-
PDF
- Split View
-
Views
-
Cite
Cite
Cécile Alanio, Anurag Verma, Divij Mathew, Sigrid Gouma, Guanxiang Liang, Thomas Dunn, Derek A Oldridge, JoEllen Weaver, Leticia Kuri-Cervantes, M Betina Pampena, Michael R Betts, Ronald G Collman, Frederic D Bushman, Nuala J Meyer, Scott E Hensley, Daniel Rader, E John Wherry, The UPenn COVID Processing Unit , Cytomegalovirus Latent Infection is Associated with an Increased Risk of COVID-19-Related Hospitalization, The Journal of Infectious Diseases, Volume 226, Issue 3, 1 August 2022, Pages 463–473, https://doi.org/10.1093/infdis/jiac020
- Share Icon Share
Abstract
Some risk factors for severe coronavirus disease 2019 (COVID-19) have been identified, including age, race, and obesity. However, 20%–50% of severe cases occur in the absence of these factors. Cytomegalovirus (CMV) is a herpesvirus that infects about 50% of all individuals worldwide and is among the most significant nongenetic determinants of immune system. We hypothesized that latent CMV infection might influence the severity of COVID-19. Our analyses demonstrate that CMV seropositivity is associated with more than twice the risk of hospitalization due to severe acute respiratory syndrome coronavirus 2 (SARS-CoV-2) infection. Immune profiling of blood and CMV DNA quantitative polymerase chain reaction in a subset of patients for whom respiratory tract samples were available revealed altered T-cell activation profiles in absence of extensive CMV replication in the upper respiratory tract. These data suggest a potential role for CMV-driven immune perturbations in affecting the outcome of SARS-CoV-2 infection and may have implications for the discrepancies in COVID-19 severity between different human populations.
Coronavirus disease 2019 (COVID-19) pandemic has resulted in nearly 260 million infections and >5 million deaths worldwide by November 2021, with notable differences in disease burden across continents and countries [1]. After infection with severe acute respiratory syndrome coronavirus 2 (SARS-CoV-2), patients with COVID-19 present with a broad spectrum of clinical manifestations, ranging from asymptomatic infections or mild disease to severe disease requiring hospitalization and mechanical ventilation [2]. The case fatality rate in patients with acute respiratory distress syndrome (ARDS), a common consequence of severe COVID-19, can be as high as 10%–50% [3]. Several risk factors, including age, obesity, hypertension, and diabetes, are associated with increased risk of COVID-19 severity [4, 5]. In addition, the incidence of COVID-19 severity is disproportionally higher among individuals of African ancestry [6]. However, 20%–50% of severe cases occur in the absence of these factors [7]. Variation in immune response is likely involved in the distinct clinical manifestations of COVID-19, but other than age, features of immune history influencing the manifestations of COVID-19 remain poorly understood.
Cytomegalovirus (CMV) is a herpesvirus infecting approximately 50% of individuals worldwide. CMV seroprevalence increases with age, as well as in socioeconomically disadvantaged groups and nonwhite populations [8–10]. CMV remains latent after the acute phase of initial infection, and is thought to reactivate regularly, leading to a “smoldering” latent infection [8]. Latent CMV infection is among the most prominent nongenetic determinants of immune set point, influencing responses to future infections or vaccinations and shaping distribution of innate and adaptive immune cell populations [9–13]. Latent CMV infection is associated with increases in subsets of natural killer (NK) cells expressing key NK receptors and large expansion of effectorlike T cells, particularly CD45RA+CD27− late differentiated effector memory reexpressing CD45RA (EMRA) CD4+ and CD8+ T cells [9, 14]. These effectorlike lymphocyte populations are involved in long-term control of active CMV replication [14].
One key feature of severe SARS-CoV-2 infection is profound lymphopenia preferentially affecting NK cells and CD8+ T-cell populations, including the same cytotoxic lymphocytes altered by CMV infection [15]. However, the potential connection between SARS-CoV-2 and CMV infections has not previously been evaluated. Primary CMV infection and reactivation in immunocompetent individuals are generally asymptomatic and self-limited, although they occasionally can result in mononucleosislike syndrome or hepatitis. However, in immunocompromised patients, such as organ or hematopoietic transplant recipients, CMV reactivation can cause severe complications [16, 17]. Similarly, CMV reactivation can be a complication of critical illness including sepsis and other diseases requiring intensive care unit (ICU) admission [16]. It is unclear whether CMV reactivation occurs in the context of severe SARS-CoV-2 infection or whether smoldering CMV infection influences the pathogenesis of COVID-19. CMV replication predominates in the lung, a major reservoir for CMV, and local reactivation may cause lung injury and/or result in complications associated with CMV and critical illness [18]. Thus, CMV infection has the potential to shape the course of SARS-CoV-2 infection, either because of CMV reactivation or due to the broader reshaping of cytotoxic lymphocyte populations.
MATERIALS AND METHODS
Study Design
The Penn Medicine BioBank (PMBB) cohort in our study refers to 984 samples selected from approximately 70 000 samples collected before the pandemic (Supplementary Table 1). The PMBB cohort is approved under institutional review board protocol 813913 and supported by the Perelman School of Medicine at University of Pennsylvania. PMBB participants consented to the retrospective use of electronic health records for research. Using these records, we identified 246 SARS-CoV-2–positive cases (based on reverse-transcription [RT] quantitative polymerase chain reaction [qPCR]) among participants who were symptomatic or not symptomatic for COVID-19. We then selected 3 controls (participants negative for SARS-CoV-2 with RT-qPCR) for each positive case, matching participants by age, sex, and race. This approach identified approximately 1200 samples. Serum samples were available for most but not all participants identified, resulting in a final cohort of 246 RT-qPCR SARS-CoV-2–positive and 738 RT-qPCR SARS-CoV-2 negative participants, which that we refer to here as the PMBB cohort. Serum samples were collected from the PMBB cohort between August 2013 and March 2020. Because this study was retrospective, we cannot exclude the possibility that some CMV-seronegative individuals may convert to being seropositive over time, though giving the yearly seroconversion rate for CMV in adults [9, 19], we expect this would be a small effect.
The Molecular Epidemiology of SepsiS in the ICU (MESSI) cohort study (protocol 808542) included patients with COVID-19 prospectively as they were admitted to the Hospital of the University of Pennsylvania with a SARS-CoV-2–positive result. Patients or their proxies, if the patients were incapacitated owing to critical illness, provided informed consent. Healthy donors were adults with no prior diagnosis of, or recent symptoms consistent with, COVID-19. Recovered donors were adults with a prior positive COVID-19 RT polymerase chain reaction (RT-qPCR) test by self-report who met the definition of recovery by the Centers for Disease Control and Prevention. All donors in the COVID-19 MESSI cohort were recruited between March and August 2020 [15]. Respiratory tract samples for CMV qPCR included endotracheal aspirate or nasopharyngeal or oropharyngeal swab samples obtained after informed consent (institutional review board protocol 823392). For the ARDS cohort, patients were also enrolled in the MESSI cohort study [20]. ARDS was defined in accordance with the Berlin definition [21]
Serology
CMV serology was performed on human serum or plasma samples using CMV immunoglobulin (Ig) G enzyme-linked immunosorbent assay (ELISA) kit (Abcam; ab108724), according to the manufacturer’s instructions. We screened 20 samples with or without heat inactivation. No significant changes were observed after heat inactivation. Therefore, all samples in our study were heat inactivated at 56°C for 1 hour before ELISA to ensure that COVID-19 and non–COVID-19 samples were treated identically. All samples were assayed in duplicate. The limit of detection for ELISA antibody titers was set at 20 standard units after internal calibration.
CMV qPCR
DNA was extracted from respiratory tract samples (PowerSoil kit; Qiagen). qPCR was performed using TaqMan Fast Universal PCR (Thermo Fisher Scientific) and a QuantStudio 3 Real Time PCR System (Applied Biosystems) with the following program: 20 seconds at 95°C for 1 cycle and 40 cycles of 95°C for 3 seconds and 60°C for 30 seconds. Each reaction contained 900 nmol/L of each primer and a 250-nmol/L probe. The sequences of primers and probe (CMV-F, CATGAAGGTCTTTGCYCAGTAC; CMV-R, GGCCAAAGTGTAGGCTRCAATAG; and CMV-P, FAM/TGGCCCGTAGGTCATCCACACTAGG/TAMRA) have been described elsewhere [22]. qPCR replicates were performed in triplicate, and average genome copy numbers were used.
Activation-Induced Marker Expression Assay
The activation-induced marker (AIM) assay was performed as described elsewhere [23] with a CMV peptide pool (code 3619-1; Mabtech) for 24 hours.
Statistical Analyses
CMV IgG antibody titers were log2 transformed for statistical analysis. To examine the impact of CMV, we performed logistic and multiple linear regression analyses for CMV serostatus and CMV IgG levels, respectively. Odds ratios (ORs) were calculated using the R package finalfit. Uniform Manifold Approximation and Projection (UMAP) was overlaid with CMV serostatus, as described elsewhere [15].
RESULTS
Association of CMV Seropositivity With Increased COVID-19 Risk, Independent of Demographics
To interrogate the impact of previous CMV infection on SARS-CoV-2 infection and severity, we compared CMV IgG-based serostatus in prepandemic serum samples from 984 individuals in the PMBB, a disease-agnostic cohort of approximately 70 000 participants. These 984 individuals had a subsequent SARS-CoV-2 RT-PCR test with 246 positive and 738 negative results . Given potential confounding biases for SARS-CoV-2 infection with patient demographic characteristics, we designed a matched case-control analysis. For each individual with PCR-confirmed SARS-CoV-2 (RT-PCR positive), we selected individuals with RT-PCR–negative results, matching sex, age, and self-reported race/ethnicity.
The median age of the 984 donors was 54 years (standard deviation, 16 years) (Table 1). Patients were predominantly white (60%) or African American (23%), and 41% were male. Among these 984 donors, 617 (62.7%) were CMV seropositive. Overall, SARS-CoV-2 infection was more frequent among CMV-seropositive than among CMV-seronegative patients (176 of 617 [28.5%] vs 70 of 367 [19%]; P < .001) (Figure 1A). To quantify the impact of CMV seropositivity on the occurrence of SARS-CoV-2 infection, we performed a binomial logistic regression while adjusting for age, sex, and self-reported race. This analysis revealed that CMV seropositivity was associated with an increased risk of SARS-CoV-2 infection, with an OR of 1.7 (95% confidence interval [CI], 1.24–2.33; P = .001) (Figure 1B and Table 1). Of note, socioeconomic status is often associated with CMV infection and/or seropositivity [10]. Although socioeconomic information was not available for the current cohort, it will be interesting to examine in future how such variables contribute to differences in SARS-CoV-2 infection status.
Predictors Associated With Severe Acute Respiratory Syndrome Coronavirus 2 Infection Status Among 984 Patients in the Penn Medicine BioBank Cohort
Predictors . | Patients, No. (%)a . | P Valueb . | |
---|---|---|---|
SARS-CoV-2 Negative . | SARS-CoV-2 Positive . | ||
Age, median (SD), y | 52.6 (15.5) | 51.7 (16.1) | .45 |
Sex | |||
Female | 438 (59.3) | 146 (59.3) | >.99 |
Male | 300 (40.7) | 100 (40.7) | |
Self-reported race | |||
American Indian | 2 (0.3) | .33 | |
Asian | 24 (3.3) | 12 (4.9) | |
African American | 167 (22.6) | 59 (24.0) | |
East Indian | 6 (0.8) | 2 (0.8) | |
Hispanic Latino/black | 7 (0.9) | 1 (0.4) | |
Hispanic Latino/white | 24 (3.3) | 12 (4.9) | |
Other | 22 (3.0) | 13 (5.3) | |
Patient declined to respond | 8 (1.1) | 3 (1.2) | |
Unknown | 25 (3.4) | 8 (3.3) | |
White | 453 (61.4) | 135 (54.9) | |
Pacific Island | 1 (0.4) | ||
CMV serostatus | |||
Negative | 297 (40.2) | 70 (28.5) | .001 |
Positive | 441 (59.8) | 176 (71.5) |
Predictors . | Patients, No. (%)a . | P Valueb . | |
---|---|---|---|
SARS-CoV-2 Negative . | SARS-CoV-2 Positive . | ||
Age, median (SD), y | 52.6 (15.5) | 51.7 (16.1) | .45 |
Sex | |||
Female | 438 (59.3) | 146 (59.3) | >.99 |
Male | 300 (40.7) | 100 (40.7) | |
Self-reported race | |||
American Indian | 2 (0.3) | .33 | |
Asian | 24 (3.3) | 12 (4.9) | |
African American | 167 (22.6) | 59 (24.0) | |
East Indian | 6 (0.8) | 2 (0.8) | |
Hispanic Latino/black | 7 (0.9) | 1 (0.4) | |
Hispanic Latino/white | 24 (3.3) | 12 (4.9) | |
Other | 22 (3.0) | 13 (5.3) | |
Patient declined to respond | 8 (1.1) | 3 (1.2) | |
Unknown | 25 (3.4) | 8 (3.3) | |
White | 453 (61.4) | 135 (54.9) | |
Pacific Island | 1 (0.4) | ||
CMV serostatus | |||
Negative | 297 (40.2) | 70 (28.5) | .001 |
Positive | 441 (59.8) | 176 (71.5) |
Abbreviations: CMV, cytomegalovirus; SARS-CoV-2, severe acute respiratory syndrome coronavirus 2; SD, standard deviation.
Data represent no. (%) of patients unless otherwise specified. SARS-CoV-2 results are based on reverse-transcription polymerase chain reaction testing.
P values based on binomial logistic regression analysis.
Predictors Associated With Severe Acute Respiratory Syndrome Coronavirus 2 Infection Status Among 984 Patients in the Penn Medicine BioBank Cohort
Predictors . | Patients, No. (%)a . | P Valueb . | |
---|---|---|---|
SARS-CoV-2 Negative . | SARS-CoV-2 Positive . | ||
Age, median (SD), y | 52.6 (15.5) | 51.7 (16.1) | .45 |
Sex | |||
Female | 438 (59.3) | 146 (59.3) | >.99 |
Male | 300 (40.7) | 100 (40.7) | |
Self-reported race | |||
American Indian | 2 (0.3) | .33 | |
Asian | 24 (3.3) | 12 (4.9) | |
African American | 167 (22.6) | 59 (24.0) | |
East Indian | 6 (0.8) | 2 (0.8) | |
Hispanic Latino/black | 7 (0.9) | 1 (0.4) | |
Hispanic Latino/white | 24 (3.3) | 12 (4.9) | |
Other | 22 (3.0) | 13 (5.3) | |
Patient declined to respond | 8 (1.1) | 3 (1.2) | |
Unknown | 25 (3.4) | 8 (3.3) | |
White | 453 (61.4) | 135 (54.9) | |
Pacific Island | 1 (0.4) | ||
CMV serostatus | |||
Negative | 297 (40.2) | 70 (28.5) | .001 |
Positive | 441 (59.8) | 176 (71.5) |
Predictors . | Patients, No. (%)a . | P Valueb . | |
---|---|---|---|
SARS-CoV-2 Negative . | SARS-CoV-2 Positive . | ||
Age, median (SD), y | 52.6 (15.5) | 51.7 (16.1) | .45 |
Sex | |||
Female | 438 (59.3) | 146 (59.3) | >.99 |
Male | 300 (40.7) | 100 (40.7) | |
Self-reported race | |||
American Indian | 2 (0.3) | .33 | |
Asian | 24 (3.3) | 12 (4.9) | |
African American | 167 (22.6) | 59 (24.0) | |
East Indian | 6 (0.8) | 2 (0.8) | |
Hispanic Latino/black | 7 (0.9) | 1 (0.4) | |
Hispanic Latino/white | 24 (3.3) | 12 (4.9) | |
Other | 22 (3.0) | 13 (5.3) | |
Patient declined to respond | 8 (1.1) | 3 (1.2) | |
Unknown | 25 (3.4) | 8 (3.3) | |
White | 453 (61.4) | 135 (54.9) | |
Pacific Island | 1 (0.4) | ||
CMV serostatus | |||
Negative | 297 (40.2) | 70 (28.5) | .001 |
Positive | 441 (59.8) | 176 (71.5) |
Abbreviations: CMV, cytomegalovirus; SARS-CoV-2, severe acute respiratory syndrome coronavirus 2; SD, standard deviation.
Data represent no. (%) of patients unless otherwise specified. SARS-CoV-2 results are based on reverse-transcription polymerase chain reaction testing.
P values based on binomial logistic regression analysis.
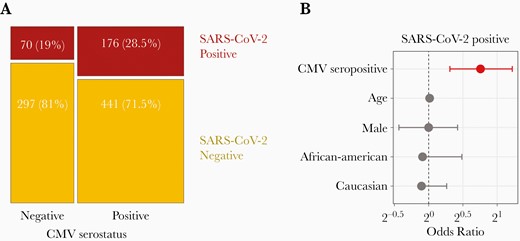
Cytomegalovirus (CMV) seropositivity is associated with an increased risk of testing positive for severe acute respiratory syndrome coronavirus 2 (SARS-CoV-2). A, Mosaic plot showing the numbers and proportions of individuals with SARS-CoV-2-positive or SARS-CoV-2–negative reverse-transcription polymerase chain reaction results as a function of CMV serostatus. B, Odds ratios and 95% confidence intervals for testing positive for SARS-CoV-2 (sample size, n = 984); red indicates a P value <.05, as indicated in Table 1.
Increased Risk of COVID-19–Related Hospitalization in CMV-Seropositive Patients
To interrogate the impact of CMV serostatus on COVID-19 outcome, we further stratified this cohort by severity of infection. Specifically, we categorized individuals into 4 groups: group 0, patients with a negative test result for COVID-19 (n = 738; group 1, patients with a positive COVID-19 result who were not hospitalized (n = 167); group 2, patients with a positive result who were hospitalized but not in the ICU (n = 49); and group 3, patients with a positive result who were hospitalized in the ICU (n = 30). CMV-seropositive individuals comprised 59.7% of group 0, 65.8% of group 1, 87.8% of group 2, and 76.7% of group 3, indicating an association of CMV seropositivity with more severe COVID-19 (P < .001 for groups 0–3; P = .01 for groups 1–3) (Figure 2A).
![Cytomegalovirus (CMV) seropositivity is associated with an increased risk of hospitalization related to coronavirus disease 2019 (COVID-19). A, Frequency of CMV seropositivity or seronegativity among individuals testing positive for COVID-19, including those not hospitalized (group [Gp] 1; n = 167), those hospitalized but not in the intensive care unit (ICU) (Gp 2; n = 49), and those hospitalized in the ICU (Gp 3; n = 30). P value is based on binomial logistic regression including patient age, sex, and self-reported race. B, Mosaic plot showing the numbers and proportions of hospitalizations due to COVID-19 as a function of CMV serostatus (sample size, n = 246). C, Odds ratios and 95% confidence intervals for hospitalization related to COVID-19 (sample size, n = 246); red indicates a P value <.05, as indicated in Table 2. D, Mean CMV immunoglobulin (Ig) G levels (in standard units [SU]) and standard deviations (SDs) as a function of COVID-19 severity in CMV-seropositive individuals (sample size, n = 246).](https://oup.silverchair-cdn.com/oup/backfile/Content_public/Journal/jid/226/3/10.1093_infdis_jiac020/1/m_jiac020_fig2.jpeg?Expires=1749900302&Signature=Bsn6J8QACkHqpWix5I0ASPyc723l6aUXz2kVmwWqcOI~mpn1zJ2qk8QPnSNj5A1XbwkWjQnm0tn~mClSYZ0q-C4WP0Zv5d0hNX20wzQsZo4vBXmMtW9FHu4JzGMmVvVZ7CpHnNwqx4ao0Q4VGSLr~g75DHbtbIM2s~KL2MhYHEwcHvvn3e8bjJ9uAlx7ATZ-VvZRtaWWgx-TSzBQ8Ho0lEi66Xybziom1pLZZlxNIvvXuS6RTkx6UpOnHsk6wFsiBKvU3P-NZkn8TYDaVZSFIunWxvzYBEtZth73F~eBi47Lyro7v1bB57GI5A7liKAVNCnN9WZr94Jgo2RukL6TOQ__&Key-Pair-Id=APKAIE5G5CRDK6RD3PGA)
Cytomegalovirus (CMV) seropositivity is associated with an increased risk of hospitalization related to coronavirus disease 2019 (COVID-19). A, Frequency of CMV seropositivity or seronegativity among individuals testing positive for COVID-19, including those not hospitalized (group [Gp] 1; n = 167), those hospitalized but not in the intensive care unit (ICU) (Gp 2; n = 49), and those hospitalized in the ICU (Gp 3; n = 30). P value is based on binomial logistic regression including patient age, sex, and self-reported race. B, Mosaic plot showing the numbers and proportions of hospitalizations due to COVID-19 as a function of CMV serostatus (sample size, n = 246). C, Odds ratios and 95% confidence intervals for hospitalization related to COVID-19 (sample size, n = 246); red indicates a P value <.05, as indicated in Table 2. D, Mean CMV immunoglobulin (Ig) G levels (in standard units [SU]) and standard deviations (SDs) as a function of COVID-19 severity in CMV-seropositive individuals (sample size, n = 246).
Predictors Associated With Coronavirus Disease 2019–Related Hospitalization
Predictors . | Patients, No. (%)a . | P Valueb . | |
---|---|---|---|
Not Hospitalized . | Hospitalized . | ||
Age, mean (SD), y | 47.2 (14.4) | 61.1 (15.4) | <.001 |
Sex | |||
Female | 105 (62.9) | 41 (51.9) | .13 |
Male | 62 (37.1) | 38 (48.1) | |
Self-reported race | |||
Asian | 9 (5.4) | 3 (3.8) | >.99 |
African American | 39 (23.4) | 20 (25.3) | |
East Indian | 1 (0.6) | 1 (1.3) | |
Hispanic Latino/black | 1 (0.6) | ||
Hispanic Latino/white | 8 (4.8) | 4 (5.1) | |
Other | 9 (5.4) | 4 (5.1) | |
Pacific Island | 1 (0.6) | ||
Patient declined to respond | 2 (1.2) | 1 (1.3) | |
Unknown | 5 (3.0) | 3 (3.8) | |
White | 92 (55.1) | 43 (54.4) | |
CMV serostatus | |||
Negative | 57 (34.1) | 13 (16.5) | .007 |
Positive | 110 (65.9) | 66 (83.5) |
Predictors . | Patients, No. (%)a . | P Valueb . | |
---|---|---|---|
Not Hospitalized . | Hospitalized . | ||
Age, mean (SD), y | 47.2 (14.4) | 61.1 (15.4) | <.001 |
Sex | |||
Female | 105 (62.9) | 41 (51.9) | .13 |
Male | 62 (37.1) | 38 (48.1) | |
Self-reported race | |||
Asian | 9 (5.4) | 3 (3.8) | >.99 |
African American | 39 (23.4) | 20 (25.3) | |
East Indian | 1 (0.6) | 1 (1.3) | |
Hispanic Latino/black | 1 (0.6) | ||
Hispanic Latino/white | 8 (4.8) | 4 (5.1) | |
Other | 9 (5.4) | 4 (5.1) | |
Pacific Island | 1 (0.6) | ||
Patient declined to respond | 2 (1.2) | 1 (1.3) | |
Unknown | 5 (3.0) | 3 (3.8) | |
White | 92 (55.1) | 43 (54.4) | |
CMV serostatus | |||
Negative | 57 (34.1) | 13 (16.5) | .007 |
Positive | 110 (65.9) | 66 (83.5) |
Abbreviations: CMV, cytomegalovirus; SD, standard deviation.
Data represent no. (%) of patients unless otherwise specified. Among 246 patients with a positive test result for coronavirus disease 2019, 167 were not hospitalized, 49 were hospitalized but not in the intensive care unit (ICU), and 30 were hospitalized in the ICU. “Hospitalized” column includes both ICU and non-ICU groups.
P values based on binomial logistic regression analysis.
Predictors Associated With Coronavirus Disease 2019–Related Hospitalization
Predictors . | Patients, No. (%)a . | P Valueb . | |
---|---|---|---|
Not Hospitalized . | Hospitalized . | ||
Age, mean (SD), y | 47.2 (14.4) | 61.1 (15.4) | <.001 |
Sex | |||
Female | 105 (62.9) | 41 (51.9) | .13 |
Male | 62 (37.1) | 38 (48.1) | |
Self-reported race | |||
Asian | 9 (5.4) | 3 (3.8) | >.99 |
African American | 39 (23.4) | 20 (25.3) | |
East Indian | 1 (0.6) | 1 (1.3) | |
Hispanic Latino/black | 1 (0.6) | ||
Hispanic Latino/white | 8 (4.8) | 4 (5.1) | |
Other | 9 (5.4) | 4 (5.1) | |
Pacific Island | 1 (0.6) | ||
Patient declined to respond | 2 (1.2) | 1 (1.3) | |
Unknown | 5 (3.0) | 3 (3.8) | |
White | 92 (55.1) | 43 (54.4) | |
CMV serostatus | |||
Negative | 57 (34.1) | 13 (16.5) | .007 |
Positive | 110 (65.9) | 66 (83.5) |
Predictors . | Patients, No. (%)a . | P Valueb . | |
---|---|---|---|
Not Hospitalized . | Hospitalized . | ||
Age, mean (SD), y | 47.2 (14.4) | 61.1 (15.4) | <.001 |
Sex | |||
Female | 105 (62.9) | 41 (51.9) | .13 |
Male | 62 (37.1) | 38 (48.1) | |
Self-reported race | |||
Asian | 9 (5.4) | 3 (3.8) | >.99 |
African American | 39 (23.4) | 20 (25.3) | |
East Indian | 1 (0.6) | 1 (1.3) | |
Hispanic Latino/black | 1 (0.6) | ||
Hispanic Latino/white | 8 (4.8) | 4 (5.1) | |
Other | 9 (5.4) | 4 (5.1) | |
Pacific Island | 1 (0.6) | ||
Patient declined to respond | 2 (1.2) | 1 (1.3) | |
Unknown | 5 (3.0) | 3 (3.8) | |
White | 92 (55.1) | 43 (54.4) | |
CMV serostatus | |||
Negative | 57 (34.1) | 13 (16.5) | .007 |
Positive | 110 (65.9) | 66 (83.5) |
Abbreviations: CMV, cytomegalovirus; SD, standard deviation.
Data represent no. (%) of patients unless otherwise specified. Among 246 patients with a positive test result for coronavirus disease 2019, 167 were not hospitalized, 49 were hospitalized but not in the intensive care unit (ICU), and 30 were hospitalized in the ICU. “Hospitalized” column includes both ICU and non-ICU groups.
P values based on binomial logistic regression analysis.
To quantify the impact of CMV seropositivity on hospitalization rate for COVID-19 infection, we evaluated CMV serostatus in hospitalized patients (groups 2 and 3). Among CMV-seropositive patients, compared with seronegative patients, there were greater numbers and higher proportions of patients with COVID-19 requiring hospitalization (66 of 176 [37.5%] vs 13 of 70 [18.5%]) (Figure 2B and Table 2). When we calculated the odds of being hospitalized with COVID-19, adjusting for age, self-reported race, and sex, CMV seropositivity was associated with an OR of 2.63 (95% CI, 1.37–5.35; P = .005) (Figure 2C). Age was also significantly associated (OR, 1.06 [95% CI, 1.04–1.09]; P < .001) in our multivariate model. Finally, quantitative CMV IgG titers in CMV-seropositive individuals were not associated with severity (Figure 2D). Together, our data suggest a mechanism in which CMV serostatus itself or the global imprint of CMV on the immune landscape, rather than levels of antibody to CMV, is associated with COVID-19 infection severity.
CMV Reactivation and EMRA T-Cell Activation in Patients With COVID-19
Quantification of COVID-19 severity includes measures of lung injury, and more severe SARS-CoV-2 infection can lead to ARDS in patients with COVID-19. To investigate whether CMV seropositivity was a general feature of more severe critical illness beyond SARS-CoV-2 infection, we measured CMV seroprevalence in an additional cohort of 44 patients with non–COVID-19 ARDS, as well as in healthy donors (n = 24), patients who had recovered from earlier mild COVID-19 (n = 27), and hospitalized adult patients with COVID-19 (n = 127) (MESSI and ARDS cohorts) (Supplementary Table 1) [15]. CMV seropositivity was 58.3% in healthy donors, 33.3% in recovered donors, and 76.4% in hospitalized patients with COVID-19. Although there were trends for CMV seroprevalence to be higher for patients with COVID-19 versus healthy controls (P = .07) and for recovered patients versus healthy controls (P = .08), the difference in CMV seropositivity between hospitalized patients with COVID-19 and nonhospitalized and recovered patients with mild COVID-19 was highly significant (P < .001) (Figure 3A). Among patients with ARDS, CMV seropositivity was 70.5% in patients without COVID-19, compared with 76.4% in those with COVID-19.
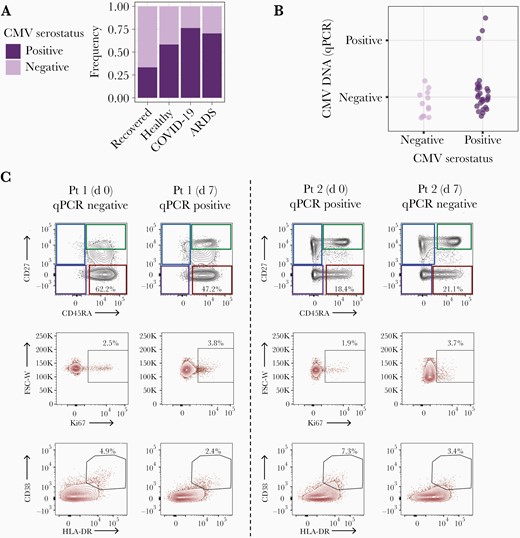
Cytomegalovirus (CMV) reactivation and effector memory reexpressing CD45RA (EMRA) T-cell activation in patients with coronavirus disease 2019 (COVID-19). A, Frequency of CMV seropositivity and seronegativity among patients recovered from mild COVID-10 (n = 27), healthy controls (n = 24), patients with COVID-19 (n = 127), and patients with non–COVID-19 acute respiratory distress syndrome (ARDS) (n = 44). Of note, these models were adjusted by age and sex but not by self-reported race, as this information was not available for healthy donors. B, CMV DNA quantitative polymerase chain reaction (qPCR) as a function of CMV serostatus, in respiratory tract samples from 40 patients with COVID-19. C, Longitudinal assessment of proportions of EMRA CD8+ T cells (red squares) using CD45RA/CD27, as well as Ki67+ and CD38+HLA-DR+ EMRA CD8+ T cells in 2 patients with CMV reactivation in the upper respiratory tract. The CMV DNA qPCR status at the time of sampling is indicated. Abbreviation: FSC-W, forward scatter width; Pt, patient.
Prior studies have shown CMV reactivation and presence of active viral replication in ARDS patients associating with severe lung damage [21, 24, 25]. To evaluate the rate of CMV reactivation in the respiratory tract of patients with COVID-19, we used qPCR to measure CMV DNA in nasopharyngeal, oropharyngeal, and endotracheal aspirates from 40 patients with COVID-19 with samples available; the rate of CMV seropositivity was 70% in these patients (28 of 40), similar to the proportion in the overall cohort. Among 28 seropositive patients, 3 had ≥1 sample positive for CMV DNA by qPCR (11%) (Figure 3B). This rate is below that observed in previous studies in blood samples from ICU patients without COVID-19 [24]. However, it is difficult to compare reactivation rates in the upper respiratory tract versus the blood, and prevalence in the respiratory tracts of healthy donors is unclear. All 3 patients had severe COVID-19 disease according to the 8-point World Health Organization ordinal scale (Supplementary Figure 1A)[26]. We examined available flow data from 2 of these patients who showed robust EMRA populations but relatively modest CD8+ T-cell activation (Figure 3C). With data available for only 2 patients with known CMV viral reactivation, however, it was not possible to link T-cell activation profiles to viral reactivation. Nevertheless, these results indicate that CMV seropositivity is associated with severe SARS-CoV-2 infection independently of viral reactivation, possibly through systemic immune perturbation.
Association Between CMV Seropositivity in Patients With COVID-19 and EMRA T-Cell Activation
To systematically examine immune perturbations induced by CMV in patients with COVID-19, we took advantage of a published immune profiling data set from our second independent cohort [15] and further applied a linear mixed model, adjusting for age and sex to identify differences based on CMV serostatus. In this previous study, our group profiled approximately 200 immune features and >30 clinical features from the donors described above—healthy, recovered, and with COVID-19 [15]. Similar to what was previously observed in healthy donors [9], CMV seropositivity in patients with COVID-19 was associated with an increase in late differentiated EMRA CD4+ and CD8+ T cells and a reduction in effector memory 1 cells (CD45RA−CD27+CCR7−) (Figure 4A). CMV seropositivity was positively associated with blood group O, high-sensitivity C-reactive protein, and African American ethnicity and negatively associated with white ethnicity, immunosuppression, and past venous thromboembolytic events (Supplementary Figure 1B).
![Cytomegalovirus (CMV) seropositivity in patients with coronavirus disease 2019 (COVID-19) is associated with effector memory reexpressing CD45RA (EMRA) T-cell activation. A, Quantification of the effect of CMV seropositivity on the abundance of circulating immune cells obtained from donors with COVID-19 (n = 127), estimated in a linear mixed model with a log-transformed immunophenotype as the response, controlled for age, sex, and self-reported race, then transformed to the original scale (with 99% confidence intervals [CIs] adjusted for false coverage). Abbreviations: cTfh, conventional T follicular helper cell; EM1, effector memory 1; PD-1, programmed cell death 1 protein. B, Unmodified Uniform Manifold Approximation and Projection (UMAP) projection of patients recovered from COVID-19 (n = 27), healthy controls (n = 24), and patients with COVID-19 (n = 127), as described elsewhere [15], using single-positive populations of all immune cell subsets. Individuals are color coded by their CMV serostatus; black square indicates cluster of CMV-seropositive patients (n = 20) further examined in D. Abbreviation: NA, non available. C, UMAP model illustrated as described elsewhere [15], with feature-weighted density contours overlaid on patients with COVID-19 (n = 127). The illustrated gradient vector was computed according to the direction of maximum Pearson correlation for the flow cytometric feature of interest (here, EMRA CD8+ T cells) and weighted in length according to the strength of the resulting Pearson coefficient. Red lines indicate high EMRA; blue lines, low EMRA T-cell proportions. The intensity of the dots represents the z score, and the dotted line is drawn perpendicular to the gradient vector. Triangles represent deaths within 90 days of admission, as reported elsewhere [15]. D, Immunophenotypes (n = 279) significantly associated with the cluster of CMV-seropositive patients (sample size, n = 20) displayed in B, as estimated in a linear mixed model with a log-transformed immunophenotype as the response, controlled for age, sex, and self-reported race and then transformed to the original scale (with 99% CIs adjusted for false coverage).](https://oup.silverchair-cdn.com/oup/backfile/Content_public/Journal/jid/226/3/10.1093_infdis_jiac020/1/m_jiac020_fig4.jpeg?Expires=1749900302&Signature=wgqOKJGvuwBQDH9PujWSbyUSYlZY7i~g67iGGUK0sxYKwzG8hQgbAamgIvWyushs32c64mbUOaBzN7ksz6IuDCaJ-1mcsvwYdRzjLTmCfL3~Qei8nVhaVMYA32FiTXHhtWKa2DF-4Dt89GJdrMAVgiJsMc4vJPwAfVCV-Setrc0tXfPeW9zomoAIurp-Fu8eUDMILnlvd8U~2n6FmjnE-HuXiH3ssLVwpLkQD6ecypX4ZHdNHdbWBEuQpCsjhsKjUE8ie7j2agis6zLlR71qZxI14bslrSz02PexgI0kj9iCuAllD1iPYHb~JSJCKmfQiCah3cd3MS6rx51R8qxeJQ__&Key-Pair-Id=APKAIE5G5CRDK6RD3PGA)
Cytomegalovirus (CMV) seropositivity in patients with coronavirus disease 2019 (COVID-19) is associated with effector memory reexpressing CD45RA (EMRA) T-cell activation. A, Quantification of the effect of CMV seropositivity on the abundance of circulating immune cells obtained from donors with COVID-19 (n = 127), estimated in a linear mixed model with a log-transformed immunophenotype as the response, controlled for age, sex, and self-reported race, then transformed to the original scale (with 99% confidence intervals [CIs] adjusted for false coverage). Abbreviations: cTfh, conventional T follicular helper cell; EM1, effector memory 1; PD-1, programmed cell death 1 protein. B, Unmodified Uniform Manifold Approximation and Projection (UMAP) projection of patients recovered from COVID-19 (n = 27), healthy controls (n = 24), and patients with COVID-19 (n = 127), as described elsewhere [15], using single-positive populations of all immune cell subsets. Individuals are color coded by their CMV serostatus; black square indicates cluster of CMV-seropositive patients (n = 20) further examined in D. Abbreviation: NA, non available. C, UMAP model illustrated as described elsewhere [15], with feature-weighted density contours overlaid on patients with COVID-19 (n = 127). The illustrated gradient vector was computed according to the direction of maximum Pearson correlation for the flow cytometric feature of interest (here, EMRA CD8+ T cells) and weighted in length according to the strength of the resulting Pearson coefficient. Red lines indicate high EMRA; blue lines, low EMRA T-cell proportions. The intensity of the dots represents the z score, and the dotted line is drawn perpendicular to the gradient vector. Triangles represent deaths within 90 days of admission, as reported elsewhere [15]. D, Immunophenotypes (n = 279) significantly associated with the cluster of CMV-seropositive patients (sample size, n = 20) displayed in B, as estimated in a linear mixed model with a log-transformed immunophenotype as the response, controlled for age, sex, and self-reported race and then transformed to the original scale (with 99% CIs adjusted for false coverage).
Our previous study on immune landscape of patients with COVID-19 not only profiled approximately 200 individual immunophenotypes but also identified 3 “immunotypes” associated with poor clinical trajectories versus improving health [15]. Immunotype 1 was characterized by CD4+ T-cell activation and proliferation and T-bet+ plasmablasts; immunotype 2 by CD8+ T-cell effector memory and EMRA subsets, some CD8+ T-cell activation and CD138+ and Ki67+ plasmablasts responses, and immunotype 3 by low activation of T- and B-cell responses [15]. These immunotypes were identified using UMAP of flow cytometry data together with clinical features that represented the immune landscape of healthy donors, recovered donors and patients with COVID-19 disease in 2-dimensional space [15].
To further interrogate the global impact of CMV, we projected CMV serostatus onto the UMAP previously generated from these healthy controls (including both CMV seronegative and CMV seropositive) and patients with COVID-19. CMV serostatus was not significantly associated with immunotypes 1, 2, or 3 (P > .05; data not shown). However, we identified a UMAP region where seropositive patients aggregated (Figure 4B). When projecting EMRA CD8+ T cells, the CMV-seropositive UMAP cluster falls at the edge of the group with high proportions of EMRA, including patients with a strong immunotype 1 signature, as indicated by high levels of component 1 (Figure 4C). Using a linear mixed model, this cluster enriched in CMV seropositivity was defined by 279 high-resolution immunophenotypes, either up-regulated or down-regulated in response to SARS-CoV-2 infection (Figure 4D and Supplementary Table 2). The major immunophenotypes increased in this cluster of patients included activated EMRA CD4+ and CD8+ T cells expressing HLA-DR and CD38, TBET, or CX3CR1, as well as CD138+ and Ki67+ plasmablasts.
In previous studies [15], these features were associated with increased disease severity. Immunophenotypes decreased in this CMV-seropositive cluster included total or nonactivated EMRA CD4+, CD8+ T cells expressing Eomesodermin (EOMES), central memory CD4+ T cells, and programmed cell death 1 protein–expressing CD8+ T cells. To evaluate the CMV specificity of activated cells, we assessed CMV peptide–dependent AIM expression [23]. After 24 hours of CMV peptide pool stimulation, antigen-specific interferon (IFN) γ+41BB+CD8+ T cells were detected in all antigen-experienced CD8+ T-cell compartments, T cells) (Figure 5A–5C and Supplementary Figure 2).
![A portion of effector memory reexpressing CD45RA (EMRA) CD8+ T cells activated during coronavirus disease 2019 (COVID-19) are cytomegalovirus (CMV) specific. A, Identification of interferon (IFN) γ+41BB+CD8+ T cells within CD45RA+CD27+CCR7+ (naive), CD45RA−CD27+CCR7+ (central memory), CD45RA−CD27+CCR7− (effector memory 1), CD45RA−CD27−CCR7− (effector memory 2), CD45RA−CD27−CCR7+ (effector memory 3), CD45RA+CD27− (EMRA) [15] in an unstimulated (top row) and a CMV peptide–stimulated (bottom row) donor. B, Overlay of IFN-γ+41BB+CD8+ T cells on a CD45RA/CD27 scatterplot from the donor as in A. C, Summary of frequencies of IFN-γ+41BB+CD8+ T cells within T-cell subsets as in A, in 4 unstimulated (blue dots) and CMV-peptide stimulated (red dots) donors.](https://oup.silverchair-cdn.com/oup/backfile/Content_public/Journal/jid/226/3/10.1093_infdis_jiac020/1/m_jiac020_fig5.jpeg?Expires=1749900302&Signature=P98M9aksRD-Upq47yb00WXqg6-zIa7qDmi~3jm0Kz7FGwRa0e-2qHtygRxbPgdkGFwid9cnxKlWw8xr0iGEm6eIwTUuQvaVrWQsJG-NVtaUpBrX5lR4Sx6BZinKgoEQqOHpe9SYyqQ-knx9-lgH7oJyZzDp~D6KJEW-HouRIB4SAqiF6MP1z71DEzrqMLagPKATYBfYqrdCUyD7FCCYuiSKt72JP5V9e3LPxQlIhZDqB3L9QiOPoDk4JyBYyM6x6M-gQQXHw3nNo3PKSt~9BkgFi1qGx3bgRAYtHUDUwY~IS1hM-v9avfUXi8PmU8swdoxAq38SHpDI0cDLdsWu4dA__&Key-Pair-Id=APKAIE5G5CRDK6RD3PGA)
A portion of effector memory reexpressing CD45RA (EMRA) CD8+ T cells activated during coronavirus disease 2019 (COVID-19) are cytomegalovirus (CMV) specific. A, Identification of interferon (IFN) γ+41BB+CD8+ T cells within CD45RA+CD27+CCR7+ (naive), CD45RA−CD27+CCR7+ (central memory), CD45RA−CD27+CCR7− (effector memory 1), CD45RA−CD27−CCR7− (effector memory 2), CD45RA−CD27−CCR7+ (effector memory 3), CD45RA+CD27− (EMRA) [15] in an unstimulated (top row) and a CMV peptide–stimulated (bottom row) donor. B, Overlay of IFN-γ+41BB+CD8+ T cells on a CD45RA/CD27 scatterplot from the donor as in A. C, Summary of frequencies of IFN-γ+41BB+CD8+ T cells within T-cell subsets as in A, in 4 unstimulated (blue dots) and CMV-peptide stimulated (red dots) donors.
These frequencies are in same range as previously reported for healthy donors [27–29]. As such, our results indicate that a portion of EMRA CD8+ T cells activated during COVID-19 may be CMV specific, but the distribution of CMV-specific CD8+ T cells among human memory T-cell subsets is unlikely to be dramatically skewed in patients with COVID-19. Rather, these data suggest that general features of immune perturbation in patients with COVID-19 preferentially affect EMRA cells. Whether this EMRA activation is causal to some aspects of more severe COVID-19 disease or simply a biomarker remains unclear, but these results indicate that CMV seropositivity in patients with COVID-19 is associated with increased activation of EMRA T cells.
DISCUSSION
Increasing age, obesity, hypertension, diabetes, underlying chronic kidney disease, and chronic pulmonary disease have been associated with increased severity of COVID-19 [4, 5, 7]. Although these factors may explain much of the variation driving cases of severe COVID-19, 20%–50% of severe cases occur in the absence of obvious preexisting conditions or demographic features associated with increased risk [7]. Previous work found an increased seroprevalence of CMV and herpes simplex virus 1 in hospitalized compared with nonhospitalized patients with COVID-19 [30], although contributions of other cofactors, such as age, self-reported race, and sex, were not clarified.
Our work extends these previous observations, controlling for age, self-reported race, and sex and applying a multivariate adjustment approach on 2 large cohorts to show that prepandemic CMV seropositivity was associated with both increased likelihood of testing positive for SARS-CoV-2 and increased severity of COVID-19 disease. While greater likelihood of SARS-CoV-2 positivity in CMV-positive individuals could reflect either social/behavioral factors influencing risk of both infections or biological effects of CMV, disease severity data suggest that prior CMV infection likely modifies the response to SARS-CoV-2 infection and contributes to severity. These observations support the possible use of CMV seropositivity as part of a risk stratification approach for patients with COVID-19. At the population level, these data may also help account for demographic and/or geographic differences in SARS-CoV-2 infection or disease burden based on population differences in CMV infection rates [11].
Comparison with immune profiling data revealed an immunological signature of severe COVID-19 associated with CMV seropositivity, including activation of EMRA T cells. EMRA T cells are increased during CMV infection in healthy individuals, are enriched in CMV-specific T-cell populations, and are thought to contribute to ongoing containment of this latent infection [9]. Activation of EMRA T cells in CMV-seropositive patients with COVID-19 could result from local reactivation of CMV in the upper respiratory tract. However, our data suggest that local respiratory CMV reactivation does not explain the association of CMV serostatus with more severe COVID-19. Previous work found that CMV viremia was similar between patients with COVID-19 and controls [31]. CMV reactivation is challenging to detect, and it is possible that reactivation outside the respiratory tract occurs in patients with COVID-19. An alternative is that the expanded EMRA T-cell populations present in CMV-seropositive individuals are activated during severe COVID-19 in a bystander manner.
Association of CMV serostatus with more severe COVID-19 suggests a role for CMV-induced immune system remodeling in the pathogenesis of SARS-CoV-2 infection. CMV has a broad effect on the immune system, shaping the blood T-cell compartment toward more effectorlike and EMRA cells, and leads to expansion of NKG2C+ NK cells [9, 12]. Although it is estimated that CMV is the cause of >30% of clonal CD8+ T-cell expansions in peripheral blood with aging, a significant portion of expanded T-cell clones are likely not CMV specific but rather proliferating in response to inflammation [29]. In agreement with this idea, approximately 2% of activated EMRA CD8+ T cells responded to CMV peptide stimulation, consistent with the possibility that at least some of the activated EMRAs in patients with COVID-19 may be CMV specific but suggesting that COVID-19 does not result in dramatic expansion of CMV-specific CD8+ T cells in the EMRA compartment, at least as detected by AIM assay. Other activated EMRA cells may arise from bystander clonal expansions and may have functional consequences by contributing to elevated circulating IFN-γ compared with seronegative individuals [13].
This effect of CMV in humans can enhance antibody responses to influenza vaccination [13]. In a mouse model of herpesvirus infection, elevated IFN-γ can provide protective immunity to unrelated infections through activation of macrophages [32]. Conversely, excessive macrophage activation may contribute to severe COVID-19 [33], raising the possibility that CMV-seropositive individuals have a higher baseline level of innate immune activation, leading to greater inflammatory response and increased disease severity during SARS-CoV-2 infection. CMV also induces CX3CR1+CD+ T cells, a subpopulation of EMRA or effector memory T cells with vascular homing and surveillance properties [34]. These CX3CR1+CD8+ T cells have been associated with atherosclerosis, transplant vascular sclerosis, and coronary restenosis [35], possibly as a result of CMV infection of endothelial cells [36]. Indeed, it was previously demonstrated that activation of this CX3CR1+ subset of CD8+ T cells in pediatric patients with multisystem inflammatory syndrome in children and COVID-19 and adult patients with COVID-19 associated with vascular complications [37]. Thus, a higher baseline number of CX3CR1+ vascular patrolling CD8+ T cells, combined with potential bystander activation of these cells during SARS-CoV-2 infection could potentially combine to enhance disease severity.
Finally, lungs are a major site of CMV latency and EMRA T cells can preferentially home to human lungs [38, 39]. It is therefore possible that activated EMRA T cells are preferentially recruited to the lung during SARS-CoV-2 and participate in organ injury driven by either low level CMV antigen expression or bystander activation from inflammatory cytokines. Thus, although future studies will be necessary to distinguish these possibilities, our data support the notion of CMV-mediated immune remodeling in the pathogenesis of severe SARS-CoV-2 infection.
Supplementary Data
Supplementary materials are available at The Journal of Infectious Diseases online. Supplementary materials consist of data provided by the author that are published to benefit the reader. The posted materials are not copyedited. The contents of all supplementary data are the sole responsibility of the authors. Questions or messages regarding errors should be addressed to the author.
Notes
Acknowledgments. We acknowledge the Penn Medicine BioBank (PMBB) and thank the Penn Medicine patients who consented to participate in this research program. We also thank the PMBB team and Regeneron Genetics Center for providing genetic variant data for analysis, as well as all patients and blood donors, their families and surrogates, and the medical personnel in charge of patient care. We thank the Wherry laboratory for discussions and for critically reading the manuscript, the Human Immunology Core (P30-CA016520) at the University of Pennsylvania for help with sample inactivation and enzyme-linked immunosorbent assays, and A. Fitzgerald, L. Khatib, and J. Graham for respiratory specimen collection.
UPenn COVID Processing Unit. This unit was made up of individuals from diverse laboratories at the University of Pennsylvania, all affiliated with the University of Pennsylvania Perelman School of Medicine, who volunteered time and effort to enable the study of patients with coronavirus disease 2019 during the pandemic; they included Amy E. Baxter, Kurt D’Andrea, Sharon Adamski, Zahidul Alam, Mary M. Addison, Katelyn T. Byrne, Aditi Chandra, Hélène C. Descamps, Nicholas Han, Yaroslav Kaminskiy, Shane C. Kammerman, Justin Kim, Allison R. Greenplate, Jacob T. Hamilton, Nune Markosyan, Julia Han Noll, Dalia K. Omran, Ajinkya Pattekar, Eric Perkey, Elizabeth M. Prager, Dana Pueschl, Austin Rennels, Jennifer B. Shah, Jake S. Shilan, Nils Wilhausen, and Ashley N. Vanderbeck.
Author contributions. Conceptualization: C. A. and E. J. W. Methodology: C. A., A. V., F. D. B., N. J. M., S. E. H., D. R., and E. J. W. Investigation: C. A., A. V., D. M., S. G., G. L., T. D., D. A. O., J. W., L. K. C., M. B. P., and the UPenn COVID Processing Unit. Project administration: N. J. M., S. E. H., and E. J. W. Supervision: M. R. B., R. G. C., F. D. B., N. J. M., and S. E. H. Writing the original draft: C. A., A. V., and E. J. W. Review and editing: all authors.
Financial support. This work was supported by the University of Pennsylvania Institute for Immunology Glick COVID-19 research award (grants AI155577, AI115712, AI117950, AI108545, AI082630, and CA210944 to M. R. B.); the Allen Institute for Immunology (to E. J. W. and grant R33HL137063 to R. G. C. and F. D. B.); the National Institutes of Health (grants HL137006 and HL137915 to N. J. M. [support for inpatient cohorts]); the Parker Institute for Cancer Immunotherapy (support to C. A., E. J. W., and the Cancer Immunology program at the University of Pennsylvania); the National Heart, Lung, and Blood Institute (grant R38 HL143613 to D. A. O.); the National Cancer Institute (grant T32 CA009140 to D. A. O.); the Smilow family (gift to the PMBB); the National Center for Advancing Translational Sciences, National Institutes of Health (Clinical and Translational Science Awards grant UL1TR001878 to the PMBB); and the Perelman School of Medicine at the University of Pennsylvania (support to the PMBB).
Potential conflicts of interest. N. J. M. receives funding to her institution from Athersys, Biomarck, Quantum Leap Healthcare Collaborative, and the Marcus Foundation, outside the current work. E. J. W. is a member of the Parker Institute for Cancer Immunotherapy, which supported the study; is an advisor for Merck, Marengo, Janssen, Related Sciences, Synthekine, and Surface Oncology; is a founder of Surface Oncology, Danger Bio, and Arsenal Biosciences; and has a patent on the programmed cell death 1 protein (PD-1) pathway. All other authors report no potential conflicts. All authors have submitted the ICMJE Form for Disclosure of Potential Conflicts of Interest. Conflicts that the editors consider relevant to the content of the manuscript have been disclosed.
Data and materials availability. All data associated with this study are present in the article or the Supplementary Materials. Code used to generate statistical analysis is available on GitHub: https://github.com/wherrylab/statistics_code.
References
Author notes
A. V. and D. M. contributed equally to this work.