-
PDF
- Split View
-
Views
-
Cite
Cite
Scott William Roy, On the Form and Origins of the Bizarre Sex Chromosomal System of the Mandarin Vole, Journal of Heredity, Volume 112, Issue 4, June 2021, Pages 328–334, https://doi.org/10.1093/jhered/esab020
- Share Icon Share
Abstract
Recent work has illuminated the bizarre sex chromosomal system of the mandarin vole, Lasiopodomys mandarinus. The ancestral sex chromosomes have been replaced by 4 neo-sex chromosomes. These sex chromosomes show non-Mendelian inheritance and epistatic sex determination, as well as unaccounted-for karyotype frequencies. I suggest a model to account for the complex observed inheritance patterns. The proposed model combines putative adaptations previously observed in rodents, including feminizing X chromosomes and Y-biased spermatogenesis, with a novel proposed mechanism of genomic imprinting of X-linked genes during oogenesis in XY females. Alternative possibilities are also discussed. The proposed scenario provides a relatively simple and testable model for the function and origins of a remarkably complex mammalian sex chromosomal system.
In contrast to the general stability of sex chromosomes across therian mammals, sex chromosomes of rodents show a variety of atypical features, including loss, or conversely amplification, of the ancient sex-determining gene SRY, loss or fusion of the ancestral Y chromosome, evolution of atypical karyotypic patterns (e.g., XO/XO or XY/XO males/females), movement of ancient Y-linked genes onto the X chromosome, the evolution of so-called “feminizing X” and “feminizing Y” chromosomes that reverse the sexual development of XY individuals, sex chromosome drive in both oogenesis and spermatogenesis, and massive expansion of sex chromosomes (e.g., Jiménez et al. 1991; Charlesworth and Dempsey 2001; Sutou et al. 2001; Burt and Trivers 2009; Acosta et al. 2011; Matveevsky et al. 2016; Mulugeta et al. 2016; Kruger et al. 2019). The reasons for this dynamism remain obscure, and more work on these many varied systems is clearly needed.
The mandarin vole, Lasiopodomys mandarinus, has stood as one of the most enduring puzzles of sex chromosome biology (Wang et al. 2003; Chen et al. 2008; Gladkikh et al. 2016; Romanenko et al. 2020). The sex chromosomes of Lasiopodomys mandarinus show many noncanonical features: 1) absence of the ancestral mammalian Y chromosome and SRY; 2) emergence of 4 neo-sex chromosomes; 3) presence of multiple neo-X chromosomes; 4) complex sex determination, in which there is no clear one-to-one association between chromosome and sex, as would be expected for a simple X/Y or Z/W chromosome system; and 5) the absence or rarity of multiple expected karyotypes. Romanenko et al. 2020 recently made great progress in understanding this fascinating system, in particular on sex determination and the occurrence and production of different karyotypes (points 4 and 5).
In the recent study, as in previous work, 4 different morphologically defined sex chromosomes are observed, termed neo-Y and neo-X1/X2/X3 (hereafter simply Y and X1-3). Among sampled individuals, chromosomes are almost always observed in one of only 3 karyotypic combinations, namely X1YX3X3 (male), X1X2X3 (female) and X2YX3 (female). Previous surveys had noted a dearth of X1X1X3X3 individuals, which are expected as offspring in crosses between X1YX3X3 males and X1X2X3 females. Chromosome painting evidence and observed outcomes of crosses suggest that X2 represents a fusion of X1 and X3 (as frequently seen among feminizing X chromosomes; Burt and Trivers 2009), with X2 possibly pairing with both X1 and X3 during meiosis, in which case euploid gamete combinations would be limited to X1X3, YX3, and X2. To simplify our discussion, we will omit X3, whose copy number simply follows from the rest of the karyotype (2 copies in X1X1 and X1Y individuals, 1 copy in X1X2 and X2Y individuals), and which does not immediately appear to play a role in the phenomena discussed below.
Here, I propose a 3-step model for the origins of this complex system. First, the X2 chromosome acquired a feminizing effect, perhaps coincident with the chromosomal fusion. Second, in response to the overproduction of females due to the presence of the feminizing X2 chromosome in the population, Y-biased spermatogenesis evolved to promote production of sons. Third, because Y-biased spermatogenesis does not increase son production from X2Y females, X2Y females evolved a mechanism to promote production of X1-bearing offspring, perhaps through maternal imprinting of an essential X-linked gene. Following explication of this 3-step model, I discuss various alternative models that could also explain the data, but argue that the major model presented is the most likely given available data.
Step 0: Introduction to the System and Chromosomal Origins of L. mandarinus Sex Chromosomes
The dynamics proposed here take as their starting point a standard system with XY males and XX females. However, it is worth noting that this ancestral starting point is not the typical, ancestral, and nearly universal XX/XY system of mammals. Whereas the vast majority of placental mammals have X and Y chromosomes predominantly representing retention of the ancestral XX/XY system, the X and Y chromosomes of L. mandarinus are quite different, with the ancestral L. mandarinus X (called X1 below) being derived from a fusion between the ancestral mammalian X and linkage group 13, while the ancestral mammalian Y chromosome appears to have been lost, having been replaced as the Y by the so-called neo-Y chromosome, which is derived from linkage group 13 and also contains some ancestral X-linked sequence (Romanenko et al. 2020). The apparent absence of SRY and other ancestral Y-linked genes from the sex chromosomes (Romanenko et al. 2020) leaves open the question of the mechanism of sex determination for this atypical ancestral XX/XY system.
Step 1: Evolution of a Feminizing X Chromosome (X2)
By characterizing crosses between individuals of the various karyotypes, Romanenko et al. 2020 clarified the association between karyotype and sex determination. In particular, their data and models suggest the presence of a “feminizing X” chromosome (formally, a dominant W chromosome; in choosing “feminizing X” I have adhered to the convention in the rodent sex chromosome literature). In feminizing X systems, 3 types of sex chromosomes are observed: a “standard” dominant masculinizing Y chromosome (the L. mandarinus Y), a “standard” X chromosome whose presence does not affect sex determination (X1), and a “feminizing X” that suppresses the masculinization effect of the Y (X2). Feminizing X chromosomes are thought to arise because of the transmission advantage enjoyed in XY x XY crosses: because YY progeny lack essential X-linked genes and are thus inviable, X chromosomes in XY females enjoy a transmission advantage. Thus, assuming that there is at least some reproductive compensation, X chromosomes that feminize XY embryos are expected to enjoy a selective advantage (for a more in depth description of these dynamics and their condition dependence, see e.g., Bull and Bulmer 1981; Bull 1983; Burt and Trivers 2009). Figure 1A shows the general model for feminizing-X system, and Figure 1B shows the observed patterns in L. mandarinus, highlighting the similarities under a model where X2 has a feminizing effect. Thus, consistent with the authors’ conclusions, the first step of my proposed model is the origins of a feminizing X chromosome (Figure 2A).
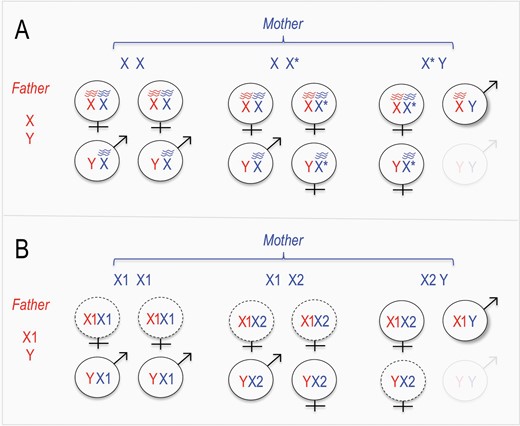
Outcomes of crosses under known and proposed feminizing X chromosome systems. (A) Under known feminizing X chromosome systems, the feminizing X chromosome (X*) causes feminization of X*Y individuals. In crosses between X*Y females and XY males, the inviability of YY offspring (faint) leads to 2/3 of surviving offspring inheriting the X* chromosome. (B) The sex chromosome system of L. mandarinus resembles known feminizing X chromosome systems, with the X2 and X1 chromosomes fulfilling the roles of feminizing and non-feminizing X chromosomes, respectively. In addition, dotted outlines represent classes of offspring that are less frequent than would be expected from Mendelian inheritance.
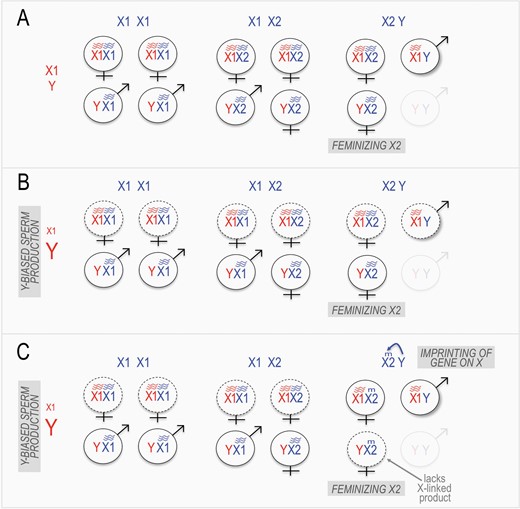
A 3-step model for the origins of the complex mandarin vole sex chromosome system. (A) The X2 chromosome arises as a “feminizing” X chromosome, due to the fact that inviability of YY offspring leads to a transmission advantage in X2Y x X1Y crosses. (B) In response to female-biased sex ratios due to the presence of X2 in the population, males evolve Y-biased sperm production, leading to underproduction of various offspring classes (dotted outlines). (C) Also in response to female-biased sex ratios, a Y-linked gene evolves the ability to impose maternal imprinting on an essential X-linked factor, leading to inviability of X2Y offspring, and thus shifting offspring production towards X1X2 and X1Y offspring. Wavy lines indicate gene expression of an essential X-linked product, “m” indicates silencing of the gene in offspring (“imprinting’, e.g., by methylation).
Step 2: Evolution of Y-Biased Spermatogenesis
The authors also reveal an intriguing and complicated pattern of transmission. Dotted lines highlight the general patterns in Figure 1B, while Figure 3 shows the specific numerical results. In the reported crosses, various pronounced skews are observed relative to the Mendelian expectations. Offspring are skewed towards X1Y offspring (and away from X1X1) in X1X1 × X1Y crosses, towards X1Y and X2Y offspring (and away from X1X1 and X1X2) in X1X2 × X1Y crosses, and towards X1X2 and X1Y (and away from X2Y and YY) in X2Y × X1Y crosses.
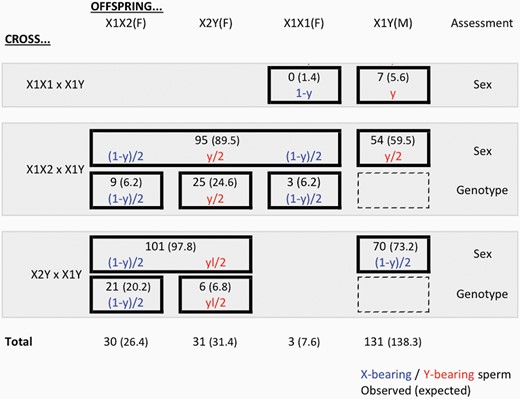
Results of crosses of L. mandarinus. Tests of either sex (male versus female) or genotyping of females were performed for 3 crosses in L. mandarinus. For each cross, the observed numbers are given, with the expected numbers assuming the maximum likelihood values for the fraction of Y-bearing sperm (y) and for the fraction of X2Y offspring of X2Y mothers that survive (l), as described in the text. Data are from Romanenko et al. (2020).
Curiously, the outcomes of crosses are as expected if X1- and Y-bearing sperm have very different successes, but that this difference in success is reversed in different crosses. For instance, in crosses between males (X1Y) and X1X1 females, offspring expected from fertilization by Y-bearing sperm are primarily observed: X1Y sons are observed but X1X1 daughters are not. The same holds in X1Y × X1X2 crosses: the 2 offspring classes expected from fertilization by Y-bearing sperm dominate among offspring, with X1Y and X2Y common but X1X1 and X1X2 rare. On the other hand, for the third cross, the opposite is true: in X1Y × X2Y crosses, it is the offspring classes produced by fertilization by X1-bearing sperm that dominate: X1X2 and X1Y are common, but X2Y and YY are rare or absent.
In the absence of additional functional and genetic data, it remains possible that the various deviations from Mendelian inheritance are due to many different factors—one factor explaining the lack of X1X1 progeny, another the lack of YY progeny, a third explaining the dearth of fertilizations of X1X2 mothers by X1 sperm, yet another explaining the dearth of fertilizations of X2Y mothers by Y-bearing sperm, and potentially others whose direct effects are not visible within the limited available data.
However, the data could also be explained by a simpler model. Notably, the offspring phenotypes and karyotypes of the X1X1 and X1X2 females (top 3 experiments in Figure 3) match well the expectations assuming a bias in sperm production where around 80% of sperm are Y-bearing (the maximum likelihood estimate is 79.8%; see Methods). For instance, a 79.8% bias would predict most of the non-Mendelian features of the data, including X1X1 mothers’ bias towards X1Y sons over X1X1 daughters (79.8% expected, 100% observed, P = 0.36 by 2-tailed binomial distribution test), X1X2 mothers’ bias towards X1Y sons over daughters (39.9% expected, 36.2% observed, P = 0.39), and X1X2 mother’s bias towards X2Y daughters over other daughters (66.4% predicted, 67.6% observed, P = 1). The dearth of X1X1 offspring for both X1X1 and X1X2 mothers is intriguing but not statistically significant (7.6 predicted, 3 observed; nominal P = 0.072 by simulations, see Methods and Figure 4).
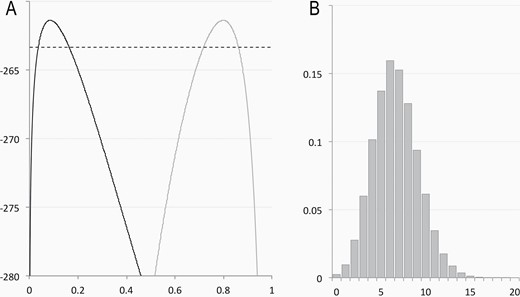
Estimates of parameters. (A) Log-likelihood values for various values of y (gray line) and l (black line), showing maximum likelihood values of 0.798 and 0.085, respectively, and cutoff for confidence intervals (dotted line) based on standard likelihood methods. (B) Results from 100 000 random simulations of number of X1X1 sons based on crosses shown in Figure 3, yielding a 2-tailed P-value of 0.072.
Thus, I propose that the second step in the evolution of the L. mandarinus system was the origins of a skew towards Y-biased sperm production (Figure 2B). Together with a feminizing activity of X2 (step 1, above), such Y-biased spermatogenesis could explain both the karyotype-genotype relationships of L. mandarinus and the overproduction of Y-bearing offspring of non-X2Y females. This leaves unexplained only the overproduction of X1-bearing offspring to X2Y females.
Step 3: Evolution of Maternal Imprinting of an Essential X-Linked Gene in X2Y Females
Such a combination of a feminizing X chromosome and Y-biased sperm production resembles the case observed in Akodon species and Dicrostonyx torquatus, in which available evidence suggests a feminizing sex chromosome along with Y-biased sperm production (Gileva 1987; Hoekstra and Hoekstra 2001). As noted above, for most females (X1X1 and X1X2 females), together constituting a predicted 80% of all females under equilibrium assuming equal fitness (Bull and Bulmer 1981), fertilization with Y-containing sperm produces a higher proportion of males, thus males that produce Y-biased sperm stand to benefit.
However, the case is very different from the third class of females, namely X2Y females. Because YY offspring perish, use of Y-bearing sperm by X2Y females produces only X2Y daughters–thus under Y-biased spermatogenesis, X2Y females produce both very high fractions of daughters (e.g., 89.9% = 79.8% X2Y plus 10.1% X1X2, assuming 79.8% Y-bearing sperm production), and granddaughters (88.8% = 79.8%/89.9% daughters are expected to be X2Y females, which themselves will produce mostly daughters). Thus the selection on X2Y females to skew offspring production is expected to be intense. The most direct solution may be found in Akodon species, in which XY females appear to produce Y-biased eggs, leading to skew towards male offspring (Hoekstra and Hoekstra 2001); however, it is possible that such a bias is unstable given selection on the feminizing X chromosome to counter the bias; indeed, the production of only X-bearing eggs in XY females in Myopus schisticolor might attest to the capacity of the X chromosome to manipulate oogenesis in XY females (Winking et al. 1981).
The observed preference for X1-bearing sperm by X2Y L. mandarinus females may be seen as an alternative mechanism for adaptation to overproduction of daughters. Because individuals of the rarer sex necessarily have higher mean fitness, female-biased sex ratios are expected to lead to a selective advantage for individuals that have more male descendents. By enriching for offspring fertilized by X-bearing sperm, X2Y females increase son production, and decrease production of granddaughters by decreasing production of largely granddaughter-producing X2Y daughters. However, if the ultimate causes of preference for X-bearing sperm are understandable, the mechanism by which this is achieved is more mysterious. Notably, whereas female mammals are known to achieve post-zygotic preference on the basis of sex (see the many references in Navara 2018), this is not the case here, as the pattern here follows presence of the X1 chromosome, not the sex (both X1X2 daughters and X1Y sons are more frequent than expected). Formally, an interesting possibility is pre- or post-zygotic decisions based on presence/absence of the X1 allele, although achievement of such a strong bias only in one genotype of female would seemingly require de novo evolution of the control of fertilization or of post-zygotic decision-making pathways by novel factors.
One more potentially mechanistically straightforward mechanism (but see below) would be maternal genomic imprinting leading to silencing of a (nearly) essential X-linked gene (i.e., one present on both X1 and X2 but not Y) during oogenesis of X2Y females. Under such imprinting, the essential X-linked gene would not be expressed from the maternally-inherited X in embryos, and thus viability of offspring from X2Y mothers would depend on inheritance of an unimprinted X1 chromosome through the sperm (Figure 2C). Crucially, such imprinting would need to occur only in X2Y females, since in other females it would increase, not decrease, daughter production. Restriction to X2Y females could be explained if the gene driving imprinting were Y-linked (or, less straightforwardly, if non-Y-linked genes could sense the presence of the Y chromosome). One limitation of this model is the apparently low rate of adaptative evolution on Y chromosomes (although potentially maintenance of newly Y-linked previously-autosomal genes would be expected to increase opportunities for adaptation.) (Note that while an even more straightforward mechanism could be loss of a X1-linked gene from the X2, such a loss would abolish the X2’s transmission advantage and is therefore unlikely to evolve).
Observation of occasional X2Y offspring could be explained by loss of imprinting of the gene, a phenomenon that is frequently seen during various stages of development (e.g., Lambertini et al. 2008), and which could be more common for newly-evolved cases, of imprinting or in cases of intragenomic conflict over imprinting (for instance, as in this case, since the X2 chromosome likely incurs a cost from being imprinted due to mortality of X2Y offspring). As for the case of estimating the degree of skew in spermatogenesis, the fraction of surviving X2 offspring, l, can be estimated from the data (see Methods). The maximum likelihood value of l, 0.085, provides a good fit to the data, with the observed offspring frequencies of X2Y females consistent for both the fraction of daughters (57.2% expected, 59.0% observed; P = 0.64) and for fraction of daughters with X2Y genotype (22.2% expected, 25.2% observed; P = 0.83) (Figure 3).
Thus, I propose that the third step in the process was the evolution of maternal imprinting of an X-linked gene important for viability, by a Y-linked factor in X2Y females.
Summary of the Model
In total, then, I propose that the observed phenotype-genotype correspondences and outcomes of crosses for L. mandarinus could be explained by a 3-step evolutionary history:
(i) Inviability of YY individuals drives the rise of a feminizing X chromosome. First, an X2 allele arises that causes feminization of XY individuals. Because of inviability of YY offspring, this allele is transmitted to 2/3 of offspring of XY females. Given this transmission advantage, this allele enjoys an initial advantage and rises in frequency. Its rise in frequency drives a female-skewed sex ratio, which leads to selection on all females to produce more sons.
(ii) Female-biased population sex ratio drives the rise of Y-biased sperm production. Second, an autosomal or Y-linked allele arises that causes Y-biased sperm production (~80% Y-containing sperm), leading to production of more sons. Because sons have higher average fitness under female-biased sex ratios, this allele enjoys an advantage and rises in frequency and fixes in the population. Notably, while Y-biased sperm production increases son production when the mother is X1X1 or X1X2, for X2Y females it increases the overall number of daughters due to increased X2Y daughter production. This circumstance leads to strong selection on X2Y females to produce more sons.
(iii) Female-biased population sex ratio and highly-female-biased offspring sex ratio of X2Y females drive the origins of a mechanism to kill X2Y offspring, perhaps through imprinting of an essential X-linked gene. Third, an allele (likely Y-linked but potentially autosomal) arises that causes imprinting of an essential X-linked gene during oogenesis of X2Y mothers, leading to the inviability of X2Y daughters. X2Y females carrying this allele produce a higher proportion of sons, thus the allele enjoys an advantage and rises in frequency and fixes in the population.
Alternative Models
Alternative models are imaginable, even if we constrain ourselves to models that proceed by a series of adaptive (rather than neutral) steps. Most clearly, it is possible that Y-biased spermatogenesis evolved first due to a selfish driving Y, producing male-biased sex ratios and thus selecting for a feminizing X (a possibility pointed out by an anonymous reviewer). However, the proposed order seems more likely insofar as feminizing X chromosomes have been observed in rodents in the absence of driving. Moreover, insofar as Y-biased spermatogenesis is more likely to evolve when the entire genome benefits (as in the case of rebalancing the sex ratio, as opposed to selfish Y drive), the proposed model is arguably more likely a priori.
Another alternative involves XY females’ bias towards production of offspring fertilized by X-bearing sperm. I have proposed an imprinting-based model, however, one important alternative is biased fertilization, with XY females’ eggs preferring X-bearing sperm. Such a model would avoid the one peculiarity of the imprinting model, namely the question of why the X2 chromosome does not evolve to escape imprinting (although this quandary could be resolved if an escape allele has not yet had time to evolve, or if there are ongoing and overlapping cycles of suppression and escape). The imprinting model has the advantage of relying on the pre-existing mechanism of genomic imprinting, whereas biased fertilization invokes a novel mechanism evolving that occurs only in XY females’ eggs. However, I acknowledge that my intuition on this point matches neither that of the original authors (Romanenko et al. 2020) nor that of 2 anonymous reviewers, and look forward to further empirical and theoretical work on the issue.
Future Directions
Both of the novel aspects of the proposed model—Y-biased sperm production and maternal imprinting of an X-linked gene—lend themselves to testing. The former could be tested through genotyping of sperm, while the latter could be probed by searching for X-linked genes that are specifically imprinted in X1X2 daughters of X2Y females. Whether or not this model proves accurate, similar modeling attempts of other systems are likely to be of importance in making progress on our understanding of complex sex chromosomal systems of rodents and other organisms. The important work of Romanenko and coauthors has greatly furthered our understanding of L. mandarinus sex chromosome biology. Further transmission and molecular genetic studies are urgent to reveal the form of, and forces shaping, this most remarkable of systems.
Methods
The likelihood of seeing the data given a value y for the fraction of Y-bearing sperm from X1Y males and a value l for the fraction of X2Y offspring of X2Y females that survive (due, for instance, to stochastic loss of imprinting), is given from the data in Figure 2 as:
Numerical evaluation for values of y and l from 0 to 1 shows that this value is maximized at y = 0.798 and l = 0.085 (Figure 4A).
The number of expected X1X1 offspring expected from the X1X1 × X1Y and X1X2 x X1Y crosses studied was simulated assuming the maximum likelihood values and the probabilities shown in Figure 3. Among 100 000 simulated sets, 7192 had a value as different or more different than the expectation (7.63) as does the real set (3), for a P value of 0.072 (Figure 4B).
Acknowledgments
I thank Polly Campbell and Noelle Anderson for helpful comments on early drafts of this work and 2 anonymous reviewers whose detailed suggestions on substance and presentation greatly improved the manuscript.
Conflict of Interest
The authors declare no conflict of interests.
References