-
PDF
- Split View
-
Views
-
Cite
Cite
Merle Stein, Florent Elefteriou, Björn Busse, Imke AK Fiedler, Ronald Young Kwon, Eric Farrell, Mubashir Ahmad, Anita Ignatius, Liam Grover, Liesbet Geris, Jan Tuckermann, Why Animal Experiments Are Still Indispensable in Bone Research: A Statement by the European Calcified Tissue Society, Journal of Bone and Mineral Research, Volume 38, Issue 8, 1 August 2023, Pages 1045–1061, https://doi.org/10.1002/jbmr.4868
- Share Icon Share
ABSTRACT
Major achievements in bone research have always relied on animal models and in vitro systems derived from patient and animal material. However, the use of animals in research has drawn intense ethical debate and the complete abolition of animal experimentation is demanded by fractions of the population. This phenomenon is enhanced by the reproducibility crisis in science and the advance of in vitro and in silico techniques. 3D culture, organ‐on‐a‐chip, and computer models have improved enormously over the last few years. Nevertheless, the overall complexity of bone tissue cross‐talk and the systemic and local regulation of bone physiology can often only be addressed in entire vertebrates. Powerful genetic methods such as conditional mutagenesis, lineage tracing, and modeling of the diseases enhanced the understanding of the entire skeletal system. In this review endorsed by the European Calcified Tissue Society (ECTS), a working group of investigators from Europe and the US provides an overview of the strengths and limitations of experimental animal models, including rodents, fish, and large animals, as well the potential and shortcomings of in vitro and in silico technologies in skeletal research. We propose that the proper combination of the right animal model for a specific hypothesis and state‐of‐the‐art in vitro and/or in silico technology is essential to solving remaining important questions in bone research. This is crucial for executing most efficiently the 3R principles to reduce, refine, and replace animal experimentation, for enhancing our knowledge of skeletal biology, and for the treatment of bone diseases that affect a large part of society. © 2023 The Authors. Journal of Bone and Mineral Research published by Wiley Periodicals LLC on behalf of American Society for Bone and Mineral Research (ASBMR).
Introduction
After 1.4 million European citizens have signed a petition entitled “Save Cruelty‐Free Cosmetics,”(1) the EU parliament will discuss and eventually decide whether to abandon all animal experiments in the European Union. Similarly, in the US, there is a growing public sentiment against animal experiments. In a Gallup poll, the moral acceptance of medical animal research dropped from 63% in 2002 to 52% in 2022.(2) Reflecting this sentiment, legislation approved in 2022 eliminated the previous requirement that new drugs need to be tested in animals to receive approval from the US Food and Drug Administration (FDA).(3)
In this context, local ethics committees decide about animal licenses with increasing sensitivity toward the trade‐off between ethics and scientific rationale. In the US, Institutional Animal Care and Use Committees (IACUC) are responsible for the oversight of animal care and use programs. IACUCs review, at least semiannually, institutions' experimental programs and inspect facilities to report welfare concerns, make recommendations on aspects of care, housing, or personnel training, and are authorized to suspend activities involving animals. Importantly, IACUC, as well as most grant agencies, require justification of animal numbers to be supported by statistical power analyses.
Progress in in vitro cell‐based systems as well as in silico models is used as an argument to suggest that all animal experiments have become dispensable. In this light, it appears necessary for the skeletal research community to assess and defend the general reasons for animal experiments in our field, in particular when systemic diseases are investigated that still cannot be entirely modeled in vitro or in silico.
This review aims to provide a balanced view of the benefits and pitfalls of various in vitro and in vivo models and to increase awareness of their suitability to specific research questions. In addition, it should equip scientists for a discussion on the topic with laymen, stakeholders, and decision‐makers with the final goal to convince them that animal experiments in the field of musculoskeletal research are still necessary, highly regulated and supervised by competent institutional bodies, and used only if in vitro and in silico models are not mimicking the systemic bone physiology.
The Current Landscape of Animal Experiments Used to Investigate Skeletal Diseases
Skeletal research focuses on understanding bone development, growth, remodeling, and aging to discover new treatments for numerous skeletal conditions including osteoporosis, arthritis, low back pain, genetic diseases, and bone tumors/metastasis that significantly impact public health systems. Their prevalence is anticipated to rise because of the increasingly aging population. Disability‐adjusted life years and global deaths from low bone mineral density–related fractures have increased significantly in the last decade: 121.07% (4,436,789–9,808,464 cases) and 148.65% (121,248–301,482 cases), respectively, with the highest disease burden in India, China, US, Japan, and Germany.(4)
Systemic bone diseases
Bone is crucial for systemic regulation of calcium and phosphate homeostasis and serves as an endocrine organ. Understanding bone pathophysiology in a systemic context is therefore essential for improving patient treatment options.
Metabolic bone diseases
Metabolic bone diseases can have genetic, hormonal, and/or environmental causes (eg, malnutrition) and include, among others, osteomalacia (rickets) and hyperparathyroidism. These conditions cause weakened bone, frequent fractures, and possible growth retardation in children and are often investigated in transgenic rodents.(5) Using such models, it was shown that the knockout of some genes that were expected to result in dramatic bone phenotypes was more subtle than expected (eg, estrogen receptor alpha(6)), whereas other molecules involved in bone metabolism like IL‐5 were only first identified by revealing their skeletal phenotype in transgenic mouse models.(7) Animal models have, furthermore, been used to study the potential effects of certain nutritional interventions, including the effects of vitamin D and calcium supplementation on bone health, influencing the development of dietary guidelines for preventing osteoporosis.(8‐10)
Osteoporosis
Osteoporosis, another metabolic bone disease affecting millions of people worldwide, is characterized by bone loss, leading to an increased risk of fractures. For osteoporosis research, genetically modified and/or ovariectomized or glucocorticoid treated rodents—and more recently zebrafish(11)—are used to study underlying mechanisms of menopausal or glucocorticoid‐induced bone loss, respectively, and to test the efficacy of new treatments, such as bisphosphonates and selective estrogen receptor modulators (SERMs).(12‐15) In more translational settings, large animals, mostly sheep,(16) and, rarely, non‐human primate models are used.
Rare genetic bone diseases
Rare genetic bone diseases affecting the entire skeleton like osteogenesis imperfecta, skeletal dysplasias, and osteopetrosis are major concerns, as they manifest in early childhood and can be life‐threatening. The research of genetic diseases can benefit from genetically modified cell lines, which can be used to elucidate pathways and help to screen for potential treatment. Ultimately, treatment effects and the success of potential drugs must be investigated in vivo in vertebrate models to verify their mechanisms of action.(17) For historical reasons, these models have mostly been mice, but with the availability of CRISPR/Cas9, options have increased dramatically.(18) In this context, zebrafish offer a valuable alternative to mice because of their ease of manipulation, transparent extra‐uterine development, and suitability for high‐throughput drug screening even at embryonic stages.(19)
Local diseases
Local diseases such as bone tumors (eg, giant cell tumors) perhaps offer the best starting point for replacement methods. Here, in vitro methods have tremendous potential in shedding light on the cells and signaling involved and identifying possible treatments.(20) Furthermore, these diseases are suited to test the limits of in silico models or organ‐on‐a‐chip.(21,22) Final validation, however, is still dependent on a vertebrate model.
Bone repair and regeneration
Bone repair and regeneration may be studied in a clinical setting or with an interest in basic bone biology/physiology. Fin bone injury or amputation models of zebrafish have been important in determining factors involved in osteogenesis and bone regeneration.(23,24) Fracture models at specific anatomical locations and with different surgical interventions are routinely done in rodents. In the clinical setting, more emphasis is placed on mechanical loading, thus requiring large animals.(25) These models are less frequently used but are essential in translational orthopedic settings.
For numerous reasons, about 90% of drug candidates, also those with promising results in vivo, never make it to the market,(26) but differences between animal and human physiology are a minor issue in most cases. Certainly, all in vivo and in vitro models have limitations but are in combination useful to analyze and understand particular aspects of human diseases (Fig. 1). It is noteworthy that not only in vitro but also in vivo models have been drastically refined over the last decades and years to more accurately reflect human diseases and to ensure humane animal treatment. Animal models often allow a comprehensive analysis of bone pathophysiology; thereby, they provide the basis for functional interference with essential disease factors, which can lead to the description of druggable targets and the subsequent development of novel therapeutic strategies. The following paragraphs provide an overview of the different currently used models, their advantages, and caveats (Table 1).
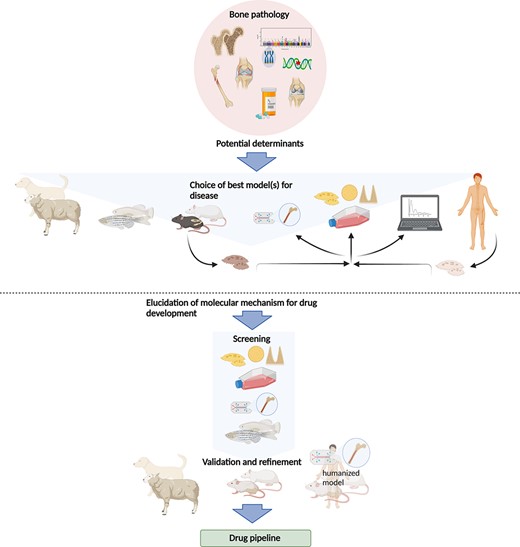
The current use of in vitro and in vivo models in preclinical research of bone pathologies. The etiology of a given bone pathology as well as the hypothesis investigated suggest one or more suitable model(s) to study the question. These may be sheep, teleost fish, rodents, or cells (from animals or human patients). Cells may be analyzed directly or grown in 2D or 3D cultures or “on a chip.” Data gained from these experiments can be used for in silico models. The combination of different models should allow elucidation of molecular mechanisms that can then be exploited for therapeutic strategies, where the different models can be used as readout systems. For large screens to elucidate molecular mechanisms of bone diseases, cell culture and organ‐on‐the‐chip models as well as small fish are better suitable. Refinement and validation of screening results then have to be carried out in larger animal models such as rodents or larger mammals or humanized systems. This allows the application of these assays for drug testing to discover novel products to treat common and rare bone diseases.
Model | Advantages | Limitations |
In vitro cell culture (2D, 3D, organ‐on‐a‐chip) |
|
|
In silico (data‐driven and knowledge‐driven modeling) |
|
|
Fish models |
|
|
Murine models |
|
|
Ectopic models |
|
|
Large animal models (sheep) |
|
|
Model | Advantages | Limitations |
In vitro cell culture (2D, 3D, organ‐on‐a‐chip) |
|
|
In silico (data‐driven and knowledge‐driven modeling) |
|
|
Fish models |
|
|
Murine models |
|
|
Ectopic models |
|
|
Large animal models (sheep) |
|
|
Model | Advantages | Limitations |
In vitro cell culture (2D, 3D, organ‐on‐a‐chip) |
|
|
In silico (data‐driven and knowledge‐driven modeling) |
|
|
Fish models |
|
|
Murine models |
|
|
Ectopic models |
|
|
Large animal models (sheep) |
|
|
Model | Advantages | Limitations |
In vitro cell culture (2D, 3D, organ‐on‐a‐chip) |
|
|
In silico (data‐driven and knowledge‐driven modeling) |
|
|
Fish models |
|
|
Murine models |
|
|
Ectopic models |
|
|
Large animal models (sheep) |
|
|
In Vitro Models for Skeletal Research
2D Cell culture models
Two‐dimensional (2D) tissue culture models have dominated bone research for half a century. Calvarial pre‐osteoblast cells, stromal mesenchymal cells from bone marrow, and outgrowths from human bone specimens can be utilized to recapitulate osteoblast differentiation.(27‐31) Osteoclasts can be derived from peripheral blood monocytes or bone marrow of rodents, birds (mainly chickens), and rabbits to allow observation of their development.(32‐38) Osteocytes are a challenging bone cell type in culture, but particularly the development of cell lines has allowed for the investigation of some osteocyte aspects in vitro (for a review of in vitro and in vivo osteocyte models, see Kalajzic and colleagues(39)). Induced pluripotent stem cells (iPSCs) have recently become another prospect for bone regeneration and disease modeling.(40)
A wide variety of in vitro screening approaches have been employed to identify potential regulators of bone cell differentiation.(41‐45) For example, high‐throughput siRNA(41,42) and compound screens have identified small molecules such as roscovitine, rapamycin, and FK506, which augment osteoblast differentiation in vitro.(43) These approaches allow us to reduce animal experimentation by testing potential drugs at a cellular level. As such, only successful drug targets might be exploited in animal models, limiting and specifying the use of animals. Despite successes in identifying anabolic and catabolic factors, strong limitations are obvious. The readout of early differentiation markers such as alkaline phosphatase, for example, is not always predictive for the entire process of bone formation.
One of the major limitations of 2D cell culture is the poor equivalence of this environment to the conditions within the bone.(46) There are numerous attempts now to combine multiple cell types in vitro and to increase the levels of complexity. For example, several research groups include hypertrophy and mineralization regimes in their in vitro cultures,(47,48) as well as altering pH and oxygen tension.(49,50) The highly mineralized nature of bony tissue, in addition to anatomic structure, means that plastic or other planar surfaces are a poor environmental proxy. Importantly, these cultures are unable to maintain the phenotype of osteocytes and osteoclasts for more than several weeks. As a consequence, much work has focused on the optimal culture of osteoprogenitors and osteoblasts on these surfaces. However, recruitment and control of osteoblasts in the human body also rely on the presence of other cell types, with, for example, EphA4(51) from osteoclasts stimulating osteoblast formation. Osteocytes are by far the most abundant cells in bone and orchestrate osteoblast and osteoclast function, yet they are missing in most 2D cultures. Another major drawback comprises the altered behavior and differentiation capacity of isolated primary cells in culture (eg, calvarial cells differentiating into adipocytes).
Bone Organ Culture
To overcome the aforementioned limitations, bone organ cultures are used, where harvested bone tissue is placed in a nutrient‐rich medium, allowing it to remain viable and functional for several days to weeks.(52‐54) These cultures were used to study processes including bone growth, remodeling, and repair.(52,55,56) Here bone cell interaction can be studied in the absence of confounding factors present in vivo.(52) Furthermore, treatment of the organ culture with substances instead of the whole organism increases animal welfare. However, the tissue may change in response to the stress of being removed from the organism. Some limitations can be overcome by complex co‐culture models, which include multiple cell types to mimic, among others, the remodeling of the tissue,(57) immune responses to the differentiation processes,(58) vascularization, and even the formation of a bone marrow niche.(59,60) Nevertheless, the use of isolated bone tissue may not capture the complex interaction between different cell types and tissues in vivo.
3D Cell Culture Models
Further developments extend to three dimensions using scaffold structures. But even in “3D” foams(61) the cells do not receive mechanical cues from all directions. To receive a continuous mechanical 3D stimulus, for example, a fibrin matrix, attached to a sparingly soluble calcium phosphate bracket has been used.(62) Here embedded osteoprogenitor cells differentiated and maintained an osteocyte phenotype for over a year and even produced a lacuna‐canalicular network. Similar results can be achieved with fibroin.(63) These principles have allowed the development of tricultures that maintain the phenotype of osteoblasts, osteoclasts, and osteocytes and have successfully been used to explore the influence of unloading on the bone resorption process.(64) This system has also allowed new insights into osteoid deposition and mineralization and may enable researchers to focus on individual variables of bone remodeling, which is not feasible in vivo. Such models may be harnessed in screens with more variables and repetitions than would be ethically appropriate in vivo. Biological processes are also more easily controlled and monitored in these cultures than in vivo. Although it is accepted that these systems still require significant development to introduce an immune and neuronal component, vasculature, and surrounding tissue to properly replicate the in vivo environment, it is clear that the combined use of modern 3D cultures with in vivo models will serve to reduce animal use and help refine both in vitro and in vivo models.
Organ‐on‐a‐chip (multichannel 3D microfluidic cell culture platforms that simulate the mechanics, activities, and physiological response of an entire organ), while in their infancy in the bone field, will soon very likely advance in vitro bone models, making them more attractive for basic and preclinical research.(21,22)
Perspectives of in vitro models
Although it will be several years before some of these technologies and intricate in vitro models will be sufficiently developed to replace in vivo models, some are already appropriate for more simplified experiments, such as drug toxicity screening. For characterization and discoveries of new structures in bone in vivo (eg, innervation, blood, and lymphatic vessels), in vitro models are not suitable because they rely on what is known from the in vivo situation. However, researchers are already performing more experiments in vitro and in silico before progressing into in vivo models. This trend will only continue to grow but may never entirely replace the intricacies of animals because of to the reasons mentioned above.
In Silico Models for Skeletal Research: State of the Art
Over the last decades, in silico computer models and simulations (referring to “silicon,” the main component of computer chips) have increasingly emerged in the biomedical sciences. Bone research and disease management frequently use in silico tools, although they might not always be perceived as such. An example is MRI images, where a physics‐based model is required to transform the raw data into interpretable images. Different in silico models allow virtual testing of biological hypotheses. Data derived from these models is quantitative and, unlike many biological experiments, not limited to single endpoints. This data can provide insight into underlying mechanisms with an accuracy not possible in biological experiments and can describe outcomes and numerical values previously unknown.(65)
A plethora of bioinformatics and machine learning (ML) tools are now available for processing the enormous amount of data generated by omics and sequencing initiatives (eg, genomewide association studies [GWAS]; see also Box 1). These data‐driven models allow insight into basic biology as well as clinical variation. One example is the development of the skeletal cell atlas(66) bringing together all available murine single‐cell RNA sequencing data sets into a comprehensive framework, making use of open‐source bioinformatics codes. This atlas provides a physiological blueprint of the limb development process and a platform to investigate in silico, for example, the effects of genetic mutations and knockouts. Using Boolean modeling, additive modeling, or other systems biology technologies allows us to turn these blueprints into actionable gene regulatory models. These models can then be used to perform extensive in silico screening experiments to identify optimal culture conditions (medium composition) or possible (combinations of) druggable targets in skeletal diseases.(67) Eventually, these targets will then have to be tested in vitro and/or in vivo. Agent‐based models that simulate actions and interactions of autonomous agents, such as individual molecules or bone cells, have been used to investigate different drivers for limb bud outgrowth, including the combination of differential cell adhesion and elasticity to determine limb bud shape(68) or filopodial tension to explain the convergent extension of limb bud tissue.(69) These models may be utilized to predict certain outcomes of knockout animal experiments and might thereby restrict the generation of knockout experiments to those that are likely to exhibit a clear function of a gene product in an in vivo environment.
Genomewide association studies (GWAS) involve scanning genetic markers across the whole genomes of individuals within large cohorts to identify genetic variants associated with disease‐related traits. Over the past decade, GWAS have identified more than 500 genomic loci harboring genetic variants associated with bone mineral density (BMD), as well as eBMD, a correlate of BMD measured via ultrasound.(94‐96) The majority of BMD‐associated variants are non‐coding. Most likely some or even most causal variants reside in cis‐regulatory elements that alter the expression of protein‐coding genes in their proximity. Importantly, genes at BMD‐associated loci include key members of pathways targeted by osteoporosis therapeutics, suggesting that other actionable, unidentified drug targets reside at other loci. Because the biological mechanisms underlying BMD associations may involve developmental, mechanical, autocrine, and paracrine factors that are difficult to model in vitro, gene discovery in animal models is a critical approach to identify causal genes underlying GWAS variants and therefore for the translation of GWAS findings into clinical targets.
Tissue‐level models describing bone (patho)physiology using partial differential equations are currently the most prevalent type of modeling in the bone field with applications ranging from limb development over fracture healing to bone remodeling. An example of a successful model predicted neotissue growth in 3D‐printed scaffolds.(70) This model was developed starting from the curvature‐based growth principle and subsequently confirmed by in vitro experiments. It was then used to optimize the structure of a 3D‐printed scaffold for maxillofacial applications and tested in an in vivo rat model, confirming the superiority of the in silico‐designed scaffold over the clinical golden standard.(71)
At the level of the whole organism, gait models are a standard tool to assess mechanical loading in muscles, joints, and bones in animal experiments as well as clinical studies.(72) Finally, in silico clinical trials can be used to plan, augment, or replace physical trials. As an example, testing new drugs for osteoporosis using fractures as primary clinical endpoints requires 1000 patients followed up over many years. The biomechanical computed tomography (BTC) provides a surrogate endpoint calculated using quantitative CT images and continuum mechanics.(73,74) The BTC is a good predictor of the patient's bone biomechanical strength (and hence fracture risk), and its use, once approved by regulators, will allow for smaller cohort sizes.
In Vivo Models for Skeletal Research: State of the Art
Teleost fish models
Preclinical bone research is carried out in in vivo models. In contrast to established rodent models in skeletal research, small‐sized laboratory teleost models (ie, bony fish models) have only emerged over the last few decades. This was driven by sequencing of the zebrafish genome, which allowed for a greater understanding of its similarity to that of humans and other vertebrates.(75) Because of evolutionarily conserved genetic, developmental, and compositional similarities between bones of teleosts and mammals, not only zebrafish(19,76‐79) but also medaka are used to model skeletal diseases and test drug targets.(11,19,76‐81) The diversity of laboratory teleost species offers additional advantages in the scope of both intervention studies and studies of aging‐related bone degeneration. Killifish with a life span of up to 6 months, for example, might reduce time‐intensive experiments in longer‐lived species.(82,83) Moreover, because of the transparency of teleosts up to early larval stages, they are commonly used in concert with transgenic modifications for direct visualization of the effects of genetic or chemical perturbations on osteoblast and osteoclast differentiation, skeletal morphogenesis, and mineralization.(84‐86) Because of the small size even in adulthood, teleosts are amenable to high‐resolution, whole‐body imaging, which enables deep phenotyping at a large number of skeletal sites.(87)
Despite teleost fish being suitable organisms to study many aspects of bone development, matrix mineralization, modeling, and even the effect of microgravity,(88) there are some important differences to humans, highlighting their limitations (Table 1). The bone matrix of some species, for example, medaka and killifish, is anosteocytic, limiting their use for modeling diseases affecting the osteocyte‐lacunar network. Moreover, the mammalian bone remodeling process is not directly comparable to small‐sized teleosts, which have a different bone microarchitecture (eg, lack of an osteonal cortex and extensive trabecular compartments). Given the aquatic environment of fish, further species‐related differences in terms of anatomy, mineral uptake, and musculoskeletal loading apply. In general, biological mechanisms discovered in zebrafish should also be examined in mammalian models to assess their translational potential.
Genetic zebrafish models
As an experimental system, zebrafish are positioned between in vitro and in vivo models, combining the ability to perform experimental manipulations traditionally confined to cell culture, with the complex physiology of an intact organism. Because of their small size, low cost, and ease of genetic manipulation, zebrafish are well suited for forward and reverse genetic screens. For zebrafish, there is a powerful toolbox including CRISPR‐based gene editing,(89,90) the broad availability of mutants,(91) and resource centers such as the Zebrafish International Resource Center (ZIRC) and European Zebrafish Resource Center (EZRC). The pursuit of these reverse genetic screens could augment and advance ongoing systematic knockout mouse phenotyping projects, such as the International Mouse Phenotyping Consortium (IMPC)(79,92,93) and ancillary bone phenotyping projects,(94‐96) for example, by prioritizing screened genes and enabling more effective use of resources.(79) Zebrafish screens could significantly aid follow‐up functional studies to GWAS or sequencing studies by allowing the generation of mutant phenotype data specifically for genes at loci harboring trait‐associated variants.
Models for several human genes whose mutations are linked to rare and complex skeletal genetic conditions have been established in zebrafish, for example, Plod2 (Bruck syndrome),(97) col11a2 (early‐onset osteoarthritis),(98) col1a1a, col1a1b, and col1a2 (osteogenesis imperfecta),(99) lrp5 mutants (osteoporosis‐pseudoglioma syndrome),(100,101) enpp1 (ectopic mineralization),(102) and kif6 mutants for modeling spinal curvature disorders.(103) These and numerous additional models have highlighted the value of teleost models for elucidating the pathophysiology of genetic skeletal diseases and mineralization defects.(19,104,105) Zebrafish mutants have evolved as particularly powerful tools for screening the effects of drugs and osteoactive compounds on the skeleton.(11,106,107) Molecules may be administered through the aquatic environment without the need for injections. For instance, submerging embryos of the Chihuahua zebrafish model of osteogenesis imperfecta in water containing 4‐phenylbutyric acid (4‐PBA) resulted in ameliorated bone mineralization in larvae, and long‐term treatment reduced skeletal deformities.(108) Such in vivo approaches in zebrafish allow efficient and direct assessment of systemic effects and are helpful to accelerate the search for treatment options.
The need for zebrafish to support human genetic research will grow with continuing advances in whole‐genome sequencing (WGS) and associated genetic studies, which discover candidate pathogenic variants at rates exceeding our ability to analyze their functions.
Rodent models
More than any other in vivo model, the use of rats and mice is a critical pillar of skeletal research and drug development. It was indeed the advent of reverse genetics in rodents that allowed researchers to decipher important factors for bone growth and remodeling in a systemic context.(109) One prominent example is the discovery of receptor activator of NF‐κB ligand (RANKL) during osteoclastogenesis: Pivotal studies in genetically engineered mice with altered expression of the RANKL/osteoprotegerin (OPG) system demonstrated its influence on osteoclast formation and bone physiology.(110‐112) Based on these fundamental discoveries, the antibody denosumab against RANKL was developed as a rational treatment for osteoporosis.(113,114) Another success story includes the development of romosozumab (anti‐sclerostin).(115‐120) Human GWAS candidates like Wnt16(121‐124) and RSPO3(125,126) involved in bone density have also been validated in mouse models.
For 441 known genes where mutations cause human genetic skeletal disorders, at least 260 mouse models have been phenotyped (coverage of 59%).(127) Additionally, there are standard models for almost all bone diseases in rodents and other vertebrates, such as postmenopausal and age‐related osteoporosis, diabetes‐induced bone loss, inflammatory arthritis, and fracture healing. All of these resemble the human diseases in characteristic aspects.
In skeletal research, rats have been a preferred model for postmenopausal osteoporosis induced by ovariectomy/orchiectomy, steroid‐induced bone loss, dietary interventions (eg, low calcium), immobilization (by surgery or tail suspension),(128) jaw osteonecrosis,(129) and fracture healing.(130) Molecular factors involved in human bone (re)modeling are generally well conserved in rodents, so most genetic skeletal diseases can be recapitulated in mice.(127) Mice models, however, have limitations as growth plates do not necessarily close as during skeletal maturation in humans. Further, they do not show natural menopause, and ovariectomy might not reflect this entirely, which resembles an acute loss of estrogen in contrast to the slower decline of estrogen in natural menopause. The rodent cortical bone also does not harbor Harversian channels, and their quadruped gait leads to different loading of the axial skeleton. With increasing evidence of links between whole‐body metabolism and skeletal biology,(131) it is also necessary to keep in mind that mice have a faster metabolism and are often not raised under thermoneutrality.
Genetic mouse models
The emergence of reverse mouse genetics and the possibility of global gene inactivation in “knockout” mice (KO) or the introduction of specific mutations in “knock‐in” (KI) mice have contributed immensely to our knowledge of bone formation and treatment of monogenic skeletal diseases. Successful examples include the various forms of chondrodysplasia (for which antagonists of activated FGFR3 or downstream pathways are now clinically tested), hypophosphatemia (now successfully treated with a recombinant form of alkaline phosphatase), fibrodysplasia ossificans, or osteogenesis imperfecta.(132‐134) In the most recent IMPC data release (17.0), bone mineral density data in knockout mice are available for about one‐quarter of the protein‐coding genes (7141 of ~25,000–30,000). After characterization at the basal level, the skeletal phenotype of transgenic rodents may be combined with additional challenges such as ovariectomy (OVX) or systemic glucocorticoid (GC) treatment to mimic postmenopausal or glucocorticoid‐induced osteoporosis.
Although global KO mice are still an important tool in skeletal research, their usefulness may be limited by embryonic lethality or by the inherent limitation of phenotypes caused by embryonic global gene inactivation. The latter hinders the interpretation of the phenotype at postnatal adult stages by having to tease apart the primary and secondary effects of gene modification. These drawbacks can be avoided by a conditional gene knockout approach (cKO). Based on the Cre‐LoxP system of gene recombination, this approach allows deletion or expression of a gene in a temporospatial manner(135) (Box 2). The remaining limitations stem from the irreversible nature of the gene ablation and the incomplete knowledge of which cells exactly express the Cre‐recombinase under selected promoters.(136,137) The increasing number, availability, and lack of characterization of Cre lines have raised concern about their authentication, but solutions to this concern are being addressed.(138) Efficient breeding strategies can limit the number of mice. To limit animal numbers, scientists commonly collect multiple tissues, and power analyses are used to calculate necessary animal numbers for the most efficient outcome.
The Cre recombinase was first discovered in the bacteria P1 phage. Since introducing it into transgenic modified mice in the 1990s, it has become an extremely powerful tool to allow conditional loss‐ and gain‐of‐function studies, including lineage tracing. This requires a “Cre” mouse line in which the Cre‐recombinase is expressed under the control of a chosen promoter active in a relatively specific population of cells or tissues. This enzyme, once expressed and active, can recognize bacteriophage‐derived “LoxP” sequences inserted into the genome of a second mouse line, generally in the 5′ region of a gene to be inactivated, cut the sequence in between, and join the two generated DNA ends to effectively delete the sequence between two LoxP sites. Crossing these two mouse lines leads to mice where gene inactivation occurs in all cells where the Cre‐recombinase is expressed and active (and their progeny as this genomic event is not reversible). Some “Cre” lines express modified forms of the Cre‐recombinase that are inactive but can be activated after injection of an inducer, thereby rendering the system inducible and allowing spatiotemporal control of gene inactivation. In the most widely used tamoxifen‐induced system (CreER[T2]) the Cre‐recombinase is fused to a mutated hormone‐binding domain of the estrogen receptor and can be activated by binding to tamoxifen, thus allowing translocation of the cytoplasmic Cre into the nucleus and recombination. In another approach, doxycyclin (Dox) can be used in transgenic tTA (Tet off) mouse models to prevent Cre transcription, for example, in unborn mice by treating pregnant females. (Repeated) withdrawal then leads to a (or several) defined time window(s) of gene deletion. Less frequently, a reverse tTA (rtTA) is used for a Tet‐on approach that activates recombination upon Dox treatment.
This Cre‐loxP strategy also allows, thanks to the use of the Rosa26 LoxP‐STOP‐LoxP allele, the expression of normal or mutated or fluorescent genes in a specific tissue or cell lineage and an inducible manner. This way, cell tracing can be performed, thus allowing one to follow the fate of specific cells during development, aging, or diseases, or to assess the functional consequence of mutated genes in specific cell populations. This system can also be used to ablate specific cell populations by controlling the expression of “suicide” genes in selected cell populations.
Through the deletion of specific genes in restricted cell lineages, cKO mouse models can provide important information about interactions within and between bone cells and other tissues. cKO mice models combined with lineage tracing were pivotal in discovering the fate of skeletal stem cells, their diversity,(139,140) and their importance in bone healing.(141) Mouse models also enabled the discovery of a distinct type of blood vessel(142) and, more recently, lymphatic vessels important for regeneration after bone injury.(143)
Ectopic bone models
Human cell transplantations are often performed on matrix structures to develop ectopic sites for bone. Rodents, in particular mice, are employed to model bone formation and bone physiology with human cells while avoiding tissue culture artifacts (eg, the absence of vasculature and innervations). These humanized structures can be used for drug testing but also for basic research questions because they recapitulate all stages of bone development or regeneration (Box 3). Furthermore, ectopic bone formation offers an approach to investigate the osteogenic potential of molecules or biomaterials needed to assist in bone healing or augmentation.
Scientists employ ectopic models to assess several aspects of bone formation and repair processes, especially when assessing new approaches/materials/small molecules in the tissue engineering and regenerative medicine (TERM) field. There are instances where it can be desirable to use an ectopic location to test the osteoinductive properties of a drug/small molecule or a biomaterial.(193) Given the correct stimuli, it is possible to recapitulate almost all aspects of bone formation in an ectopic location. The most commonly used locations include the kidney capsule, intramuscular locations, and subcutaneous implantation. The benefits of the placement of a construct/drug under the fibrous outer kidney capsule where it undergoes spontaneous bone formation are the high level of vascularization, lack of endogenous bone‐forming cells (which can be useful when assessing the biology of a specific cell type or osteoinductive factor), and some level of the mechanical load caused by compression of the fibrous capsule. However, the model is technically challenging and invasive and is not commonly used.(194,195)
Intramuscular implantation of cells, biomaterials, and various growth factors/small molecules are often used as an ectopic model of bone formation. Although this model does not involve the creation of a defect, it is still relatively invasive given the requirement for some level of blunt dissection to implant a construct and/or the space required within the muscle for new bone to form. One advantage of this model compared with other ectopic models is the presence of a source of cells that have the osteogenic capacity, the satellite cells. These cells can, for example, undergo osteogenesis in the presence of bone morphogenetic proteins (BMPs).(196,197) By far the most commonly used model of ectopic bone formation is the subcutaneous implantation of various constructs to assess bone formation. In this scenario, all manner of biomaterial, in combination with various cell types and small molecules, can be assessed for their ability to induce bone formation in the absence of surrounding bone‐forming cells or a bony environment.(198‐200) This can be very useful to remove any confounding effects of endogenous factors or cells on the experimental outcome. The model has further advantages of being minimally invasive and simple to perform. Although these models do not contain the relevant functional mechanical loading component, it may be argued that neither do many long bone defects because of the need for fixation/stabilization of the defect for reasons of animal welfare and to allow healing to occur. Subcutaneous bone models are also being used in the field of oncology, where researchers are actively developing new models of bone metastasis using humanized models of bone formation to create more clinically relevant models.(201‐203)
Others have shown a role in the process of endochondral ossification in atherosclerosis in mice.(204) What all of these models have in common is that the host animal provides a vascular supply and all of the requisite cells required for bone formation and remodeling to take place. This cannot occur in in vitro systems.
Finally, to bring the bone environment to the ectopic models, Andres Sastre and colleagues have developed a “semi‐orthotopic” bone defect model that incorporates a viable bovine bone plug in a subcutaneous pocket of a nude mouse.(205) This allows for the creation of several bony defects within this implanted bone plug in one animal without the need to perform invasive surgery on the animal, thereby having several defects an order of magnitude larger in volume than could be created in a mouse femur. Whether this approach might be used to replace some of the existing experiments that would be performed in orthotopic and ectopic settings remains to be seen. In general, a lot of skeletal research is based on previous cultivation and characterization of bone cells; these may stem from cell lines or primary human cells differentiated or cultured ex vivo. The models described above can be applied in a huge range of manners that address questions surrounding bone development and healing as well as the formation of the marrow niche. They are still necessary and are now being recognized as very relevant for multiple models of cancer metastasis and leukemia and also have great utility in understanding diseases of heterotopic ossification. Subcutaneous models have the advantage of being “ectopic” and therefore are not influenced by a surrounding bony environment. This allows the testing of the true osteogenic potential of a cell, small molecule, or biomaterial without interference from the surrounding tissue. By contrast, the inclusion of a bony environment in a subcutaneous pocket brings the advantage of being able to interrogate crucial steps of bone healing, such as integration and remodeling, which is not possible in most subcutaneous models.
For implant testing, the osteoinductive properties of materials such as calcium phosphate ceramic(206) or β‐tricalcium phosphate scaffolds(207) can also be tested ectopically in large animals, for example, in sheep muscle. Also, auto‐transplanted mesenchymal stem cells were able to induce bone formation in a ceramic bone substitute in sheep without the additional need for BMPs.(208)
Large animal models
Larger animals, such as rabbits, dogs, pigs, small ruminants (sheep, goats), and non‐human primates, are less frequently used in skeletal research.(130,144‐146) The main reasons are ethical considerations. High costs, the limited obtainability of skeletally mature or aged animals, and the time‐consuming breeding and experimental effort also restrict the use of large models. Furthermore, the evaluation of molecular mechanisms is restricted because of limited transgenic organisms and specific analytical tools (eg, antibodies). Also, the application of experimental drugs in large animals can be expensive because of their body weight. Despite these disadvantages for basic molecular research, large animals are of utmost importance in translational settings because they most closely mimic the human situation in terms of bone dimensions, structure, and turnover. Therefore, they are particularly important to advance surgical techniques, orthopedic implants, fracture fixation devices, novel biomaterials (eg, bone grafts), and tissue engineering approaches and to investigate fracture healing.(16,130,144,146) A further advantage of large animal models is that the impact of mechanical loading can be considered, which is not possible in mice or rats but important for bone remodeling, the osseointegration of implants, and degradation of biomaterials.(147)
After mostly abandoning dog models,(25,148) sheep have proven particularly appropriate for skeletal research because bone dimensions are similar to humans and the ease of husbandry.(16,25,149‐152) However, their bone microstructure differs in some aspects.(148,153,154) The cortical bone of young sheep is plexiform because of their fast body growth. With age, secondary Haversian bone becomes more prevalent.(148,150) Importantly, compared with humans, sheep have a higher bone mineral density and mechanical strength,(152,155) which must be taken into account in experimental settings, especially for implant testing or induction of osteoporosis. However, the bone formation rate of sheep (1.2–1.5 mm/d) is similar to that of humans (1.0–1.5 mm/d)(148,149) as is the healing rate of bone fractures.(148,156) Also, common human bone turnover markers, including alkaline phosphatase, osteocalcin, and tartrate‐resistant acid phosphatase, are useful to monitor bone status in sheep.(157)
Sheep are often used to test anti‐osteoporotic drugs, treatments for fractures, and the efficacy of implant fixation.(149,151,152,158) OVX models only lead to transitional and moderate bone loss(152,159‐161) and were therefore combined with a calcium and vitamin D‐restricted diet(162,163) and with additional GC treatment.(152,161,162) GC application in sheep leads to considerable bone loss, structural deterioration, and biomechanical impairment comparable with GC‐induced osteoporosis in humans and has therefore been most widely used.(16,152,161,162) However, GC therapy can provoke major side effects depending on the treatment regime, such as susceptibility to infections and discomfort, raising ethical concerns.(164)
One disadvantage of sheep as an osteoporosis model is that there are seasonal changes in bone metabolism.(165) Another drawback is the different gastrointestinal system, which impedes the oral administration of drugs.
Nonetheless, any osteosynthesis devices and treatment strategies were evaluated in sheep under clinically relevant conditions.(25,166,167) Furthermore, osteoporotic fractures in metaphyseal regions can be modeled in this species, which is difficult in small animals.(130,168,169) Other sheep models to study centrally induced bone loss have been developed.(152,158,170‐173) One example is the study from Bindl and colleagues, which analyzed metaphyseal fracture healing after surgical disconnection of the hypothalamus and pituitary gland, resulting in delayed bone formation, as it is often observed in osteoporotic patients.(174) These complex models lead to a considerable decline of bone mass(158,172) and closely resemble the human situation(170,171,174) but are challenging and raise similar ethical questions as the GC therapy.
Besides sheep, non‐human primates and species like rhesus macaques, Macaca mulatta, or Papio ursinus have been used for bone research.(175‐177) For ethical reasons, these experiments are not possible in Europe. Several successful approaches highlight the translational potential of these models, for example, in demonstrating that alcohol is a risk factor for osteoporosis after HIV infection.(175) Non‐human primates have also been used to test biologicals for osteoporosis treatment or fracture healing.(178,179) Particularly in translational bone research, non‐human primates are the most accurate model in terms of bone metabolism and structure.
In summary, large animal models are indispensable for specific skeletal research questions because they can facilitate the process from bench to bedside. A great drawback, the difficulty of genetic engineering, could be overcome in the near future. Indeed, the first CRISPR/Cas9 transgenic sheep model for hypophosphatasia was recently generated and recapitulates dentoalveolar defects reported in humans.(18)
Discussion
Currently, scientists working with animal models in skeletal research are faced with fast methodological progress and rapidly changing legislative and ethical societal landscapes in which to navigate. The relatively new ease of genetic manipulation on the one hand and increased awareness of a reproducibility crisis and demands for improved animal welfare calls for an assessment of current animal experimentation and guidelines that help scientists to conduct their research as sensibly and effectively as possible and boost translation.
Standards for animal experiments in skeletal research
Around the time of the early bone cell cultures, in 1959, Russel and Burch published a milestone for humane animal research and urged the implementation of the 3 Rs (replace, reduce, refine).(180) It has become clear that these 3 Rs are not enough and the data from these experiments also need to be robust, registered, and reported (6 Rs) to ensure scientific value.(181)
Since the EU Directive 2010/63/EU on the protection of animals used for scientific purposes took effect in 2013, the transition into the labs has been slow. The 3R‐based directive has a wider scope and defines strict regulations on housing standards, experimentation, and care. Granted experiments require strict minimization and assessment of pain and distress and categorization of experimental severity. Furthermore, regular risk‐based inspections are carried out and transparency is improved through retrospective assessments and the publication of non‐technical project summaries for the lay public. Still, the legislation on animal experiments has not changed as much as the general awareness of the issue. It is, therefore, important to point out that granting of the planned experiment by the local authorities ensures legal safety and strict ethical regulation. Transparency protects from legal charges, and careful experimental planning with meticulous documentation ensures scientific value. Nevertheless, the limited flexibility of granted experiments and increased time demand for application and documentation result in ethical problems of their own. Mendelian ratios and gene manipulation effects may dictate a greater number of animals being born than will be used for the actual experiment. The euthanasia of (surplus) animals should only be the result of a careful breeding plan and evaluation of possible alternative uses.(182) Before planning animal experiments, scientists have to justify which species and strain, model, and tissues to analyze, as well as the sex, age, and other treatments affecting mineral and hormonal homeostasis of the bone, such as mechanical strain and nutrition. In line with ethical regulations, projects are under constant species‐specific animal care supervision and subjected to scientific and ethical approvals. There is thus currently an efficient system in place to guarantee the ethical and minimal usage of animals for research.
One major step toward improving animal research and giving scientists some guidelines came with ARRIVE in 2010: a list of 20 recommendations for correct reporting of small animal experiments including, among others, study design, sample size, inclusion/exclusion criteria, and randomization. These criteria were developed in consultation with scientists, statisticians, journal editors, and several public or federal funding agencies.(183) Despite positive resonance, these guidelines are not comprehensively followed, although more journals now require authors to refer to them. Ten years later, ARRIVE has been revised to further facilitate its use.(184) Other initiatives such as the European Quality in Preclinical Data (EQIPD) aim at improving the planning stage of research.(185,186) Preregistration of experiments to increase liability and visibility is also increasing.
For the bone field, Manolagas and Kronenberg proposed several still‐valid points to overcome the reproducibility crisis in 2014.(187) They urged scientists and journals to endorse and use guidelines for the reporting of small animal skeletal phenotypes to ensure consistency and reproducibility (currently for animal experiments, the ARRIVE [2.0] guidelines [2020](184); for bone histomorphometry, the ASBMR nomenclature guidelines [2012](188); for μCT, the JBMR [2010] guidelines,(189) as well as the ASTM standard F2721 [2014] for segmental bone defects(190)). The authors also advocated an increased availability of statistics and data reporting courses and encouraged the field to not shy away from vigorous scientific debate. In grant applications and manuscripts, greater emphasis was asked to be placed on scientific premises and rigor, instead of novelty and publication speed.
In addition, we believe that animal models should prioritize clinical targets for functional studies. Further important improvements toward the 6R pledge include developing well‐annotated, accessible reference variant‐phenotype databases, adopting phenotype description standards, data sharing, and systems to collect validated alternatives. Around 20% of all animal testing is performed for regulatory purposes.(191) Here, greater harmonization of the regulatory requirements between agencies of different countries can reduce required animal numbers.
Future developments for animal experiments in skeletal research
Non‐animal methods (NAMs) are not a by‐product of research. They require a clear vision, a dedicated development path, and a strategy for validation, standardization, and regulatory approval. Once alternatives are approved for drug testing, it should no longer be allowed to perform the corresponding in vivo experiments. This is currently the case in the toxicology community, where organizations like the European Union Reference Laboratory for Alternatives to Animal Testing (EURL‐ECVAM, for validation of non‐animal alternatives)(192) and the Organization for Economic Co‐operation and Development (OECD, for guidelines, harmonization, and good practice development) play an important role. Policy decisions and investments have allowed the development of new methodologies like serum‐free cell culture, organ‐on‐a chip, or advanced in silico modeling, which will also benefit the bone field.
Nevertheless, recent discoveries using lineage tracing in genetically altered animals for the characterization of skeletal stem cells,(139‐141) subtypes of vasculature,(142,143) and lymphatic vessels demonstrate the value of the use of animal experiments for basic research that cannot be performed in in vitro models.
We believe it is obvious that the progress and success of skeletal research are highly dependent on the use of a variety of different models (Fig. 1). Each model has advantages and limitations that are useful to be aware of. In vitro assays can provide answers in terms of cell signaling, gene expression, and cell behavior, but in an often non‐physiological, artificial context with higher levels of oxygen and nutrients and without systemic context. In contrast, in the whole animal, cellular behavior is investigated within its physiological context and is thus more likely to represent biologically relevant mechanisms. Furthermore, aging, a very important aspect of bone biology and health, can be investigated realistically only in whole organisms. But the complexity at the structural, cellular, mechanical, and endocrine/paracrine levels makes it more challenging to correctly interpret phenotypes. In silico approaches can integrate data and/or knowledge acquired from in vitro or in vivo experiments and provide a computational framework to test biological hypotheses, run large‐scale screening experiments, optimize treatment design, and perform in silico (clinical) trials. Computer models now allow the first predictions of loss and maybe gain of functions of genes on cellular differentiation patterns in bone biology, providing an excellent tool to decide whether a genetic animal experiment is likely to reveal a phenotype and thus a functional explanation of novel factors in bone growth and physiology.(66) However, credibility assessment requires the availability of high‐quality dedicated data that cannot be sourced sufficiently from the currently available in vitro models or human clinical studies. The goal can only be to use the best possible combination of methodology that involves scientific rigorousness and considers ethical awareness and restrictions to understand fundamental biology and develop advanced treatment options for bone diseases.
Author contibutions
M.S. and J.T. conceptualized the manuscript, MS, FE, BB, IF, RYK, EF, MA, AI, LG, LG and JT wrote the first draft and edited and revised the manuscript.
Acknowledgments
We are grateful to the members of the Basic Science Action Group of the European Calcified Tissue Society (ECTS) for critical reading of the manuscript, namely, Michaela Tencerová, Claudine Blin‐Wakkach, Katherine Staines, Kent Soe and Sylvain Provot. This work was supported by the 3R‐Network BW to JT and by the German Research Foundation (CRC1149, project number 251293561, INST 40/492‐3 to AI and JT and INST 40/682‐1 to AI), and by the National Institute of Arthritis and Musculoskeletal and Skin Diseases of the National Institutes of Health (award number AR074417 to RYK and R01AR077949 to FE), and the European Research Council (ERC) under the European Union's Horizon 2020 research and innovation program (grant agreement no. 772418 to LG). The authors acknowledge that the figure was drawn using BioRender.com. Open Access funding enabled and organized by Projekt DEAL.
Peer Review
The peer review history for this article is available at https://www.webofscience.com/api/gateway/wos/peer-review/10.1002/jbmr.4868.
Disclosures
The authors have no conflicts of interest. JT is a member of the ECTS Basic Science Action Group. BB is a member of the Board of Directors of the ECTS (European Calcified Tissue Society).
References
Summary report on the statistics on the use of animals for scientific purposes in the member states of the European Union and Norway in 2019; https://ec.europa.eu/environment/chemicals/lab_animals/pdf/SWD2019_Part_A_and_B.pdf