Potential Conflicts of Interest. Conflicts that the editors consider relevant to the content of the manuscript have been disclosed.