-
PDF
- Split View
-
Views
-
Cite
Cite
Jessica E Manning, David M Morens, Shaden Kamhawi, Jesus G Valenzuela, Matthew Memoli, Mosquito Saliva: The Hope for a Universal Arbovirus Vaccine?, The Journal of Infectious Diseases, Volume 218, Issue 1, 1 July 2018, Pages 7–15, https://doi.org/10.1093/infdis/jiy179
- Share Icon Share
Abstract
Arthropod-borne viruses (arboviruses) are taxonomically diverse causes of significant morbidity and mortality. In recent decades, important mosquito-borne viruses such as West Nile, chikungunya, dengue, and Zika have re-emerged and spread widely, in some cases pandemically, to cause serious public health emergencies. There are no licensed vaccines against most of these viruses, and vaccine development and use has been complicated by the number of different viruses to protect against, by subtype and strain variation, and by the inability to predict when and where outbreaks will occur. A new approach to preventing arboviral diseases is suggested by the observation that arthropod saliva facilitates transmission of pathogens, including leishmania parasites, Borrelia burgdorferi, and some arboviruses. Viruses carried within mosquito saliva may more easily initiate host infection by taking advantage of the host’s innate and adaptive immune responses to saliva. This provides a rationale for creating vaccines against mosquito salivary proteins, rather than against only the virus proteins contained within the saliva. As proof of principle, immunization with sand fly salivary antigens to prevent leishmania infection has shown promising results in animal models. A similar approach using salivary proteins of important vector mosquitoes, such as Aedes aegypti, might protect against multiple mosquito-borne viral infections.
Currently, hundreds of millions of human mosquito-borne infections occur each year [1–3]. There are as many as 19 million species of arthropods, many of which carry disease-causing pathogens that are transmitted via “bites” to humans and animals [4]. Arthropod salivas secreted by vectors such as ticks, sand flies, and mosquitoes are composed of heterogenous mixes of active compounds that facilitate transmission of pathogens into mammalian hosts including cows, dogs, and humans [5]. Such vector-borne pathogens make up 17% of all communicable diseases in humans, causing 1 billion cases and 1 million deaths per year [1]. A significant contributor to this morbidity and mortality is the taxonomically diverse group of mosquito-borne viruses of increasing clinical importance (Table 1).
Vector . | Virus . | Family . | Geographical Distribution . |
---|---|---|---|
Aedes spp | Dengue virus | Flavivirus | Africa, Asia, Americas |
Yellow fever virus | Flavivirus | Africa, South America | |
Zika virus† | Flavivirus | Africa, Asia, Americas | |
Chikungunya virus | Alphavirus | Africa, Asia, Americas | |
Semliki Forest virus | Alphavirus | Africa, Asia, Europe | |
La Crosse encephalitis virus | Bunyavirus | North America | |
Spondweni virus | Flavivirus | Africa | |
Culex spp | West Nile virus† | Flavivirus | North America |
St. Louis encephalitis virus | Flavivirus | North America | |
Japanese encephalitis virus† | Flavivirus | Southeast Asia | |
Sindbis virus | Alphavirus | Africa, Asia, Australia | |
Usutu virus | Flavivirus | Africa, Europe | |
Aedes and/or Culex spp | Venezuelan equine encephalitis virus | Alphavirus | Americas |
Ross River virus | Alphavirus | Australia | |
Eastern equine encephalitis virus‡ | Alphavirus | North America | |
Rift Valley fever virus | Bunyavirus | Africa and Middle East | |
Cache Valley virus | Bunyavirus | North America | |
Haemogogus spp | Mayaro virus§ | Alphavirus | Africa |
Anopheles spp | O’nyong-nyong virus | Alphavirus | Africa |
Vector . | Virus . | Family . | Geographical Distribution . |
---|---|---|---|
Aedes spp | Dengue virus | Flavivirus | Africa, Asia, Americas |
Yellow fever virus | Flavivirus | Africa, South America | |
Zika virus† | Flavivirus | Africa, Asia, Americas | |
Chikungunya virus | Alphavirus | Africa, Asia, Americas | |
Semliki Forest virus | Alphavirus | Africa, Asia, Europe | |
La Crosse encephalitis virus | Bunyavirus | North America | |
Spondweni virus | Flavivirus | Africa | |
Culex spp | West Nile virus† | Flavivirus | North America |
St. Louis encephalitis virus | Flavivirus | North America | |
Japanese encephalitis virus† | Flavivirus | Southeast Asia | |
Sindbis virus | Alphavirus | Africa, Asia, Australia | |
Usutu virus | Flavivirus | Africa, Europe | |
Aedes and/or Culex spp | Venezuelan equine encephalitis virus | Alphavirus | Americas |
Ross River virus | Alphavirus | Australia | |
Eastern equine encephalitis virus‡ | Alphavirus | North America | |
Rift Valley fever virus | Bunyavirus | Africa and Middle East | |
Cache Valley virus | Bunyavirus | North America | |
Haemogogus spp | Mayaro virus§ | Alphavirus | Africa |
Anopheles spp | O’nyong-nyong virus | Alphavirus | Africa |
*Names used in this table are from the 10th report of the International Committee of Taxonomy of Viruses: The Classification and Nomenclature of Viruses (2017).
†May have additional vectors.
‡Vector competence demonstrated in Aedes spp.
Vector . | Virus . | Family . | Geographical Distribution . |
---|---|---|---|
Aedes spp | Dengue virus | Flavivirus | Africa, Asia, Americas |
Yellow fever virus | Flavivirus | Africa, South America | |
Zika virus† | Flavivirus | Africa, Asia, Americas | |
Chikungunya virus | Alphavirus | Africa, Asia, Americas | |
Semliki Forest virus | Alphavirus | Africa, Asia, Europe | |
La Crosse encephalitis virus | Bunyavirus | North America | |
Spondweni virus | Flavivirus | Africa | |
Culex spp | West Nile virus† | Flavivirus | North America |
St. Louis encephalitis virus | Flavivirus | North America | |
Japanese encephalitis virus† | Flavivirus | Southeast Asia | |
Sindbis virus | Alphavirus | Africa, Asia, Australia | |
Usutu virus | Flavivirus | Africa, Europe | |
Aedes and/or Culex spp | Venezuelan equine encephalitis virus | Alphavirus | Americas |
Ross River virus | Alphavirus | Australia | |
Eastern equine encephalitis virus‡ | Alphavirus | North America | |
Rift Valley fever virus | Bunyavirus | Africa and Middle East | |
Cache Valley virus | Bunyavirus | North America | |
Haemogogus spp | Mayaro virus§ | Alphavirus | Africa |
Anopheles spp | O’nyong-nyong virus | Alphavirus | Africa |
Vector . | Virus . | Family . | Geographical Distribution . |
---|---|---|---|
Aedes spp | Dengue virus | Flavivirus | Africa, Asia, Americas |
Yellow fever virus | Flavivirus | Africa, South America | |
Zika virus† | Flavivirus | Africa, Asia, Americas | |
Chikungunya virus | Alphavirus | Africa, Asia, Americas | |
Semliki Forest virus | Alphavirus | Africa, Asia, Europe | |
La Crosse encephalitis virus | Bunyavirus | North America | |
Spondweni virus | Flavivirus | Africa | |
Culex spp | West Nile virus† | Flavivirus | North America |
St. Louis encephalitis virus | Flavivirus | North America | |
Japanese encephalitis virus† | Flavivirus | Southeast Asia | |
Sindbis virus | Alphavirus | Africa, Asia, Australia | |
Usutu virus | Flavivirus | Africa, Europe | |
Aedes and/or Culex spp | Venezuelan equine encephalitis virus | Alphavirus | Americas |
Ross River virus | Alphavirus | Australia | |
Eastern equine encephalitis virus‡ | Alphavirus | North America | |
Rift Valley fever virus | Bunyavirus | Africa and Middle East | |
Cache Valley virus | Bunyavirus | North America | |
Haemogogus spp | Mayaro virus§ | Alphavirus | Africa |
Anopheles spp | O’nyong-nyong virus | Alphavirus | Africa |
*Names used in this table are from the 10th report of the International Committee of Taxonomy of Viruses: The Classification and Nomenclature of Viruses (2017).
†May have additional vectors.
‡Vector competence demonstrated in Aedes spp.
Rapidly emerging viruses such as Zika virus (ZIKV) and chikungunya virus (CHIKV), carried by Aedes aegypti mosquitos, in the Americas have added to the ongoing burden of more established arboviruses such as the 4 dengue viruses (DENV) and West Nile virus (WNV) [1]. The major determinants of arboviral epidemics (ie, vector capacity, pathogen fitness, host susceptibility, and environmental conditions) make accurate predictions difficult [6]. This lack of predictability is of increasing public health concern, particularly given that new mechanistic models using environmental and socioeconomic factors suggest that other arboviruses, such as Ross River, Rift Valley, and potentially Mayaro viruses, have been considered likely to emerge and spread in the future [7].
Despite intense efforts, traditional public health approaches have not been able to control unexpected arboviral epidemics. Since it emerged in the United States in 1999, WNV has led to over 18000 cases of neuroinvasive disease with attack rates that vary unpredictably from season to season [8]. Since 2000, CHIKV has re-emerged on 6 continents as a source of debilitating acute and chronic joint pain that significantly contributes to the economic burden of arboviral diseases [3]. In terms of overall burden of disease, DENV has long been the most clinically significant arbovirus, infecting 390 million people each year with approximately 100 million symptomatic infections [2]. More recently, in 2015, the ZIKV epidemic and its attributable birth defects underline the need for a safe and effective Zika vaccine in Central and South Americas [9]. Moreover, in the past year, with a case fatality rate of 35%, yellow fever virus (YFV) re-emerged in the largest YFV outbreak in the Americas in a decade [10].
Vaccines are among the main defenses against arboviruses. Licensed and widely used arbovirus vaccines exist for tick-borne encephalitis virus, Japanese encephalitis virus, and YFV, although shortages occur with the YFV vaccine supply [11]. A DENV vaccine is now licensed in select countries, but its development has been more complicated given the presence of 4 circulating serotypes and controversies surrounding the phenomenon of possible vaccine-induced immune potentiation of more severe illness in children [2, 12]. Furthermore, given that ZIKV and DENV are phylogenetically related flaviviruses, it is not clear whether cross-reactive B- and T-cell responses would lead to more severe clinical manifestations of ZIKV infection in DENV-vaccinated or immune individuals. Current research efforts are underway to elucidate such complicated immunologic interplay.
Given these complex flavivirus immune interactions, along with great arbovirus diversity, vector-based vaccine approaches may offer a solution to control mosquito-borne disease by taking advantage of a common variable—mosquito saliva [6]. Because the human host’s natural response to vector saliva may promote the infectivity of pathogens carried in the saliva, the presence of vector saliva aids in the establishment of systemic infection [5, 13–15]. This phenomenon could potentially be harnessed in a novel vaccine approach via modification of the host response to arthropod saliva through vaccination with arthropod saliva, such as saliva from ticks, sand flies, or mosquitos [16–19], conceivably preventing widespread infection by creating an immune environment that blocks transmission or destroys the pathogen.
By targeting the vector-pathogen-host interface, mosquito saliva-based vaccines might bypass in vivo virus-specific immunological phenomena because they act very early at the site of the vector bite in the skin, pre-empting or complementing host antiviral immune responses [20]. This also provides the possible additional benefit of providing pan-viral protection for a specific vector such as A aegypti that transmits ZIKV, DENV, YFV, and CHIKV, which would be particularly useful for endemic regions with multiple cocirculating arboviruses [21]. Efforts are currently underway to develop and test vaccines using select mosquito salivary peptides with the goal of protection against multiple mosquito-borne pathogens, particularly emerging threats such as ZIKV as well as arboviruses that have yet to emerge [22]. The following is a summary of the immunology and rationale of mosquito salivary protein-based vaccines as well as future challenges for their development and use (Box 1).
Endurance of the immune response to a saliva protein-based vaccine when natural exposure in humans is not well characterized, although may be short in duration without ongoing vector exposure
Variable efficacy or immunogenicity dependent upon a population’s local vector abundance and biting rates
Unclear role of saliva-induced local inflammation in helping or hindering viral replication and dissemination
Clinical significance of allergic-type reactivity to saliva proteins or other potential adverse events
Possibility of an enhanced infection postvaccination given animal model, selection of wrong protein, or vector-pathogen pair specificity
Reduced ability to draw conclusions from immunocompromised mouse models of arboviruses to the human immune response
Lack of impact on non-arthropod transmission mechanisms (eg, sexual transmission, vertical transmission, blood transfusion)
MOSQUITO SALIVA MODULATES THE HOST IMMUNE RESPONSE
What Happens During a Mosquito Bite?
Mosquitoes do not “bite” when they take a blood meal. Rather, the mosquito inserts its proboscis, a mouth-like structure, into the skin. As it probes for blood, the mosquito ejects a salivary mix of vasodilators, anticoagulants, and other anti-hemostatic components into both the epidermis and the dermis [6]. In addition to factors that keep blood flowing during engorgement, mosquito saliva also contains proteins that modulate both innate and adaptive immune responses. Feeding by Aedes and Culex mosquitoes in C3H/HeJ mice down-regulated Th1 cytokines such as interferon (IFN)-γ and up-regulated Th2 cytokines such as interleukin (IL)-4 and IL-10, cytokine shifts that were not seen when mosquitoes fed on flavivirus-resistant host mice [23]. Increased Th2 response is the cause of irritation and allergic reaction seen with mosquito bites [24]. Although there is decreased T-cell recruitment to the site of injury after mosquito feeding, there is increased recruitment of neutrophils, eosinophils, and dendritic cells [25]. Both inoculation of salivary gland extracts and feeding by Aedes or Culex mosquitos inhibit mast cell degranulation. However, with repeated exposures to the vector’s bites, an individual is desensitized to some components of the saliva and is able to mount a more effective proinflammatory Th1 response, providing a scientific basis for vaccination with salivary peptides [24].
What Happens When the Mosquito Is Carrying an Arbovirus?
When a mosquito takes blood from a viremic host, the arbovirus is transported to the mosquito midgut. To establish infection, an arbovirus must first penetrate the midgut barrier and within several days infect the salivary glands and other distal tissues [6]. After an extrinsic incubation period that varies between arboviruses, the infected mosquito can now inoculate virus-laden saliva into the dermis of the next human host upon which it feeds (Figure 1A) [1, 6]. Most such arboviruses inoculated into dermal pericapillary tissues actively replicate in Langerhans cells, which migrate from the initial dermal site to regional lymph nodes [25]. From there, the virus replicates to achieve varying levels of viremia, which may correlate with the onset of classic clinical symptoms such as fevers and rash [25]. As the viremia clears, complications of arboviral infection such as dengue shock syndrome, chronic arthritis in CHIKV, and Guillaime-Barré Syndrome in Zika, an active area of research, may well depend upon an individual’s immune status (eg, flavivirus-naive) [3].
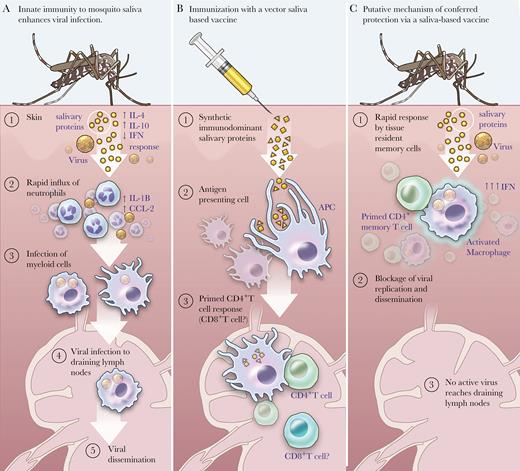
Mosquito saliva-based vaccine: proposed mechanism of action. (A) A virus-infected mosquito seeks a blood meal while injecting salivary proteins and viral particles from its salivary glands. The bite trauma coupled with the salivary effects cause vascular leakage, edema, mast cell degranulation, and release of host Th2-predominant cytokines. As the virus infects stromal cells in the dermis, neutrophils are recruited to the dermis, which in turn release chemokines that attract myeloid cells that become infected, and which traffic virus to the lymph nodes, leading to systemic infection. (Note: This scenario is based on evidence primarily derived from dengue virus-infected mice using natural transmission models, as cited within text.) (B) Upon inoculation with immunodominant synthetic salivary peptides, antigen-presenting cells such as dendritic cells can take them up and interact with CD4+ T cells to prime an immune response upon re-exposure to mosquito saliva. It is not clear what role CD8+ T cells play in this milieu. (C) Upon challenge with a virus-infected mosquito, the host may mount a rapid response to the saliva via skin-resident memory CD4 T cells. The saliva is then unable to immunomodulate the host immune response towards a Th2 response, but the host can mount a Th1-type antiviral response with interferon release by CD4+ T cells and macrophage activation. Given that the virus is a “bystander” because the response is primed to salivary antigens, systemic infection may not succeed because of one of the following scenarios: (1) the virus is unable to infect myeloid cells and replicate; (2) the virus is unable to infect cellular targets such as macrophages or monocyte-derived dendritic cells that will traffic virus to draining lymph nodes; or (3) the virus is phagocytosed by the activated macrophage. Further research is needed to determine the exact mechanism of action. Abbreviations: APC, antigen presenting cell; IFN, interferon; IL, interleukin.
As noted, mosquito saliva can facilitate the infectivity of an arbovirus by dampening the host’s antiviral Th1 immune response [26–28]. Codeposition of mosquito saliva with an offending pathogen allows the pathogen to enter the host and more easily replicate [29]. Mice inoculated intradermally with WNV in the presence of Culex tarsalis saliva (via mosquito feeding or coinoculation) developed higher viral loads and faster onset of neuroinvasive disease compared with mice who were inoculated intradermally with WNV alone [30]. This difference was not observed when salivary gland extract was administered at a site distal to viral inoculation, suggesting that the immunomodulatory effects of saliva operate locally.
In a study of mice inoculated with Aedes mosquito salivary gland extract, DENV pathogenesis during secondary infection was associated with increased viral titers in the skin, overall infection and migration of skin immune cells, and endothelial permeability [31]. The presence of Aedes spp salivary gland extract has also been shown to suppress antimicrobial peptide secretion by human keratinocytes, which in turn leads to enhanced DENV replication in these cells. The protein responsible for this immune-dampening effect is a 34-kDa salivary protein of unknown function, but it was found to inhibit expression of type 1 IFN, as well as its regulatory factors [32]. Another salivary protein, CLIPA3 protease, promotes DENV replication and dissemination via cleavage of extracellular matrix proteins, liquefying the dermal layer. This dermal liquefaction theoretically permits interaction between virions and local immune cells that subsequently migrate to draining lymph nodes [33]. The recent discovery of a 15-kDa salivary protein LTRIN illustrates how a salivary protein augments ZIKV pathogenesis in Ifnar−/− mice by disrupting the communication link between the lymph nodes and surrounding stromal environment [34].
Understanding of the immune response in human skin after an infected mosquito bite is evolving. A recent study of the mosquito-borne alphavirus Semliki Forest virus in immunocompetent mice highlighted the role of the cutaneous innate immune response in saliva-mediated pathogenesis [5]. In this study, exposure to virus-infected A aegypti mosquito saliva via feeding caused an influx of neutrophils that facilitated infection of macrophages and monocyte-derived dendritic cells, which subsequently trafficked virus to draining lymph nodes. By depleting neutrophils and inhibiting the inflammation, the host mouse could suppress early phases of viral infectivity and dissemination. Furthermore, bite-associated edema was correlated to retention of virus in the skin at higher concentrations and facilitated ongoing infection of keratinocytes [5]. This evidence suggests that the disease-promoting mechanisms of saliva are not exclusive to perturbation of the host antiviral response but also include alteration of innate responses in the dermis [5, 23, 26].
What Happens At the Pathogen-Skin-Saliva Interface After Vaccinations With Salivary Antigens?
In mouse models of malaria and leishmaniasis, repeated host exposure to vector saliva shifts the immune response from a Th2 to a Th1 response, correlated with reduced development of disease [18, 35]. This suggests that if a vertebrate host is immunized with synthetic immunodominant peptides derived from mosquito saliva (Figure 1B), subsequent exposure to the same mosquito saliva may allow immunomodulation of the host response that disadvantages the coinoculated arbovirus. Specifically, if an immunized vertebrate host has antisaliva peptide antibodies, the host immune response could maintain or augment its Th1 response and IFN release because the saliva’s immunomodulatory ability would be dampened (Figure 1C) [32, 33]. This could potentially lead to a decrease in immune cell recruitment to the site of the bite at the skin surface and a subsequent decrease in cellular traffic to the draining lymph nodes as seen in animal models [5, 32]. Whether other local effects such as disruption of endothelial integrity or dermal liquefaction occur—or might be worsened in the setting of an increased proinflammatory Th1 response—is not clear [31, 33].
The overall goal of vaccinating against vector saliva is to hinder creation of the physiologic microenvironment necessary for pathogen transmission, but further studies are necessary to determine the exact mechanisms. If successful, the pathogen may be stopped at the skin interface before reaching draining lymph nodes and causing systemic infection [Figure 1C].
LESSONS LEARNED FROM THE SALIVA OF OTHER ARTHROPODS: SAND FLIES AND TICKS
Sand Fly Salivary Protein Vaccine Development
Saliva of the phlebotomine sand fly Lutzomyia, the vector of various species of Leishmania, is more extensively characterized than mosquito saliva. Sialotranscriptomics has now assigned function to numerous sand fly salivary proteins leading to elucidation of salivary-mediated Leishmania pathogenesis [36]. The parasite is transmitted most effectively in naive compared with saliva-exposed animals, presumably owing to the development of protective antisaliva antibodies in the latter [35]. Preclinical models of infection in mice, hamsters, dogs, and rhesus macaques offer a proof-of-concept vaccine strategy using sand fly salivary components [14, 16, 19, 35]. As a result, saliva-based prophylaxis against leishmaniasis is better understood than other arthropod-borne diseases.
Exposure to uninfected sand fly saliva protected rodents from subsequent Leishmania major infections, and, extending from this observation, rodent immunization with salivary protein-encoding deoxyribonucleic acid (DNA) plasmids mitigated visceral leishmaniasis infections [19, 35]. Delayed-type hypersensitivity responses with elevated cutaneous IFN-γ expression contributed to parasite killing in the dermis and primed specific immunity to leishmaniasis [16, 37], suggesting that immunization to a salivary protein changes the immunological environment to a Th1-type response, thereby altering parasite antigen presentation and immune response. Similarly, beagles immunized with different sand fly salivary proteins showed elicitation of robust and long-lasting cellular immunity, underlining the protective role of a Th1 response in visceral leishmaniasis infections [14]. More recently, immunization of rhesus macaques with the sand fly salivary protein PdSP15 demonstrated a correlation between a Th1 cell-mediated immune response and protection from cutaneous leishmaniasis, as evidenced by reduction in lesion size and parasite load, although not necessarily by prevention of infection at the bite site in immunized animals [16].
As demonstrated in a rodent challenge model of leishmaniasis, it may be useful to combine pathogen and salivary components in a single vaccine [38]. A DNA vaccine containing the salivary protein PpSP15 complementary DNA was combined with a live recombinant nonpathogenic Leishmania tarentolae strain expressing select immunogenic cysteine proteases. BALB/c and C57BL/6 mice were then challenged with L major infection. The strongest protective effect was seen after mice were primed with salivary PpSP15 DNA then boosted with PpSP15 DNA and recombinant L tarentolae [38]. With at least 3 promising candidates, leishmania vaccine development is advancing in preclinical trials with consideration of natural transmission, the priming of animal models with sand fly bites before vaccination, and careful adjuvant selection to boost a Th1 response given existing reservoirs of asymptomatic infections in endemic areas [20].
Tick-Targeted Vaccine Development
Ticks also secrete saliva into the host during feeding and are known to carry a wide array of pathogens including Borrelia burgdorferi (the spirochete of Lyme disease), other bacteria responsible for the human diseases of anaplasmosis and ehrlichiosis, and viruses such as Crimean-Congo hemorrhagic fever and Kyasunar Forest disease [39]. Although vaccines do exist for tick-borne encephalitis viruses, there are no vaccines for other important tick-borne diseases. This dearth of therapeutic and preventive options is driving the progress of anti-tick vaccine development today.
Studies have demonstrated that after repeated vector exposure, various mammalian species develop “tick immunity” that is caused by antisalivary gland antibodies [40–44]. One possible tick saliva-targeted vaccine candidate is Salp15, a 15-kDa salivary protein with highly conserved amino acid sequences across Ixodes tick species that is responsible for inhibiting CD4 T-cell activation [15, 43]. When Balb/c mice are immunized with Salp15 protein, CD4+ T-cell activation is not affected and the immunized mice still produce high levels of IFN-γ. Despite CD4 T-cell activation’s role in humoral antibody production, there was no difference in murine antibody production in this study [15]. However, subsequent studies did show that C3H/HeJ mice immunized with Salp15 were protected from Borrelia infection delivered by both needle inoculation and by tick feeding [41].
Translation of promising preclinical study results to the natural host-tick-pathogen setting has been slow. The first evaluation of a vaccine targeting tick proteins involved in feeding on a natural host was published in 2016 [17]. This study evaluated a multivalent vaccine cocktail of Rhipicephalus appendiculatus tick antigens necessary for feeding on cattle and transmitting Theileria parva, the parasite responsible for East Coast Fever in cattle, a costly disease in Africa. Although the cattle demonstrated strong immune responses to all the peptide antigens in the vaccine, there was no demonstrable effect on tick feeding or reduction in transmission of T parva [17]. The observed lack of antitick effect may have been caused by antigenic competition or the presence of histamine-binding proteins also found in tick saliva that are responsible for reducing inflammation at the site of the bite. Therefore, although it is attractive to target arthropod saliva antigens as vaccine candidates, the complexity of tick saliva (with over 400 known proteins of likely redundant functions) may pose obstacles to tick-based vaccine development [17, 39].
Because arthropod salivary components are highly diverse, some proteins involved in pathogen transmission seem to counter each other when tested in isolation—with certain proteins facilitating, and others impeding, transmission [6, 13, 33, 45, 46]. In the case of ticks, saliva’s effects on sentinel tissue-resident cells is cell-type dependent, suggesting that although some salivary peptides may enhance pathogenesis, the composite effect may not ensure pathogen transmission [42]. There are also specificities of each pathogen-vector pair that respond differently to various insults. For example, in mosquitos, experimental animal data show that immunization with some isolated salivary proteins can augment virus transmission, such as the case with the salivary protein D7 immunization leading to increased WNV mortality in mice [45, 46]. However, when virus-inoculated mosquitos or whole salivary gland extracts are used in experiments, it appears that the overall effect of mosquito saliva facilitates pathogen transmission [6]. Given that the various actions of mosquito salivary proteins could complicate vaccine development (Box 1), selection of vaccine candidate peptides must be carefully approached. To facilitate this selection, functional sialotranscriptomics of mosquitos could further clarify the various roles of mosquito salivary proteins as it has done in leishmaniasis [20, 36]. Assembling the universal picture of vector-driven host immunologic responses and the interactive effects of salivary proteins will be essential to moving the field forward (Box 2).
What is the role of the vector’s microbiome in pathogen transmission efficiency?
For arboviruses carried by the same vector, how does coinfection impact the vertebrate host immune respond to a transmission-blocking vaccine?
How does arboviral replication in the dermis impact skin pathophysiology of arbovirus infections?
Although Aedes aegypti salivary proteins have been well characterized, what further knowledge may be gained from characterization of Aedes albopictus salivary repertoire?
How do multiple exposures to Aedes spp saliva in endemic populations impact the effectiveness of vector?
A ROAD AHEAD FOR MOSQUITO SALIVA-BASED VACCINES?
Preclinical Models of Infection
In preclinical studies, needle inoculation of a virus into an experimental host does not assess the critical role that the vector may play in disseminating an infection [13, 28]. This may be one reason that some candidate vaccines of vector-borne diseases succeed in the laboratory but fail as they progress in development to field-like conditions [47]. Therefore, animal models that mimic natural transmission via mosquito bite or coinoculation of mosquito saliva with the pathogen may be preferable, especially when evaluating immunogenicity of candidate vaccines during the late phases of preclinical testing. Using a natural mode of transmission is more logistically complex and has not been widely adopted even in recent mouse models of arboviral pathogenesis, but it is often used in malaria mouse models as well as controlled human malaria infections [48, 49]. Comparison of Plasmodium infection via needle inoculation versus mosquito bite are inconclusive with regard to saliva-mediated effects, which may be attributed to the sporozoites’ quick exit from the dermis and possible escape of dermal immune responses [18, 50, 51].
In the instance of ZIKV, animal models differ from humans with regard to immunogenetics, antibody repertoire, viral tropism, and pre-existing flavivirus cross-immunity [48, 52]. Perhaps as a result, ZIKV mouse models require very high viral challenges, typically done via needle inoculation, or IFN-knockout models such as Ifnar−/− mice to induce disease and transmission [48]. As a whole, the immunocompromised mouse models for salivary-mediated arboviral pathogenesis present challenges when extrapolating to how the human immune response will respond to a salivary protein-based vaccination platform (Box 1). More robust data extend from a recently developed ZIKV nonhuman primate model, in which investigators demonstrate a novel system in which rhesus macaques were successfully challenged with ZIKV via infected A aegypti bite, leading to significantly different ZIKV tissue distribution, sequence heterogeneity, and diversity between bite- versus needle-challenged animals [52]. Given that bite-challenged macaques more closely resembled human ZIKV infection [52], it is useful to consider challenging more animal hosts using a natural bite model of transmission with the appropriate vector as arboviral vaccine development accelerates. As seen with the first tick-targeted vaccine for East Coast Fever, early evaluation of vector-borne disease vaccines in biologically relevant challenge systems is necessary [17].
Translation to the Clinic
Clinical manifestations of arbovirus infection may include cutaneous signs and symptoms, with a wide range of severity from maculopapular exanthems to papulovesicular enanthems to hemorrhagic or necrotic lesions [25]. Human skin explant models, which typically needle-inoculate virus into skin donated from patients undergoing abdominoplasties, have provided some in situ evaluations of arboviral tropism for resident skin immune cells [53, 54]. However, understanding how mosquito saliva proteins affect the function and migration of immune cells in the skin is the first step in a comprehensive evaluation of the human host response to these antigens.
To date, no human studies evaluating mosquito salivary protein-based vaccines have been published, although Phase 1 clinical trials are underway in healthy volunteers living in nonendemic areas [22]. Yet, the host immune response to vector bites—particularly in the skin—may be different in endemic areas given an individual’s lifelong exposure to certain mosquito species and the arboviruses they carry [55]. Two studies in dengue-endemic South America confirm human immunoglobulin G antibody responses as corollaries of Aedes exposure, but these responses appear to be short-lived without ongoing exposure to Aedes mosquitos, which could pose problems for vaccine development (Box 1) [56, 57]. This observation may prompt clinical studies to better characterize the immunomodulation in the dermis of multiple exposures to a mosquito vector over time. Such immunomodulation may justify a quicker transition from Phase 1 safety trials to field trial sites in endemic areas to assess vaccine effectiveness. Without a firm grasp of both innate and adaptive responses to the mosquito bite, including after short- and long-term exposure to vector saliva, manipulation of this milieu to affect arthropod-borne disease initiation is difficult. Using this information to guide future vaccine design would be beneficial for all mosquito-borne diseases from malaria to emerging arboviruses such as ZIKV. As seen in leishmaniasis [20], pathogen-targeting vaccines complemented by vector salivary antigens may yield the most promising results.
CONCLUSIONS
Arboviruses are increasingly a global health threat. For many of these viruses, we lack both effective preventive and therapeutic defenses outside of conventional personal protective gear against mosquitos and supportive care for those who become ill. When a new mosquito-borne virus emerges or re-emerges, only then do substantial vaccine development efforts begin. This reactionary approach to emerging arboviruses has fortunately resulted in accelerated development of CHIKV and ZIKV vaccine candidates. However, licensed vaccines may not be available until after the the epidemic has waned, leaving public health officials without effective vaccines at the height of a health crisis. Once a vaccine is developed, the evaluation of its efficacy proves difficult because incidence has dropped, thereby impeding its progress in a regulatory pathway towards approval [57]. If more “universal” vaccines derived from mosquito saliva were developed, they could be readily available to offer some protection, even if short-term, in the emergent setting of an arboviral epidemic.
Novel and creative approaches are needed as vector-borne pathogens continue to emerge. It is now clear that vector saliva determinants dictate successful early infection and dissemination of the virus. Acceleration of mosquito saliva-based vaccine candidates into clinical trials is one of the next critical steps in expanding the armamentarium against arboviral epidemics.
Notes
Acknowledgments. We thank Ryan Kissinger, Visual Medical Arts, Research and Technologies Branch, National Institute of Allergy and Infectious Diseases, National Institutes of Health (NIH), for the artwork.
Disclaimer. The content is solely the responsibility of the authors and does not necessarily represent the official views of the National Institutes of Health.
Financial support. This work was funded by the Intramural Research Program of the NIH, National Institute of Allergy and Infectious Diseases.
Potential conflicts of interest. All authors: No reported conflicts of interest. All authors have submitted the ICMJE Form for Disclosure of Potential Conflicts of Interest. Conflicts that the editors consider relevant to the content of the manuscript have been disclosed.
References