-
PDF
- Split View
-
Views
-
Cite
Cite
Helene L E Midttun, Nathalie Acevedo, Per Skallerup, Sara Almeida, Kerstin Skovgaard, Lars Andresen, Søren Skov, Luis Caraballo, Irma van Die, Claus B Jørgensen, Merete Fredholm, Stig M Thamsborg, Peter Nejsum, Andrew R Williams, Ascaris Suum Infection Downregulates Inflammatory Pathways in the Pig Intestine In Vivo and in Human Dendritic Cells In Vitro, The Journal of Infectious Diseases, Volume 217, Issue 2, 15 January 2018, Pages 310–319, https://doi.org/10.1093/infdis/jix585
- Share Icon Share
Abstract
Ascaris suum is a helminth parasite of pigs closely related to its human counterpart, A. lumbricoides, which infects almost 1 billion people. Ascaris is thought to modulate host immune and inflammatory responses, which may drive immune hyporesponsiveness during chronic infections. Using transcriptomic analysis, we show here that pigs with a chronic A. suum infection have a substantial suppression of inflammatory pathways in the intestinal mucosa, with a broad downregulation of genes encoding cytokines and antigen-processing and costimulatory molecules. A. suum body fluid (ABF) suppressed similar transcriptional pathways in human dendritic cells (DCs) in vitro. DCs exposed to ABF secreted minimal amounts of cytokines and had impaired production of cyclooxygengase-2, altered glucose metabolism, and reduced capacity to induce interferon-gamma production in T cells. Our in vivo and in vitro data provide an insight into mucosal immune modulation during Ascaris infection, and show that A. suum profoundly suppresses immune and inflammatory pathways.
Approximately 1 billion people worldwide are estimated to be infected with the soil-transmitted helminth Ascaris lumbricoides, causing substantial morbidity [1]. A. lumbricoides and its pig counterpart, A. suum, are morphologically indistinguishable, and closely related genetically [2]. A. suum is ubiquitous in pigs, affecting feed utilization and growth performance [3]. The success of Ascaris and many other helminths to infect their hosts may be partly due to their ability to establish chronic infections, which may be achieved by active modulation of the host immune response [4]. In this way, helminths including Ascaris can suppress both parasite-specific and bystander immune responses, leading to a state of immune hyporesponsiveness which, apart from promoting chronic helminth infections, may interfere with the ability of the host to mount effective responses to other pathogens and to vaccines. In humans, A. lumbricoides infection may prevent effective induction of immune response to cholera vaccines, modulate host responses to other intestinal pathogens such as Giardia, and alter the pathogenicity of malaria coinfections [5–10]. In addition, in heavily infected regions helminthiases, including ascariasis, have been associated with low frequency of both allergic symptoms and skin test positivity [11]. Furthermore, A. lumbricoides cystatin has been shown to ameliorate symptoms in a mouse model of dextran sodium sulfate-induced colitis [12]. In pigs, efficacy of vaccination against Mycoplasma is compromised by concurrent A. suum infection, and exposure to A. suum may increase the risk of viral coinfection [13, 14].
The immunoregulatory effects of helminths are widely investigated and the mechanisms by which they suppress host immunity are beginning to be elucidated. Helminths induce Th2-biased immune responses whereby T-helper cells secrete IL-4 and IL-13 and suppress IFN-γ production, thereby inhibiting Th1 and Th17 responses [4, 15–18]. Moreover, many helminths are strong inducers of T-regulatory cells, driving a state of immuneanergy [19, 20]. Suppression of Th1 and Th17 function may be driven in part by modulation of dendritic cell (DC) and macrophage activity, as it is apparent that helminths can impair proinflammatory activity and T-cell–activating capacity in DCs [21, 22], and modulate macrophage function towards an anti-inflammatory phenotype [12, 23]. Consistent with this, peripheral blood leukocytes from humans infected with helminths have increased production of the regulatory/anti-inflammatory cytokine IL-10 following mitogenic stimulation [24–26].
Given the similarities between host physiology and parasite species, A. suum infection in pigs represents an excellent model for investigating host-parasite interactions of A. lumbricoides, and provides an opportunity to examine in detail the host-parasite interaction in the gastrointestinal tract. Acute A. suum infection induces stereotypical Th2-effector mechanisms such as eosinophilia and increased gut motility, leading to expulsion of the majority of the parasites at the fourth larval stage [27, 28]. However, in some animals, worms may continue to develop to the adult stage and establish a chronic infection, which may persist for 1–2 years. Our aim was to examine the effect of chronic infection on the immunological milieu in the pig intestine and possible mechanisms involved. To this end, we generated a transcriptional snapshot of the mucosal environment in pigs infected with A. suum. Combined with transcriptomics and gene pathway analyses of in vitro cultures of human monocyte-derived DCs exposed to antigen from adult A. suum, we show that A. suum strongly modulates host immune and inflammatory responses to drive a stage of immune anergy. Our results increase our understanding of the mechanisms underlying the chronic nature of Ascaris infections.
METHODS
In Vivo Experiment
The design of the pig experiment has been described previously [29, 30]. Briefly, crossbred Duroc/Danish Landrace/Yorkshire pigs of 2 genotypes were trickle-infected with A. suum eggs (25 eggs/kg/day) twice per week for 8 weeks or left as uninfected controls. Pigs were euthanized on days 55–59 post-first infection (PI). The study was approved by the Danish Animal Experimentation Inspectorate (License number 2010/561–1914). Mucosal samples were obtained from the central part of the jejunum, rinsed with saline, and snap-frozen in liquid nitrogen. Thirteen pigs harbored macroscopic worm burdens at this time point and were deemed to be chronically infected. Due to limited space on the array chip, 10 infected pigs and 9 uninfected controls were chosen on the basis of RNA quality (RIN values) for microarray (see Section Microarrays). Initial analysis of these data showed no significant differences between genotypes, and thus both genotypes were pooled for subsequent analysis.
Ascaris antigen
A. suum antigenic body fluid (ABF) was obtained from fresh adult A. suum worms as previously described [31].
Microarrays
RNA was extracted from jejunum mucosa by the phenol/chloroform method and RNA quality assessed using Bioanalyzer (Agilent Technologies). cDNA synthesis was done using the Whole Transcriptome (WT) Expression kit (Ambion), samples were fragmented and labeled with the Gene Chip WT Terminal Labeling kit (Affymetrix), and genome-wide expression was analyzed by the Affymetrix WT Assay Porcine Gene 1.1 ST in the Gene Titan Platform. RNA was extracted from human cells using RNAeasy mini kits (Qiagen) and the quality assessed using the Experion platform (Biorad). High-quality RNA (RIN > 9) was used for microarray, which was performed by AROS Biotech A/S (Aarhus, Denmark) using the Illumina HT-12 v4.0 BeadArray platform. Data were quantile normalized and log2 transformed prior to statistical analysis using statistical analysis for microarrays [32]. For the statistical analysis, only genes significantly expressed in at least one of the treatment groups were included, and predicted genes and genes with no gene symbol were removed. Gene set enrichment analysis (GSEA) was used for pathway analysis (Broad Institute, http://software.broadinstitute.org) using canonical pathways v5.1. Pig and human microarray data have been uploaded to GEO under accession numbers GSE104092 and GSE102895, respectively.
Human Cell Isolation and Differentiation
Buffy coats were obtained from anonymous volunteers following written informed consent (Copenhagen University Hospital). Peripheral blood mononuclear cells (PBMC) were isolated on Histopaque (Sigma-Aldrich), and monocytes were then purified using anti-CD14 microbeads and magnetic separation (MACS, Miltenyi Biotech). Mature DCs were obtained from monocytes by 4 days differentiation followed by addition of lipopolysaccharide (LPS; 10 ng/mL; Sigma-Aldrich) as previously described [33]. DCs were either incubated with ABF (10 µg/mL) or phosphate-buffered saline (PBS) as a control 30 minutes prior to the addition of LPS. For quantitative polymerase chain reaction (qPCR) analysis, cells were harvested 6 hours after LPS addition; for cytokine enzyme-linked immunosorbent assay (ELISA) and western blot analyses, cells or culture supernatants were harvested after 24 hours. Experiments with DCs were repeated 3–5 times, each time using cells from a new blood donor.
Quantitative PCR
For pig samples, 2 cDNA replicates were synthesized for each RNA sample. First-strand cDNA synthesis, including gDNA removal, was performed using the QuantiTect Reverse Transcription Kit (Qiagen) according to the manufacturer’s instructions. qPCR was performed using 96.96 dynamic arrays (Fluidigm, CA) as described previously [31]. qPCR was carried out on a BioMark HD Reader (Fluidigm) using the following conditions: 2 minutes at 50°C, 10 minutes at 95 °C followed by 35 cycles of 15 seconds at 95°C and 1 minute at 60°C. Experimental data were normalized against a mean of the reference genes ACTB, B2M, TBP, RPL13A, and GAPDH, which were selected using NormFinder [34] and GeNorm [35]. Duplicate cDNA samples were averaged after normalization, and log2 transformed prior to statistical analysis. Primer sequences are listed in Supplementary Table 1.
For human samples, cDNA was synthesized using qScript cDNA SuperMix (Quanta Biosciences). Primer sequences are listed in Supplementary Table 2. Samples were prepared as follows: 2 × PerfeCTa SYBR Green fastmix (Quanta Biosciences), 5 pmol/µL forward primer and reverse primer, 10 µg cDNA sample, RNAse-free water to a total of 20 µL. A 2-step qPCR program was used for all downregulated genes: an initial denaturation step at 95°C for 30 seconds followed by 40 cycles of 15 seconds at 95°C and 30 seconds at 60°C. RPLP0 was used as a reference gene for normalization. Expression was calculated as copy number based on values relative to the quantity of the reference gene.
ELISA
Secreted tumor necrosis factor-alpha (TNF-α), interleukin-6 (IL-6), IL-12p70, IL-10, and IL-23 were detected with commercial antibody pairs (Thermo Fisher Scientific). CXCL1 was detected with an ELISA kit (Quantakine ELISA, R&D systems), according to the manufacturer’s instructions.
Western Blot
Cell pellets were lysed in RIPA buffer (Thermo Fisher Scientific). Sodium dodecyl sulfate polyacrylamide gel electrophoresis (SDS-PAGE) was performed using NuPAGE lithium dodecyl sulfate (LDS) sample buffer (Thermo Fisher Scientific) and dl-dithiotreitol (Sigma-Aldrich), and a 10% agarose gel. The gel was blotted to a membrane via iBlot (Thermo Fisher Scientific), according to the manufacturer’s protocol. COX2 was detected with rabbit antihuman COX2 (Cell Signaling Technology) and mouse antihuman glyceraldehyde-3-phosphate dehydrogenase (GAPDH) (GenScript) was used as a reference. Donkey antirabbit and donkey antimouse (LI-COR®) were used as secondary antibodies. Results were visualized on LI-COR Odyssey Fc and analyzed by Image Studio v5.2.
Glucose Uptake and Lactate Production in Dendritic Cells
DCs were incubated with 10 µM fluorescent glucose (2NBDG, Thermo Fisher Scientific) together with LPS and/or ABF (50 µg/mL) or were left unstimulated. Cells were incubated for either 1, 2, or 24 hours, washed twice with PBS, and glucose uptake measured using an Accuri C6 flow cytometer (BD Biosciences). To measure lactate production, DCs were stimulated as described above, and lactate concentrations were measured using Accutrend® Lactate (Roche).
IFN-γ Production in T-cell Cultures
DCs were matured for 48 hours with either LPS (10 ng/mL) or LPS together with ABF (20 µg/mL). DCs were then washed, counted, and added to naive, CD4+ T cells (isolated using the naive T-cell MACS kit, Miltenyi Biotech) at a 1:10 DC:T-cell ratio. T cells were expanded using recombinant human IL-2 for 10–12 days, after which they were restimulated with Phorbol 12-myristate 13-acetate, ionomycin, and brefeldin A, and intracellular interferon-gamma (IFN-γ) production was measured by flow cytometry as described previously [33].
Statistical Analyses
Where indicated, paired or unpaired 2-tailed t tests were carried out using Graphpad prism v7.0 (Graphpad Software, San Diego, CA). In the case of lactate production, a 1-tailed t test was used as we previously hypothesized that lactate would be reduced based on the results of the GSEA.
RESULTS
Chronic Infection with A. suum Downregulates Inflammatory and Antigen-processing Pathways in the Intestinal Mucosa
To investigate the effect of chronic A. suum infection in pigs in vivo, microarray analysis was carried out to compare intestinal tissue from pigs infected with adult worms and naive, uninfected pigs. Infected pigs showed a significant downregulation of 99 genes as compared to naive pigs, while there was a significant upregulation of only 9 genes (Supplementary Table 3). A summary of the most significantly up- and downregulated genes is presented in Figure 1. Notably, many of the downregulated genes encoded cytokines/chemokines, inflammatory effector molecules, and antigen processing and costimulatory molecules. Several cytokines from the interleukin (IL) family and chemokines from the C-X-C motif ligands (CXCL) family were particularly affected (Figure 1A). The most upregulated gene was GSDMC, encoding gasdermin-C, a protein whose function is not well known but may be involved in pyroptosis and antimicrobial activity [36]. qPCR analysis on the mucosal samples confirmed the results of the microarray, with significant downregulation of notable genes such as IFNG and CXCL11, and upregulation of GSDMC as well as the eosinophil chemoattractant CCL26 (Figure 1B). The microarray data were used for GSEA to identify significantly up- and downregulated gene pathways (Supplementary Table 4). Of the most significantly downregulated pathways (Q value < 0.005; Table 1), the vast majority (20/22) were involved in inflammation, cytokine production, and antigen processing, further indicating that chronic A. suum infection modulates the local immune environment in the intestine. Pathways that were upregulated were primarily involved in energy metabolism; however, none of these reached significance when corrected for false-discovery rate (Q > 0.1; Supplementary Table 4).
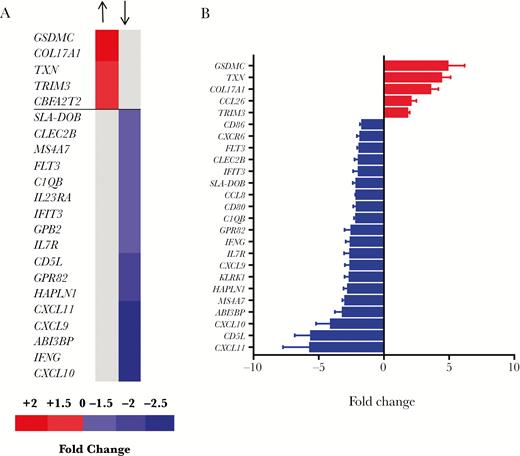
Ascaris suum infection downregulates immune and inflammatory-related genes in the pig jejunal mucosa. A, Differentially expressed genes identified by microarray in the jejunal mucosa of pigs infected with adult Ascaris suum, relative to uninfected pigs. Shown are all significantly upregulated genes and the top 20 downregulated genes as ranked by fold change (see Supplementary Material for full list of all downregulated genes). B, Quantitative PCR validation of selected differentially expressed genes, ranked by fold change. Shown is the mean ± SEM For all genes, relative expression is different between infected and uninfected pigs (P < .05 by Student t test), except IFIT3 (P = .065).
Down-regulated Gene Pathways (Q value < 0.005) Identified by Gene-set Enrichment Analysis in Pigs Chronically Infected With Ascaris suum Compared to Uninfected Control Animals
Pathway . |
---|
PID IL12-2 pathwaya |
KEGG toll like receptor signaling pathwaya |
REACTOME immune systema |
KEGG systemic lupus erythematosusa |
REACTOME chemokine receptors bind chemokinesa |
KEGG natural killer cell mediated cytotoxicitya |
REACTOME interferon signalinga |
KEGG T-cell receptor signaling pathwaya |
REACTOME immune-regulatory interactions between a lymphoid and a non-lymphoid cell |
PID IL23 pathwaya |
PID CD8 TCR downstream pathwaya |
REACTOME interferon alpha beta signalinga |
REACTOME adaptive immune system |
PID IL27 pathwaya |
REACTOME cytokine signaling in immune systema |
REACTOME costimulation by the CD28 familya |
KEGG cytokine-cytokine receptor interactiona |
KEGG chemokine signaling pathwaya |
KEGG primary immune-deficiency |
PID IL12 STAT4 pathwaya |
REACTOME interferon gamma signalinga |
KEGG Leishmania infectiona |
Pathway . |
---|
PID IL12-2 pathwaya |
KEGG toll like receptor signaling pathwaya |
REACTOME immune systema |
KEGG systemic lupus erythematosusa |
REACTOME chemokine receptors bind chemokinesa |
KEGG natural killer cell mediated cytotoxicitya |
REACTOME interferon signalinga |
KEGG T-cell receptor signaling pathwaya |
REACTOME immune-regulatory interactions between a lymphoid and a non-lymphoid cell |
PID IL23 pathwaya |
PID CD8 TCR downstream pathwaya |
REACTOME interferon alpha beta signalinga |
REACTOME adaptive immune system |
PID IL27 pathwaya |
REACTOME cytokine signaling in immune systema |
REACTOME costimulation by the CD28 familya |
KEGG cytokine-cytokine receptor interactiona |
KEGG chemokine signaling pathwaya |
KEGG primary immune-deficiency |
PID IL12 STAT4 pathwaya |
REACTOME interferon gamma signalinga |
KEGG Leishmania infectiona |
Indicates pathway involved in inflammation.
KEGG, Kyoto Encyclopedia of Genes and Genomes (www.genome.jp/kegg); PID, Pathway Interaction Database (www.ndexbio.org); REACTOME database (https://reactome.org/).
Down-regulated Gene Pathways (Q value < 0.005) Identified by Gene-set Enrichment Analysis in Pigs Chronically Infected With Ascaris suum Compared to Uninfected Control Animals
Pathway . |
---|
PID IL12-2 pathwaya |
KEGG toll like receptor signaling pathwaya |
REACTOME immune systema |
KEGG systemic lupus erythematosusa |
REACTOME chemokine receptors bind chemokinesa |
KEGG natural killer cell mediated cytotoxicitya |
REACTOME interferon signalinga |
KEGG T-cell receptor signaling pathwaya |
REACTOME immune-regulatory interactions between a lymphoid and a non-lymphoid cell |
PID IL23 pathwaya |
PID CD8 TCR downstream pathwaya |
REACTOME interferon alpha beta signalinga |
REACTOME adaptive immune system |
PID IL27 pathwaya |
REACTOME cytokine signaling in immune systema |
REACTOME costimulation by the CD28 familya |
KEGG cytokine-cytokine receptor interactiona |
KEGG chemokine signaling pathwaya |
KEGG primary immune-deficiency |
PID IL12 STAT4 pathwaya |
REACTOME interferon gamma signalinga |
KEGG Leishmania infectiona |
Pathway . |
---|
PID IL12-2 pathwaya |
KEGG toll like receptor signaling pathwaya |
REACTOME immune systema |
KEGG systemic lupus erythematosusa |
REACTOME chemokine receptors bind chemokinesa |
KEGG natural killer cell mediated cytotoxicitya |
REACTOME interferon signalinga |
KEGG T-cell receptor signaling pathwaya |
REACTOME immune-regulatory interactions between a lymphoid and a non-lymphoid cell |
PID IL23 pathwaya |
PID CD8 TCR downstream pathwaya |
REACTOME interferon alpha beta signalinga |
REACTOME adaptive immune system |
PID IL27 pathwaya |
REACTOME cytokine signaling in immune systema |
REACTOME costimulation by the CD28 familya |
KEGG cytokine-cytokine receptor interactiona |
KEGG chemokine signaling pathwaya |
KEGG primary immune-deficiency |
PID IL12 STAT4 pathwaya |
REACTOME interferon gamma signalinga |
KEGG Leishmania infectiona |
Indicates pathway involved in inflammation.
KEGG, Kyoto Encyclopedia of Genes and Genomes (www.genome.jp/kegg); PID, Pathway Interaction Database (www.ndexbio.org); REACTOME database (https://reactome.org/).
Ascaris suum Body Fluid Downregulates Inflammatory Pathways in Human Dendritic Cells In Vitro
Many of the gene pathways downregulated in A. suum-infected pigs were connected to toll-like receptor (TLR) ligation and inflammatory cytokine production. These processes are closely linked to DC function, and human DC activity is known to be modulated by helminths such as Trichuris suis and Schistosoma mansoni [21, 37]. To explore whether modulation of DC activity may contribute to the observed in vivo results, microarray analysis was also carried out on human monocyte-derived DCs cultured with either LPS alone or LPS and ABF. Cell viability was not affected by ABF (data not shown). Similar to the microarray findings using pig intestinal tissue, ABF treatment significantly downregulated many (>100) genes in DCs, whereas only 19 genes were significantly upregulated (Supplementary Table 5). Significantly up- and downregulated genes with at least a 2-fold expression change are shown in Figure 2A. The ABF treatment induced a generalized downregulation of cytokines and chemokines, as well as the prostaglandin synthase PTGES2 (encoding cyclooxygenase-2, COX2), and genes involved in cell adhesion and migration. The 2 upregulated genes were NRROS, also known as LRRC33, a negative regulator of reactive oxygen species (ROS), and STAMBPL1, which encodes a protease whose function is unclear but may be involved in NF-κB regulation [38]. The upregulation of NRROS is notable given that TXN, encoding the antioxidant protein thioredoxin, was significantly upregulated in the mucosa of infected pigs (Figure 1). This may suggest that A. suum inhibits inflammatory responses by modulating ROS production. Thus, ABF treatment of human DCs in vitro induces a clear anti-inflammatory effect, consistent with the in vivo results.
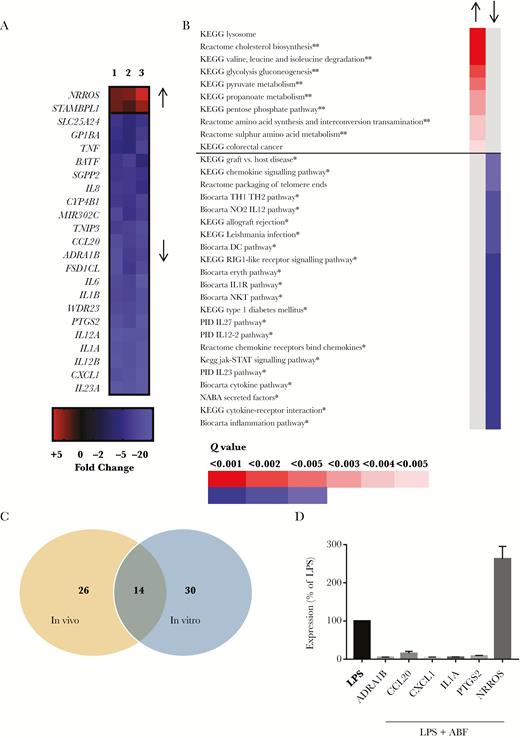
Ascaris suum adult body fluid (ABF) downregulates inflammatory pathways in lipopolysaccharide (LPS)-stimulated dendritic cells (DCs). A, Differentially expressed genes identified by microarray in DCs exposed to ABF and LPS (n = 3), relative to DCs cultured with only LPS (n = 3). Shown are significantly regulated genes with a fold-change of at least 2 (see Supplementary Material for full list of all downregulated genes). B, Gene pathways altered by ABF treatment in DCs, as determined by gene-set enrichment analysis. Shown are upregulated pathways with Q value < 0.05, and downregulated pathways with Q value < 0.005 (see Supplementary Material for full list of all differentially regulated pathways). ** Indicates pathway involved in metabolism, * indicates pathway involved in inflammation C, Unique and common gene pathways modulated by A. suum infection in vivo, and ABF treatment of DCs in vitro. D, Quantitative PCR validation of selected differentially expressed genes in DCs exposed to ABF and LPS (n = 5), relative to LPS alone (n = 5). For all genes, expression is significantly different (P < .001) relative to LPS treatment alone. Shown is the mean ± SEM.
Using GSEA, we showed that ABF downregulated 40 pathways in human DCs (Q value < 0.05; Supplementary Table 6). Pathways with a Q value of < 0.005 are shown in Figure 2B; of the 22 downregulated pathways, 21 are involved in inflammation, corroborating the results obtained in vivo in pigs. Notably, 14 of the significantly downregulated pathways were identical in the in vivo and in vitro studies, suggesting that modulation of TLR-driven responses by A. suum is a major mechanism employed by the parasite in vivo (Figure 2C). Conversely, 8 out of the 10 upregulated gene pathways in DCs were involved in metabolic function such as glucose and propionate metabolism (Figure 2B).
qPCR validation confirmed the results of the microarray, showing strong suppression of mRNA expression of ADRA1B, CCL20, CXCL1, IL1-α, and PTGES2 in DCs treated with both LPS and ABF as compared to DCs treated with only LPS (P < .0001 for all genes; Figure 2D), and increased expression of NRROS in DCs treated with LPS and ABF compared to only LPS (P < .001; Figure 2D).
ABF Regulates Cytokine Secretion and COX2 Expression in Dendritic Cells
To assess the functional activity of DCs exposed to ABF, we first measured secretion of IL-23, IL-12p70, TNF-α, IL-6, IL-10, and CXCL1 24 hours following LPS stimulation. No secretion was evident in DCs not treated with LPS, regardless of ABF treatment (data not shown). In LPS-treated cells, there was a strong suppression of cytokine secretion following ABF treatment (P < .001 for all cytokines; Figure 3A). The reduction in cytokine production may be related to inhibition of COX2 activity, as PTGES2 mRNA expression was strongly suppressed. To confirm this, we also assessed COX2 protein levels in LPS-treated DCs with and without concurrent ABF treatment, and observed significant suppression of COX2 by ABF (Figure 3B).
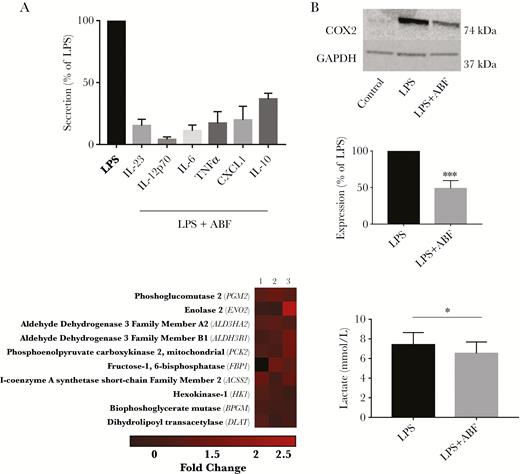
Ascaris suum adult body fluid (ABF) suppresses lipopolysaccharide (LPS)-induced cytokine secretion, cyclooxygenase (COX-2) expression, and lactate production in dendritic cells (DCs). A, Secretion of cytokines in DCs stimulated with LPS and ABF, relative to LPS only. For all cytokines, secretion is significantly different (P < .01) relative to LPS alone. The experiments were repeated at least 3 times, each time using cells from a different blood donor. Shown is the mean ± SEM. B, Reduction of LPS-induced COX-2 expression in DCs by ABF (n = 4). Shown is representative western blot from 1 experiment, as well as the mean ± SEM. ***P < .01 by Student t test. C, Modulation of genes involved in glucose metabolism in DCs stimulated with LPS plus ABF, compared to LPS alone (each n = 3). Shown are the top 10 upregulated genes in the KEGG glycolysis-gluconeogenesis pathway identified by gene-set enrichment analysis. D, Reduction of lactate production in LPS-stimulated DCs by ABF (n = 3). *P < .05 by paired 1-tailed t test.
ABF Does Not Reduce Glucose Uptake But Suppresses Lactate Production in Dendritic Cells
GSEA indicated that a number of pathways involving glucose metabolism were regulated by ABF in LPS-activated DCs. Inspection of the Kyoto Encyclopedia of Genes and Genomes (KEGG) glycolysis-gluconeogenesis pathway revealed that expression of a number of genes related to the citric-acid cycle and mitochondrial oxidative phosphorylation (such as DLAT, PCK2, HK1, and ACCS2) were higher in cells treated with LPS and ABF relative to those treated only with LPS (Figure 3C; see Supplementary Figure 1 for the full KEGG pathway). Although GSEA did not identify any significantly downregulated genes in the pathway, it was interesting to note that amongst the lowest expressed genes in cells treated with ABF were those encoding key glycolytic enzymes such as PFKL1 and TPI1 (Supplementary Figure 1). To investigate functional changes of ABF on cellular metabolism in DCs, glucose uptake and lactate production in LPS-activated DCs were measured. Glucose uptake was not different in DCs exposed to LPS alone or LPS and ABF (data not shown). However, lactate production was lower in DCs cotreated with LPS and ABF as compared to only LPS (P < .05; Figure 3D), suggesting that ABF suppressed the utilization of glucose by glycolysis in LPS-stimulated DCs.
IFN-γ Production in Naive T cells is Suppressed by ABF-treated Dendritic Cells
We noted that IFN-γ gene expression was significantly suppressed in the jejunal mucosa of pigs infected with A. suum, which would be consistent with a strong suppression of proinflammatory cytokine production from DCs. To assess whether DCs exposed to ABF impaired subsequent IFN-γ production in T cells, we cocultured DCs and naive CD4+ T cells and assessed IFN-γ production. DCs activated with LPS and ABF suppressed (P < .05) IFN-γ production in allogenic T cells following restimulation compared to DCs activated with LPS alone (Figure 4). IL-4 production in the T cells was unaffected by exposure of DCs to ABF (data not shown), suggesting that DCs exposed to ABF selectively modulate Th1 cytokine responses in naive T cells.
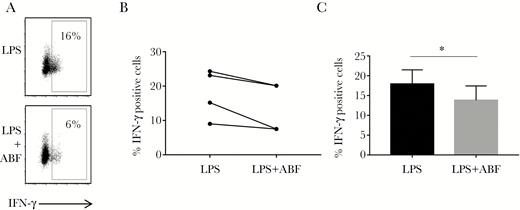
Dendritic cells (DCs) exposed to Ascaris suum adult body fluid (ABF) suppress IFN-γ production in CD4+ T cells. A, Representative flow cytometry plot showing reduction in IFN-γ production in human CD4+ T cells following activation by DCs matured with either lipopolysaccharide (LPS) or LPS and ABF for 48 hours. B, Results from 4 independent experiments, each representing DCs from a different donor, showing reduction in IFN-γ–positive cells, C, Mean ± SEM of experiments depicted in (B). * P < .05 by paired t test.
DISCUSSION
Immune hyporesponsiveness or anergy in humans as a result of helminth infection has been demonstrated by reduced proliferative responses and increased production of IL-10 in peripheral lymphocytes following stimulation with mitogens or specific antigens [4, 24]. However, studies in humans are generally limited to the activities of blood lymphocytes. Here, we have demonstrated that subclinical A. suum infection in pigs, which may be expected to reasonably resemble ascariasis in humans, profoundly modulates the immunological milieu in the intestinal mucosa, with a downregulation of transcriptional pathways related to immune function, specifically proinflammatory, Th1, and antigen processing pathways.
DCs play a key role in both immune homeostasis and initiation of immune responses to invading pathogens. DCs display a broad range of TLRs which are specially equipped for recognizing bacterial and viral products, and subsequently secrete cytokines such as TNF-α and IL-6, which help stimulate Th1-type T-cell activity after presentation of MHC-bound antigens. Specific inhibition of Th1-promoting activity in DCs may favor Th2 and/or T-regulatory responses, by inhibiting IFN-γ production in T cells and thereby promoting IL-4 responses [15, 21]. Previous reports suggest that products from a number of helminths, including A. lumbricoides, modulate TLR-driven responses in human DCs in vitro [39, 40]. Therefore, the observed downregulation of inflammatory pathways in A. suum-infected pigs prompted us to examine whether exposure of human DCs to A. suum products induced a similar modulatory effect. The concordance between our in vitro and in vivo data suggests that a key mechanism of Ascaris-induced immune modulation may be inhibition of DC activity in the intestinal mucosa.
Interestingly, our data show that many gene pathways were downregulated, whilst only a small number were upregulated. This is suggestive of a broad immunosuppressive effect, rather than a selective suppression of some pathways and upregulation of others, for example regulatory networks. Moreover, ABF treatment of DCs resulted in reduced LPS-induced secretion of both inflammatory cytokines such as IL-23 and also the regulatory cytokine IL-10. Consistent with this, others have previously noted that suppression of allergic inflammation by ABF in mice is maintained in IL-10–deficient mice [41], suggesting that stimulation of IL-10 production is not a major mechanism driving Ascaris-induced immune modulation. For now, the precise molecular mechanisms governing this immunosuppressive effect remain unresolved. Notably, our pathway analysis of ABF-exposed DCs also suggested a modulatory effect on glucose metabolism following LPS treatment, which was supported by significantly reduced lactate production in these cells. Regulation of metabolic activity is increasingly recognized as a major factor governing the maturation and activation of DCs, macrophages, and T cells. Immature and resting DCs and macrophages normally rely on oxidative phosphorylation for their energy requirements due to its high overall efficiency, but in times of rapid energy demand (eg, following TLR stimulation) a shift towards anaerobic glycolysis (and lactate production) is normally observed to allow rapid ATP production [42]. Our results are consistent with data showing that S. mansoni antigens inhibit glycolysis in murine macrophages [43], and suggest that interference with TLR-driven immunometabolism may be a contributor to helminth-induced immunosuppression.
Our results contribute to interpretation of the host-parasite interactions in Ascaris-endemic regions and in A. suum-positive pig herds. In A. suum-infected pigs, bacterial coinfections can be increased [14], and in humans the pathology of other parasitic infections such as malaria also appears to be affected, although with conflicting reports of the nature of the interaction. There are difficulties in interpreting field studies as subjects are invariably infected with other soil-transmitted helminths such as hookworms. An increase in pathology has been observed in malaria patients coinfected with soil-transmitted helminths [6]; however, in other studies severe malaria is less prevalent in helminth-infected patients [5]. This complexity may relate to the fine balance in immunopathology that arises in malarial infections: a strong proinflammatory response may be necessary to clear infections, but complications such as cerebral malaria are linked to excessive inflammation caused by production of cytokines such as IFN-γ. Therefore, if IFN-γ production is suppressed by helminth infection, depending on the context, coinfections may be exacerbated, or pathological symptoms may be reduced.
In conclusion, we have shown for the first time that Ascaris infection in pigs suppresses transcriptional pathways related to inflammatory responses. This may be linked to the profound effect of Ascaris antigen on DCs. Our results may aid interpretation of interactions between helminth infection and vaccination/coinfections, and contribute to our understanding of how helminths modulate host immune function.
Supplementary Data
Supplementary materials are available at The Journal of Infectious Diseases online. Consisting of data provided by the authors to benefit the reader, the posted materials are not copyedited and are the sole responsibility of the authors, so questions or comments should be addressed to the corresponding author.
Notes
Acknowledgments. We are grateful to Adriana Bordacelly, Susanna Cirera, and Karin Tarp for assistance.
Financial support. This work was supported by grants from the Danish Council for Independent Research (Technology and Production Sciences 12-126630 to A. R. W.; 271-09-0162 and 6111-00521 to P. N.) and the Lundbeck Foundation (14-3670A to A. R. W.).
Potential conflicts of interest. All authors: No reported conflicts of interest. All authors have submitted the ICMJE Form for Disclosure of Potential Conflicts of Interest. Conflicts that the editors consider relevant to the content of the manuscript have been disclosed.
References
Author notes
P. N. and A. R. W. are cosenior authors.