-
PDF
- Split View
-
Views
-
Cite
Cite
Francis B Ntumngia, Richard Thomson-Luque, Sandra Galusic, Gabriel Frato, Sarah Frischmann, David S Peabody, Bryce Chackerian, Marcelo U Ferreira, Christopher L King, John H Adams, Identification and Immunological Characterization of the Ligand Domain of Plasmodium vivax Reticulocyte Binding Protein 1a, The Journal of Infectious Diseases, Volume 218, Issue 7, 1 October 2018, Pages 1110–1118, https://doi.org/10.1093/infdis/jiy273
- Share Icon Share
Abstract
Erythrocyte invasion by malaria parasites is essential for blood-stage development. Consequently, parasite proteins critically involved in erythrocyte invasion, such as the Plasmodium vivax reticulocyte binding proteins (RBPs) that mediate preferential invasion of reticulocytes, are considered potential vaccine targets. Thus, targeting the RBPs could prevent blood-stage infection and disease. The RBPs are large, and little is known about their functional domains and whether individuals naturally exposed to P. vivax acquire binding-inhibitory antibodies to these critical binding regions. This study aims to functionally and immunologically characterize Plasmodium vivax RBP1a.
Recombinant proteins of overlapping fragments of RBP1a were used to determine binding specificity to erythrocytes and immunogenicity in laboratory animals. The naturally acquired antibody response to these proteins was evaluated using serum samples from individuals in regions of endemicity.
The N-terminal extracellular region, RBP1157–650 (RBP1:F8), was determined to bind both reticulocytes and normocytes, with a preference for immature reticulocytes. Antibodies elicited against rRBP1:F8 blocked binding between RBP1:F8 and erythrocytes. Naturally acquired anti-RBP1 binding-inhibitory antibodies were detected in serum specimens from P. vivax–exposed individuals from Papua New Guinea and Brazil.
Recombinant RBP1:F8 binds human erythrocytes, elicits artificially induced functional blocking antibodies, and is a target of naturally acquired binding-inhibitory antibodies.
Plasmodium vivax accounts for 132 million–391 million cases of clinical malaria each year, with about 12.4% of the infections in Africa and >70% in Asia and the Americas [1, 2]. This is a major economic burden, especially in countries where access to affordable healthcare is lacking [3, 4]. Despite its wide prevalence, malaria caused by P. vivax has long been overshadowed by malaria due to Plasmodium falciparum, which is responsible for most malaria-attributed deaths. Increasing reports of clinical severity, with emerging virulent forms of the parasite [5], widespread drug resistance [6–8], and recurrent clinical episodes [9], challenge the description of vivax malaria as benign. Generally, there is a lack of sustained naturally acquired protective immunity to P. vivax because immune responses are weak, short-lived, and biased toward strain-specific immunity [10–12]. All of these factors emphasize the need for prophylactic and therapeutic strategies, including the development of a vaccine against vivax malaria.
Host-erythrocyte invasion by merozoites is essential for blood-stage development of malaria parasites. P. vivax preferentially infects reticulocytes [13, 14], a process mediated by 2 parasite protein families known as the P. vivax Duffy binding protein (DBP) and P. vivax reticulocyte binding protein (RBP) families [15, 16]. Preference for reticulocyte cell type is typically attributed to the RBPs.
It is believed that RBPs select the reticulocytes for invasion and trigger the release of DBP from the micronemes in time for the final step of junction formation, just before invasion [17]. Ten genes of the P. vivax RBP family have been identified in the P. vivax genome, and some are highly expressed during the schizont stage in the blood [18]. Homologs are found in P. falciparum, Plasmodium knowlesi, Plasmodium reichenowi [19–21], and Plasmodium yoelii [22] and are also implicated in the early phase of the invasion process and regulate invasion through different receptor pathways. In P. falciparum, these homologs are well-characterized vaccine candidates [23, 24]. RBP1a and RBP2c are believed to form a complex and bind specifically to reticulocytes. However, their cognate receptors are not identified [16]. Given their essential role in the invasion process, RBPs are considered important vaccine targets against asexual blood stages of the parasite. Despite the essential nature of the RBPs in the invasion process, very little is known about their functional domains, their role in the invasion process, or their receptors on reticulocytes.
In this study, we functionally and immunologically characterized one member of this gene family (RBP1a). We determined that its ligand domain lies within the N-terminal extracellular region and binds both reticulocytes and mature erythrocytes. Naturally acquired anti-RBP1 antibodies are prevalent in sera of individuals in regions with natural exposure to P. vivax infections. Vaccination-induced and naturally acquired anti-RBP1a antibodies can block the binding of rRBP1a to erythrocytes. The ability of these antibodies to inhibit merozoite invasion of reticulocytes in short-term in vitro cultures will strengthen the potential of RBP1a for consideration as a vaccine candidate against blood-stage vivax malaria.
METHODS
Recombinant Protein Production
P. vivax RBP1a is extremely large (>300 kDa), making it difficult to express as a recombinant protein. To facilitate recombinant protein expression and identification of its minimal binding domain, overlapping fragments spanning the extracellular region of the RBP1a gene from the Sal1 allele (PVX_ 098585) were designed (Figure 1A). The DNA sequences were codon optimized for Escherichia coli expression, commercially synthesized (Invitrogen, Carlsbad, CA), and cloned into the pET21(a)+ expression vector with a C-terminal 6xHis-tag to facilitate purification. Protein expression was performed in E. coli BL21 (DE3) pLysE cells (Invitrogen). Briefly, bacteria cells were cultured in Luria broth until reaching an OD600 of 0.6–0.8, and protein expression was induced with 1 mM IPTG (final concentration) for 3 hours at 30°C. Cells were harvested by centrifugation at 11000 x g for 20 min and lysed in 20 mM phosphate buffer, pH 7.4, containing 0.5 M NaCl and 20 mM imidazole. Expressed proteins were purified by affinity chromatography, using Ni+ Sepharose 6 fast flow (GE Life Sciences, Pittsburgh, PA) according to the manufacturer’s recommendations. Purity of the antigens was checked by analysis via Coomassie-stained sodium dodecyl sulfate polyacrylamide gel electrophoresis and then dialyzed against phosphate-buffered saline (PBS) before storage at −80°C.

Production of recombinant reticulocyte binding protein 1a (rRBP1a). A, Schematic of the extracellular region of Plasmodium vivax RBP1a, showing overlapping fragments expressed as recombinant proteins in Escherichia coli. Cysteine-rich regions are in white. Positions of residues for each fragment on the protein sequence are indicated. B, Coomassie-stained sodium dodecyl sulfate polyacrylamide gel electrophoresis of affinity-purified rRBP1a antigen fragments. SP, signal peptide; TM, transmembrane domain.
Animal Immunizations
BALB/c (Envigo, North America) housed in specific-pathogen-free conditions were handled in compliance with good animal practice, and experimental procedures were performed in accordance with Institutional Animal Care and Use Committee protocols approved by the Division of Research Integrity and Compliance, University of South Florida. Groups of 15 mice were each immunized with rRBP1a fragments emulsified in Titermax Gold adjuvant as reported elsewhere [25]. Each mouse received 25 µg/dose of antigen injected subcutaneously at the base of the tail at 3-week intervals for a total of 2 immunizations. Blood specimens were collected from mice 3 weeks after the second immunization, and serum was stored at −20°C. Mice immunized with Titermax alone served as controls. Polyclonal rabbit anti-RBP1:F8 antibody was obtained through a commercial vendor (Envigo).
Measurement of Antibody Titers to rRBP1a Antigens
Standard enzyme-linked immunosorbent assay (ELISA) protocols were used to determine anti-RBP1a antibody titers against the homologous antigens. Each serum specimen was analyzed by an end-point titration ELISA as previously reported [25]. Briefly, 96-well microtiter plates were coated with 200 ng/well of rRBP1a overnight at 4°C, blocked with 5% skimmed milk powder in PBS/0.05% Tween 20, and then incubated with 3-fold dilutions of mice or rabbit sera in duplicate wells. Bound antibody was detected with AP-conjugated goat-anti-mouse or goat anti-rabbit antibody (KPL, Milford, MA). Sera from animals immunized with adjuvant alone or preimmune serum were used for background control.
Naturally Acquired Serological Responses to rRBP1a
Serum samples used for this study were from a previously surveyed population in Liksul, Papua New Guinea (PNG) [26], and Remansinho, Brazil [27]. Both studies were approved by the institutional review boards at Case Western Reserve University, the PNG Institute of Medical Research Advisory Committee, and the National Human Research Ethics Committee of the Ministry of Health of Brazil (approval no. 551/2010).
Serum samples from PNG (n = 120) and Brazil (n = 68) were screened for total immunoglobulin G (IgG) reactivity against the different rRBP1a antigens by a multiplex ELISA array, using a custom-made magnetic bead–based Magpix assay (MBA; Luminex), as previously described [27]. A pool of serum from clinically immune adults from PNG and 5 individual naive North American sera were included as positive and negative controls, respectively. Briefly, 1-µL aliquots of microspheres (Luminex) bound to antigen containing 3000 beads per antigen were dispensed to individual wells of round-bottomed microtiter plates. This assay was multiplex, with beads corresponding to different antigens. Duplicates of 100 μL of plasma diluted 1:100 in PBS containing 0.05% Tween 20 and 1% bovine serum albumin (BSA; PBT-20) were added to the wells and mixed. The plates were incubated with the beads, protected from light, on a microplate shaker for 45 minutes at room temperature. After removal of plasma and 2 washing steps with 100 μL of PBT-20, 100 μL of phycoerythrin-labeled goat anti-human IgG (γ chain specific; F[abʹ]2; Jackson Immunoresearch) diluted 1:500 in PBT-20 was added and incubated, protected from light, on a shaker for 15 minutes at room temperature. After 3 washes with 100 μL/well of PBT-20, the beads were resuspended in 100 μL of PBT-20 and analyzed on a Bio-Plex Multiplex Reader System with Bioplex Manager 6.1 software (BioRad, Hercules, CA). Samples with a mean fluorescence intensity (MFI) greater than the average MFI + 2 SD of the negative control sera for each antigen (the cutoff) were classified as positive. Individual serum positivity was expressed as a reactive index, calculated as the sample MFI divided by the cutoff [27].
Flow Cytometry to Determine Erythrocyte Binding
The different rRBP1a antigen fragments were tested for binding to human erythrocytes as previously described [28, 29]. Reticulocytes were isolated from buffy packs (Interstate Blood Bank, Memphis, TN) by immunomagnetic sorting, using CD71 beads (Miltenyi Biotec) as previously described [28]. Briefly, 5 µg of recombinant antigen in 100 µL of PBS/0.5% BSA was incubated with either 1 µL of purified reticulocytes (purity, >80%), reticulocyte-depleted red blood cells (normocytes), or total red blood cells for 3 hours at room temperature, with shaking. Unbound antigen was washed with the same buffer, and the cells were again incubated with mouse anti-His Alexa Fluor 488–conjugated antibody (Qiagen, Germantown, MD) at 4°C in the dark for 1 hour. After another wash step, the cells were resuspended in PBS/0.5% BSA, and erythrocyte binding was quantified by acquiring 100000 events per sample on a BD Accuri C6 flow cytometer. The data were analyzed using FlowJo 10.0 (Treestar, Ashland, OR). Percentage binding was determined by analyzing the number of erythrocytes with bound antigen relative to erythrocytes with no antigen. Binding of recombinant thioredoxin was used to determine background levels of protein binding (cutoff), while stained cells without bound antigen were used to define reticulocyte, normocyte, and total red blood cell populations.
Enzyme Treatment of Erythrocytes
Total red blood cells were treated with trypsin, chymotrypsin, and neuraminidase as previously described [30]. Briefly, 100 µL of packed cells were incubated with either 20 mU of neuraminidase (Fisher Scientific, Pittsburgh, PA), 1 mg/mL trypsin, 1 mg/mL chymotrypsin (Sigma, St. Louis, MO), or incomplete Roswell Park Memorial Institute 1640 medium (iRPMI; control) on a rotating wheel for 1 hour at 37°C. After 1 wash with iRPMI, the trypsin- and chymotrypsin-treated cells were incubated in 0.5 mg/mL of soybean trypsin inhibitor for 10 minutes at room temperature to inactivate the enzyme. The cells were again washed 3 times in iRPMI and stored at 4°C. Recombinant RBP1:F8 was tested for binding to the enzyme-treated erythrocytes as described above.
COS7 Cell Binding Assay
COS7 cells were transfected with the plasmid pEGFP-RBP1:F8 as previously reported [31]. The construct was designed to target the expressed RBP1:F8 domain on the surface of transiently transfected COS7 cells as a recombinant fused to enhanced green fluorescent protein, used as a transfection marker. For erythrocyte binding, the transfected cells were incubated with different erythrocyte types 42 hours after transfection. Unbound cells were washed off after 2 hours, and binding of erythrocytes to the COS7 cell surface–expressed RBP1:F8 was measured by counting rosettes (≥50% of the transfected COS7 cell surface was covered by adherent erythrocytes) in 30 microscope fields at 200 times the original magnification as previously described [32]; transfection was assessed concomitantly by detecting GFP expression during fluorescence microscopy. For inhibition of binding, transfected cells were preincubated with dilutions of test antibodies prior to incubation with human erythrocytes. Percentage binding-inhibition was determined by assessing the number of rosettes in wells of transfected cells exposed to test antibodies relative to the number of rosettes in control wells exposed to preimmune serum or purified naive IgG, as follows: 100 – [a/b × 100], where a is the number of rosettes in wells with the test antibody and b is the number of rosettes in wells with the control antibody.
Statistical Analysis
Data were analyzed using GraphPad Prism software, version 6.0 (GraphPad Software). Statistical analysis was performed using Kruskal Wallis 1-way analysis of variance, followed by the Dunn adjusted pair-wise multiple comparison analysis, to evaluate differences between mean values of the different RBP1 fragments, using RBP1:F8 as control. Differences were considered statistically significant at P values of <.05.
RESULTS
Recombinant RBP1a Proteins
The P. vivax RBP1a has a molecular weight of >300 kDa. To facilitate protein expression, 8 overlapping fragments were expressed as C-terminal His-tagged proteins from the extracellular domain of RBP1a (Figure 1A). With the exception of rRBP1:F7, all recombinant proteins were affinity purified as soluble antigens by column chromatography. Recombinant RBP1:F7 was purified as an insoluble protein from inclusion bodies and refolded by rapid dilution as previously reported [25]. Purity of the proteins was determined by sodium dodecyl sulfate polyacrylamide gel electrophoresis (Figure 1B). Recombinant RBP1:F4 contained multiple bands and was not considered for further analysis (data not shown).
Erythrocyte Binding Properties of the rRBP1a Antigens
The functional activities of the rRBP1a fragments were evaluated both by standard flow cytometry and COS7 cell in vitro binding assays. With the exception of rRBP1:F5 and rRBP1:F7, all of the different RBP1a fragments showed significant binding to both Duffy-positive and Duffy-negative reticulocytes (Figure 2A and Supplementary Figure 1A and 1B). However, binding of rRBP1:F8 to reticulocytes was 2–5-fold higher than all other fragments and only rRBP1:F8 bound to mature erythrocytes (normocytes) (Figure 2A and Supplementary Figure 1C). As expected, rDBPII (positive control antigen) bound to both reticulocytes and normocytes, while recombinant thioredoxin (negative control antigen) did not bind to any cell type. Because of the stronger binding properties of rRBP1:F8, this antigen was chosen for further analysis. Recombinant RBP1:F8 showed a higher preference for binding immature reticulocytes (Figure 2B).
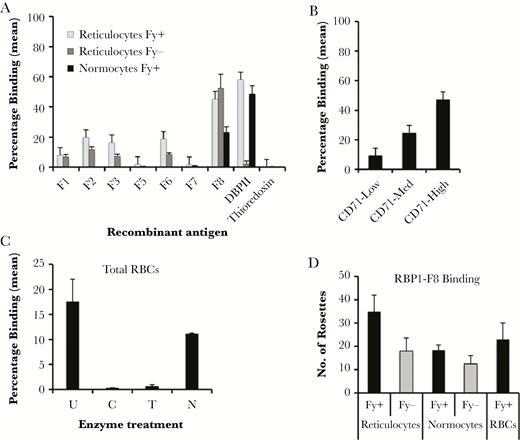
Erythrocyte-binding properties of recombinant reticulocyte binding protein 1a (rRBP1a). Recombinant RBP1 antigens binding to different red blood cell types (A), different reticulocyte subtypes (B), and enzyme-treated red blood cells (C), as revealed by flow cytometry. Recombinant Duffy binding protein II (DBPII) and thioredoxin were used as positive and negative control antigens, respectively. Bars show mean percentages of different red blood cells with bound antigen. D, COS7 cell surface–expressed RBP1:F8 binding to reticulocytes, reticulocyte-depleted erythrocytes (normocytes), and total red blood cells (RBCs). Bars represent the number of rosettes in 30 microscope fields at 20× original magnification. Error bars represent SDs from 2 independent experiments. C, chymotrypsin; Fy-, Duffy-negative cells; Fy+, Duffy-positive cells; N, neuraminidase; T, trypsin; U, untreated.
The binding specificity of rRBP1:F8 binding to enzyme-treated erythrocytes was also determined. rRBP1:F8 bound neuraminidase-treated but not trypsin- or chymotrypsin-treated erythrocytes (Figure 2C and Supplementary Figure 1D). Transiently expressed rRBP1:F8 on the surface of transfected COS7 cells also showed binding to both reticulocytes and normocytes (Figure 2D), thus confirming the results of flow cytometry.
Immunogenicity of rRBP1
To evaluate the immunogenicity of rRBP1a, groups of 15 BALB/c mice each were immunized with different overlapping rRBP1a antigens. Serum obtained from each mouse was tested by end-point titration ELISA against the homologous antigens. All mice generated high-titer anti-RBP1 antibodies against the homologous antigens (Figure 3A). Similarly, rRBP1:F8 produced high-titer antibodies in rabbits (Figure 3B). Immune serum raised against rDBPII did not cross-react with any of the rRBP1a antigens (Figure 3B and 3C).
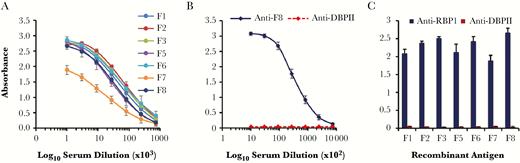
Immunogenicity of recombinant reticulocyte binding protein 1 (RBP1). Polyclonal antisera raised in mice (n = 15/antigen; A) against all rRBP1 antigens and in rabbits (n = 2; B) against rRBP1:F8 were tested by end-point dilution for reactivity with the homologous antigens. Each line represents a linear regression curve for reactivity of each mouse/rabbit serum against the respective antigens, and error bars indicate means ± SDs. (C) Antiserum against recombinant Duffy binding protein II (DBPII) was tested for cross-reactivity with recombinant RBP1 fragments. Preimmune serum served as background control.
Naturally Acquired Antibody Responses to rRBP1a
To determine whether individuals naturally exposed to P. vivax infections acquire antibodies to rRBP1a, immune responses to the different fragments were evaluated by multiplex ELISA in serum samples from individuals in malaria-endemic regions of PNG and Brazil. All antigens reacted with serum from both regions. However, there was considerable variation in the reactivity profiles of the individual serum specimens against the different fragments (Figure 4), and these responses could be assigned to 4 distinct antibody groups: high (reactive index, >300), medium (100–299), low (25–99), and none (<25; Supplementary Table 1). Of the PNG samples, 70%–85% had IgG antibodies to the different RBP1a antigens, compared with 56%–73% of the Brazilian samples. Similarly, 52% and 89% of the PNG and Brazilian samples, respectively, reacted with rDBPII. Compared with antibody responses against RBP1:F8, those against RBP1:F3 and DBPII were significantly lower for PNG samples (P = .03 and P < .001, respectively), and the response against RBP1:F2 was significantly lower for Brazilian samples (P = .004).
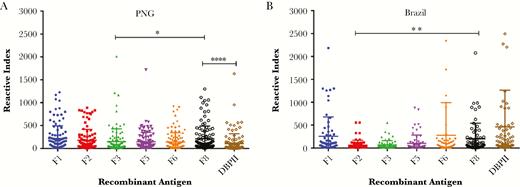
Naturally acquired immune response to recombinant reticulocyte binding protein 1 (rRBP1). Immune response to rRBP1 was determined in serum specimens from individuals from malaria-endemic regions of Papua New Guinea (PNG; n = 120; A) and Brazil (n = 68; B). Sera were screened for total immunoglobulin G against the different rRBP1a fragments and Duffy binding protein II (DBPII). Antibody titers were expressed as mean fluorescence intensity per sample, and positivity of individual serum specimens was expressed as a reactive index. Five North American naive serum samples were used as negative controls to determine the threshold of positive samples. The bars indicate the meanswithstandard deviations. *P = .03, **P = .004, and ****P < .0001.
Inhibition of RBP1a Binding to Erythrocytes
To determine anti-RBP1a activity, immune serum and purified total IgG antibodies generated against rRBP1:F8 in rabbits were tested for their ability to block binding of human erythrocytes to COS7 cell surface–expressed RBP1:F8. Both immune serum and the purified total IgG titer showed a concentration-dependent inhibition of RBP1:F8 binding to human erythrocytes (Figure 5A and 5B). Similarly, affinity purified human anti-RBP1:F8 IgG antibodies from serum of individuals naturally exposed to P. vivax infections also blocked binding of RBP1:F8 but not DBPII to erythrocytes (Figure 5C and Supplementary Figure 2).

Inhibition of erythrocyte binding to the N-terminal extracellular region of reticulocyte binding protein 1 (RBP1:F8). Mouse anti-RBP1:F8 serum (A), purified rabbit anti-RBP1:F8 total immunoglobulin G (IgG; B), and affinity-purified human anti-RBP1:F8 IgG (C) antibodies were tested for their ability to block COS7 cell surface–expressed RBP1:F8 from binding to human erythrocytes. Each bar or line represents mean percentage inhibition of binding for 2 independent experiments, with each dilution or concentration tested in duplicate. Error bars represent SDs. DBPII, Duffy binding protein II; RBCs, red blood cells.
DISCUSSION
Parasite ligands used by malaria parasites for host erythrocytes invasion are ideal targets for vaccine development to protect against blood-stage infection and disease. The P. vivax DBP is the best-characterized blood-stage vaccine candidate for P. vivax malaria and is known to interact with its cognate receptor, DARC, on human erythrocytes [33]. However, recent studies reveal evidence of P. vivax infections in DARC-negative individuals [34], suggesting the existence of alternative/redundant invasion pathways for P. vivax. Thus, there is a need to investigate the role of other ligands involved in the invasion process. It is believed that RBPs play a critical role in targeting reticulocytes for invasion [16] and trigger later invasion events leading to the final release of DBP from the micronemes, which forms the tight junction with DARC before invasion [35]. Thus, the vital role of the RBPs make them attractive vaccine targets. Furthermore, homologs of RBPs in P. falciparum including PfRh1 [36], PfRh4 [37], PfRh5 [38], are involved in erythrocyte binding and show promise as vaccine candidates. Therefore, it is logical to suggest that RBPs would also play a vital role in P. vivax invasion of reticulocytes.
Despite their vital role in the invasion process, the exact ligand domains of some RBPs are not yet defined. The binding of P. vivax RBP1a and RBP2a to reticulocytes is well established [16]. The ligand domain of RBP2a was recently identified, and its crystal structure was resolved [24]. Its general architecture is similar to that of PfRh5 [39, 40], an essential adhesin used by P. falciparum for erythrocyte invasion. In this study, we began the functional and immunological characterization of RBP1a. Using standard in vitro erythrocyte binding assays [29, 32], we identified the N-terminal region between residues 157 and 650 (RBP1:F8157–650) as the specific ligand domain of RBP1a (Figure 2A). Although other regions of RBP1a exhibited some binding to erythrocytes, RBP1:F8157-650 binding specificity was significantly higher as compared to the rest of the fragments. Further analysis indicated that this region preferentially binds immature reticulocytes, similar to P. vivax EBP2 [28]. Interestingly, RBP1:F8157–650 was the only domain that specifically bound to both reticulocytes and reticulocyte-depleted erythrocytes (normocytes), albeit with a lower binding specificity to the latter. These results are in accordance with a previous study, which reported a similar binding phenotype for RBP1a [41], suggesting that the binding phenotype of the RBPs is not restricted to reticulocytes. In related studies, RBP2b was reported as the only reticulocyte-specific binder in the RBP family [42], while RBP2a, with a crystal structure similar to that of PfRh5, binds to both cell types [24]. In another study, a 34-kDa protein domain of RBP1a (RBP1351–599) was reported as the putative binding region, with specificity for reticulocytes only [43]. Our data show that RBP1:F8F8157–650 has an erythrocyte binding specificity that is at least 2–3-fold higher than that of RBP1351–599 [43]. These differences in binding properties between rRBP1:F8F8157–650 (observed in this study) and rRBP1351–599 (reported by Han et al [43]) could be attributed to the relatively smaller size of the latter RBP, an indication that it lacked some of the key residues that make up the complete RBP1a ligand domain. This suggests that a complete structural scaffold, along with all key residues, is essential for optimal binding of RBP1a. This hypothesis is supported by data from this study, which demonstrate that the smaller RBP1:F7157–481 protein has very negligible erythrocyte binding affinity as compared to RBP1:F8157-650 (Figure 1A). Recently, Gupta et al mapped the binding domain of RBP1a to a region between residues 30 and 778. Interestingly, RBP1:F8157–650 falls within this region. However, they did not show any normocyte binding activity [44].
The receptors for most members of the RBP protein family are still unknown. A recent study revealed transferrin receptor 1 (also known as CD71) as the receptor for RBP2b. They demonstrated that this receptor is highly specific for RBP2b, an indication that P. vivax RBPs do not share a common receptor [45]. Similar to RBP2b, enzyme treatment studies indicate that the receptor for RBP1a is trypsin and chymotrypsin sensitive but neuraminidase resistant, an indication that this interaction is sialic acid independent. These data corroborate recent data from Gupta et al [44] and Han et al [43].
RBP1:F8157–650 contains a cluster of polymorphisms (17 of 42), most of which are nonsynonymous [46], a pattern similar to that of the DBP ligand domain [47], thus suggesting an important and potential functional region of the protein.
An important property of a good vaccine is its ability to induce a strong humoral immune response in immunized subjects. Similar to DBPII [25], RBP1:F8157–650 induced a strong immune response in laboratory animals. Since our previous studies have shown a lack of correlation between antibody titers and functional inhibition of DBPII binding to erythrocytes [11], we tested the ability of anti-RBP1:F8 antibodies to block the ligand-erythrocyte binding. We used an in vitro cytoadherence assay [31], which permits analysis of the specific interaction between the ligand domain (RBP1:F8157–650) expressed on the surface of transfected COS7 cells and human erythrocytes. Our data showed that anti-RBP1:F8 antibodies effectively blocked erythrocyte binding in a concentration dependent manner (Figure 5). The expressed protein on the COS7 cell mimics the native parasite protein on the merozoite, and this assay serves as a useful surrogate to evaluate the potential invasion-inhibitory effects of the anti-RBP antibodies in the absence of an effective culture system for P. vivax.
Naturally acquired antibodies to P. vivax merozoite proteins such as DBP are associated with protection against vivax malaria [27, 48]. Existing data from endemic regions suggest that members of the RBP family are naturally immunogenic [42, 49, 50]. However, very limited data exist on whether these antibodies can block RBP1a-erythrocyte binding activity. In this study, we showed the existence of anti-RBP1a antibodies in serum of individuals from two distinct malaria-endemic regions and that these antibodies have anti–RBP1a-erythrocyte binding activity. This is an indication that vaccination with rRBP1:F8 could potentially enhance naturally acquired immunity, which can be boosted through repeated exposure to infection.
In summary, we have identified the specific ligand domain of RBP1a and demonstrated that it binds both human reticulocytes and normocytes, is recognized by antibodies from individuals naturally exposed to P. vivax malaria, and can elicit blocking antibodies to prevent binding of RBP1:F8 to erythrocytes. The in vitro assay used in this study serves as a useful surrogate to evaluate the potential reticulocyte invasion-inhibitory effects of the anti-RBP1:F8 antibodies. However, the antiparasite activity of these antibodies is a more direct measure of vaccine efficacy. Future studies will evaluate the ability of these antibodies to block merozoite invasion of reticulocytes in short-term in vitro cultures to validate the vaccine potential of RBP1:F8 and support its inclusion as candidate for a multivalent blood-stage vivax malaria vaccine.
Supplementary Data
Supplementary materials are available at The Journal of Infectious Diseases online. Consisting of data provided by the authors to benefit the reader, the posted materials are not copyedited and are the sole responsibility of the authors, so questions or comments should be addressed to the corresponding author.
Notes
Acknowledgments. We thank the donors from PNG and Brazil for the human serum samples used for this study.
F. B. N. and J. H. A. designed the study. F. B. N. analyzed the data and drafted the manuscript. F. B. N., R. T. L., S. G., G. F., and S.F. performed experiments. C. L. K., D. S. P., M. U. F., and B. C. provided materials and technical advice. All authors reviewed and approved the manuscript.
Disclaimer. The content is solely the responsibility of the authors and does not necessarily represent the official views of the National Institutes of Health.
Financial support. This work was supported by the National Institutes of Allergy and Infectious Diseases, National Institutes of Health (grant R21AI107455 to F. B. N.).
Potential conflicts of interest. All authors: No reported conflicts of interest. All authors have submitted the ICMJE Form for Disclosure of Potential Conflicts of Interest. Conflicts that the editors consider relevant to the content of the manuscript have been disclosed.
References
Author notes
Presented in part: Vaccinology in the Tropics Conference, Panama City, Panama, 20–22 January 2016.