-
PDF
- Split View
-
Views
-
Cite
Cite
Abubakar Dar, Zahir Ahmad Zahir, Maqshoof Ahmad, Azhar Hussain, Muhammad Tauseef Jaffar, Robert Jhon Kremer, Bacterial allelopathy: an approach for biological control of weeds, Journal of Applied Microbiology, Volume 135, Issue 9, September 2024, lxae219, https://doi.org/10.1093/jambio/lxae219
- Share Icon Share
Abstract
Weed infestation is one of the most damaging biotic factors to limit crop production by competing with the crop for space, water, and nutrients. Different conventional approaches are being used to cope with weed infestation, including labor intensive manual removal and the use of soil-degrading, crop-damaging, and environment-deteriorating chemical herbicides. The use of chemicals for weed control has increased 2-fold after the green revolution and their non-judicious use is posing serious threats to mankind, animals, and biodiversity. The detrimental effects of these approaches have shifted the researchers’ attention from the last two decades towards alternate, sustainable, and eco-friendly approaches to cope with weed infestation. The recent approaches of weed control, including plant and microbial allelopathy have gained popularity during last decade. Farmers still use conventional methods, but the majority of farmers are very passionate about organic agriculture and describe it as a slogan in the developed world. The effectiveness of these approaches lies in host specificity by selective bacteria and differential response towards weeds and crops. Moreover, the crop growth promoting effect of microorganisms (allelopathic bacteria) possessing various growth promoting traits, that is, mineral solubilization, phytohormone production, and beneficial enzymatic activity, provide additional benefits. The significance of this review lies in the provision of a comprehensive comparison of the conventional approaches along with their potential limitations with advanced/biological weed control approaches in sustainable production. In addition, the knowledge imparted about weed control will contribute to a better understanding of biological control methods.
Introduction
The green revolution on one hand enhanced the production through high-yielding varieties but on the other hand lack of resistance against pest and diseases has resulted in the utilization of agro-chemicals (pesticides), which has increased up to 20-folds worldwide in the last 50 years for control of biotic stresses, that is, weeds, pests, and diseases (Oerke 2006). Among these, weeds are causing more loss in crop yields than other biotic stresses by competing for nutrition, water, and space (Froud-Williams 2002). Weeds alone have potential to reduce crop yields up to 34%, which is higher than any other pest, including pathogens causing up to 16% reduction in crop yields and up to 18% reduction in animals (Oerke 2006). As compared to crops, weeds can germinate and grow with greater densities under stressful and non-stressful temperature regimes due to lack of weed control (Matloob et al. 2015). Weeds mostly affect plants by competing with crops for nutrients, water, space, release of allelochemicals and through shading, which reduce yield and quality (shriveled grains, nutritional status) of crop (Rajcan and Swanton 2001). In addition to yield loss, weeds also contribute indirectly to crop damage, acting as hosts for destructive insect pests and pathogens (Booth et al. 2003).
Weed management involves direct and indirect mechanisms. Direct mechanisms aim to control weed population densities, whereas indirect methods involve the adoption of certain preventive measures involving cultural practices helpful in reducing germination, growth, and reproduction of weeds (Shad 2015). Conventional approaches are being used for the eradication of weeds from crop land, that is, manual, mechanized/mechanical, and chemical weeding (Chauvel et al. 2012). These methods are helpful in controlling weeds but have few limitations, that is, manual methods require skilled labor, mechanical methods emit greenhouse gases and chemicals pose serious threats to humans and biodiversity, a serious concern in sustainable crop production. It is therefore critical to find weed control solutions (biological weed control) with minimum damage to the environment, soil biodiversity, human health, and food quality. Therefore, this review provides a comparison of conventional weed-control approaches with biological approaches for sustainable crop production.
Conventional weed control
The conventional weed control strategies include manual, mechanical, and chemical methods for weeds management. The adoption rate of these methods is variable depending upon the topography, skilled labor, advanced machinery, and availability of chemicals (Datta and Knezevic 2013). Manual weeding involves weed control by skilled labor through hoeing, plucking, and pulling with or without localized hand-driven equipment. This has been an effective way to reduce weed population in crops (Piggin et al. 2001, Young et al. 2014). Manual weeding provides efficient weed control in almost all crops at any growth stage and under many field conditions, and in areas with cheap labor availability. However, manual weeding is no longer an effective/affordable method these days due to lack of skilled labor, which is more attracted towards the industries in the cities (Naylor 2008, Carballido et al. 2013). Also, repetition of weeding two or three times in a crop and involvement of intensive labor makes manual weed control non-affordable and non-feasible (Rao et al. 2007, Abbas et al. 2020a).
Mechanical weed control involves using tractor/animal driven implements, including weeders, cultivators, and harrows to eradicate weeds. Mechanical weed control operates with less labor and saves considerable time compared with manual weed control. The mechanical methods are suitable only for crops grown in rows having enough row-to-row distance to make movement of implements feasible, that is, harrows, torsion weeder, finger weeder, and weed blower (Van der Weide et al. 2008). A major limitation to this technique is the inability of these implements to discriminate between crop plant and weed, that causes injury to crop as well (Abbas et al. 2020c). Weeds that grow in crop rows are the main problem because the chances of crop damage increase during their eradication. The equipment is mostly tractor driven, and the heavy load of tractors may lead to soil compaction and deterioration of soil quality, that is, enhanced soil erosion, aggregates breakage, nutrient leaching, surface runoff, eutrophication, and global warming (Ahlgren 2004, Smith et al. 2011). Other drawbacks include destruction of soil biodiversity, soil fauna habitats, and higher fuel consumption (Culliney 2005).
Chemical weed control deals with the application of chemicals (herbicides) to kill and reduce the population of weeds in crops. Weed control was revolutionized in 1940s by application of two herbicides namely 2-methyl-4-chlorophenoxyacetic acid (MCPA) and 2,4-dichlorophenoxy acetic acid (2,4-D) for specific weed control in cereals (Zimdahl 1994). The reason for their popularity lies in their advantages provided in terms of reduced labor, cost effectiveness, time saving, energy saving, and reduction in soil erosion (Ghorbani et al. 2005). The limitations of chemical herbicides include environmental constraints, biodiversity loss, resistance in weeds, and human health issues. Soloneski et al. (2016) estimated that only 0.1% of the total herbicides used to control weeds reached their specific targets and the remaining amount released in the environment caused multiple damages like (i) weed floral shift. For example, continuous application of 2,4-D for broad-leaved weeds has shifted weed flora towards grasses after a certain period and reduced its efficiency against the target weeds (Hakansson 2003), (ii) development of herbicide-resistance in weeds; various reports regarding herbicide-resistance in weeds have been published during the last 10 years (Heap 2014; Bo et al. 2017; Travlos, et al. 2020; Ofosu et al. 2023). Presently, 523 unique cases regarding herbicide-resistance in 269 weed species have been registered against 167 herbicides globally (Heap 2023), (iii) persistence in nature; herbicides are xenobiotics and have ability to persist in the environment for longer periods without degradation (McLaughlin and Kinzelbach 2015), (iv) damage to soil biodiversity; another harmful aspect of chemical herbicide use is the irreversible damage to non-target species, including wildlife and beneficial microbial diversity (Hakansson 2003, Pacanoski 2007, Geiger et al. 2010), (v) water pollution; as the concentration of chemicals in groundwater, lakes, and rivers goes above 200 ppb, causes water pollution (Reddy et al. 1997; Hamilton et al. 2003), deterioration of human health by accumulation of chemicals in the edible portion of plants and entering the food chain. Boedeker et al. (2020) estimated that about 385 million people were suffering from unintentional acute pesticide poisoning per annum worldwide, whereas about 1.0 million deaths by herbicidal exposure are reported every year due to chronic diseases from herbicide residues (Blair et al. 2015).
Biological weed control
Biological weed control is the use of living entities and their products (phytotoxins, secondary metabolites) for growth suppression, reducing population densities, vigor, and reproductive capacity of targeted weed without harm to the main crop (Charudattan 2005, Mustafa et al. 2019, Polyak and Sukcharevich 2019, Rasool et al. 2019). The first evidence of biological control has been reported as protection of natural enemies 2000 years ago in China (van Lenteren et al. 2018; Shields et al. 2019). However, modernization of this technique appeared about 200 years ago (DeBach 1964, van Lenteren and Godfray 2005). Biological weed control has benefits over the other weed control techniques because (1) the introduced agents can perpetuate and distribute themselves throughout the weed's range; (2) the impact of host-specific agents is focused on a single weed species without harm to other plants; (3) the cost of developing biological control is relatively inexpensive ($1.5 million) compared to much higher costs for other approaches (Andres 1977; Harris 1979); (4) the agents are non-pollutive, energy efficient, and biodegradable; and (5) the knowledge generated during pre-release and evaluation studies contributes to improved understanding of weed ecosystems and environmental factors regulating natural communities (Goeden and Andres 1999). Natural enemies of weeds limit weed population to its natural range and play significant role in balancing natural biodiversity (Bellows 2001). Broadly, biological control agents are of two types: those that directly interfere with weed physiology and those that expose the weed plant to insect and disease attack (Bellows and Headrick 1999).
The biological control can be further divided into four distinct categories: (i) natural biocontrol, (ii) conservation biocontrol, (iii) classical biocontrol, and (iv) augmentative biocontrol (Cock et al. 2010).
Natural biological control
The major share in biological control is contributed by nature. The pest population is controlled by a natural antagonist (beneficial microorganisms) without any human effort (Reid et al. 2005). The phenomenon of natural biological control is spontaneously occurring in all ecosystems of the world when and where needed, irrespective of the fact that anthropogenic interventions and their benefits are very high in terms of economics (Waage and Greathead 1988).
Various reports on natural biological control are available that show the availability of naturally occurring antagonist populations in soils. The molecular analysis of the community structure showed the presence of biological control agents in the soil without inoculation (Stenberg et al. 2021). Shen and his colleagues (2015) checked the community structure of two different orchards with and without Fusarium wilt infected banana plants and found that both orchards contained different microbial community structures. The orchard free from Fusarium wilt was abundant in Chthonomonas, Pseudomonas, and Tumebacillus. The comparison of these genera in both types of soil indicated a positive correlation of Pseudomonas genera with suppression of Fusarium wilt in banana. Furthermore, the term ‘soil suppressiveness’ was used in plant pathology by Cook and Baker (1983) to represent the natural biological control of plant diseases generally through involvement of the whole microbiome of this soil or specifically through involvement of specific taxa against specific disease (Kwak and Weller 2013). This suppressive activity of soil is spontaneous without any external aid so referred as perfect example of the natural biological control as described by the Siegel-Hertz et al. (2018). Alongside the weed seed predation by the native vertebrates and invertebrates is an example of natural biological weed control as described by White et al. (2007).
Conservation biological control
As the term suggests, the conservation biocontrol strategy involves protecting and conserving natural antagonists for biocontrol of pests and weeds; the first type of biological control approach involves human intervention and is a readily available practice in pest management but may not be suitable for all types of pests and weeds (Trognitz et al. 2016). In other words, environmental modification or modifying cultural practices to protect and boost natural antagonists for reducing deleterious effects of crop pests is called conservation biocontrol (Eilenberg et al. 2001). This approach not only conserves the natural microbiome but also reduces the occurrence of plant diseases and provides resilience against pathogen infections (Weller et al. 2002, Mendes et al. 2011). Conservation biocontrol not only focuses on enhancing population densities of natural antagonists but also diversifies their biodiversity (Cardinale et al. 2003). The strategy is based on providing better and richer diets so that the natural antagonists can form higher populations to ensure effective biocontrol of the target pest (Koji et al. 2007).
Few examples of conservation biological control include strip harvesting of alfalfa (Medicago sativa) in California to protect the cotton (Gossypium hirsutum) from its natural antagonists lygus bug (Lygus hesperus Knight) was an early example of conservation biological control (Ehler 1998; Shields et al. 2019). Moreover, the provision of an alternate host for the biocontrol agent to maintain their population throughout the year is another example of conservation biological control. Hardy (1938) controlled Plutella maculipennis (insect of cruciferous crops) by raring Angitia cerophaga on alternate host Cnephasia wahlbomiana, in England. The term ‘suppressive soils’ is used to indicate soils having natural capacity to reduce/cure diseases that is a natural biocontrol approach. These soils can be used to protect specific crops against different diseases and weed biotypes (Eilenberg et al. 2001). However, the natural soils can be converted to suppressive soils by adding organic matter to enhance saprophytic microbial populations. As suggested by Campbell (1989) green organic matter addition to avoid potato scab by controlling its pathogen Streptomyces scabiei is a classic example of conservation biological control. This biological pest control approach is effective in the long term, but climate change, industrialization, urbanization, and deforestation are the main causes of the destruction of the natural habitats of friendly microflora and fauna.
Classical biological control
The term classical biological control can be defined as “introduction of the natural antagonist into a new environment aiming at a long-term control of an exotic species (weed)” (Eilenberg et al. 2001, Scheepens et al. 2001). The term “classical” refers to its early development and use in biocontrol of pests (Greathead 1994). Furthermore, this strategy has been used widely for insect herbivores to eradicate weeds from arable land (Panta et al. 2024). This approach also involves microorganisms for pests (arthropods) and weeds biological control in arable land (Hajek et al. 2000; Oerke 2006). The principal objective of this approach is the permanent establishment of the natural antagonist in an area for long term effectiveness which differentiates this approach from other biological control approaches (Eilenberg et al. 2001). This approach deals with selection of a biological control agent from its native region and its application to the area invaded by exotic species (weed/unwanted plant) (Charudattan and Dinoor 2000). After release of the biocontrol agent in the environment, the biocontrol agent acclimatizes itself to the environmental conditions and maintains its population to control the target host (Goeden and Andres 1999). Great care is needed after release of the biological control agent as the introduction is irreversible and it is necessary to test the agent against non-target species prior to release. Sometimes it happens that the agent changes its targeted host after release as described by Scheepens et al. (2001). In 1795, the first success of classical biocontrol was reported for cochineal (Dactylopius ceylonicus), first released into India and accidently moved to Sri Lanka to control prickly pear cactus (Walton 2005). Moreover, insects with phytophagous nutritional habits are generally used for classical weed biocontrol (Goeden 1988).
Another example of classical biocontrol is the introduction of Cactoblastis cactorum in Australia from South America in mid 1920s to control the prickly pear cactus (Opuntia cacti). Three million eggs of C. cactorum were spread in the infested field, which suppressed the dense cactus population to few plants (Tsukamoto et al. 1997; Zimmermann et al. 2000). Tanner and his colleagues (2015) introduced rust fungus (Puccinia komarovii var. glanduliferae) against Impatiens glandulifera in England. Biocontrol agents usually have a wide host range but the provision of conducive environmental conditions under conservation approach enhance the chances of its success against the targeted invasive plants (Ghorbani et al. 2005, Dagno et al. 2012). Weedy species of Rubus alceifolius, Chondrilla juncea, Ageratina riperia, Carduus thoermeri, and Acacia saligna have been successfully controlled through the classical approach by introducing exotic saprophytic fungal species including rust and smut fungi (Leiss 2001).
Development of a classical biocontrol agent requires considerable time (10–20 years) and capital (millions of dollars) for its foreign exploration, pre-release evaluation and post-release monitoring (McFadyen 2000). Quarantine evaluation is another aspect of time consumption in approval of the introduction of biocontrol agent to natural environment. Culliney et al. (2003) reported such case of Acythopeus spp. approval in Hawaii required seven years under quarantine before approval for introduction to control invasive cucurbits. Four cases of non-target effect were reported in Hawaii by Funasaki et al. (1988) while McFadyen (1998) reported eight new cases of non-target effects by insect biocontrol agents worldwide. For example, European weevil introduction to control exotic thistles in the USA during 1969 (Julien and Griffiths 1988) significantly reduced population densities of Cirsium spp. by reducing seed production and threatening some native and endangered species as well (Louda et al. 1997).
Augmentative/inundative biological control
This strategy refers to the periodic application of natural antagonists already present in an area as and when required. These types of biocontrol agents are not self-sustaining and may require multiple applications in a year to control weeds (Hakansson 2003, Bale et al. 2008, Parnell et al. 2016). Inundation provides an advantage over classical approaches by introducing exotic species (Charudattan 2005). An augmentation approach is often used for potential development of commercial bioherbicides. Most of the bioherbicides originate from fungi also known as mycoherbicides (Dagno et al. 2012). It has been reported that these bioherbicides are applied worldwide on an area of 30 million hectares (van Lenteren et al. 2018).
Hasan et al. (2021) enlisted various bioherbicides of plant origin commercially available for weed control in different ecosystems including WeedZap®, Avenger® Weed Killer, Weed Slayer®, GreenMatch™ EX, BioWeed™, Beloukha®, and Sarritor®. Khamare et al. (2022) also provides a comprehensive review on the role of different allelochemicals in weeds’ suppression, various methods of weed control by managing cultural practices; namely, intercropping allelopathic crops, mulching, crop rotation, cover crops, and use of aqueous extracts of allelopathic plants. Moreover, enlisted plant originated bioherbicides of different origin but most of them are non-selective or not host specific that becomes a major concern using these chemicals in weed control. The first mycoherbicide was commercialized in 1980s under the brand name of DeVine in Florida for controlling strangler vine (Araujia odorata) in citrus (Kenny 1986). As of 2012, sixteen bioherbicides were registered in different countries with the common attribute of phyto-pathogenic fungi with brand names of Dr. BioSedge, BioMal, Stump-Out, and Collego (Dagno et al. 2012). The first bacterial based bioherbicides Camperico, which is Xanthomonas campestris pv. poae was used in golf courses for controlling Poa annua (Charudattan 2001) and recently Pseudomonas fluorescence strain D7 has been commercialized through Verdesian Life Sciences, for biocontrol of downy brome (Kennedy 2018, Lazarus et al. 2021). About 170 species were used as augmentative biocontrol agents in Europe (Cock et al. 2010). Van Lenteren (2012) reported 230 species of augmentative biocontrol agents in integrated pest management worldwide. Furthermore, a survey in 2016 documented 350 biocontrol agents used worldwide instead of augmentation biocontrol (van Lenteren et al. 2018). Worldwide pesticides consumption during 2018, was estimated at 64.04 billion US$ with an expected annual growth rate of 5%–6% during next five years (Research and Markets 2019a). However, the global bioherbicide market was estimated to grow up to 6.3 billion US$ by 2026 from 1.7 billion in 2015 (Dunham 2015) with 17.7% expected increase between 2018 and 2026 (Research and Markets 2019b). The higher potential of expected growth showed the scope of bioherbicides for sustainable agriculture in future which has been due to higher demands of organically produced food in the developed world. The world's largest biocontrol agent commercial market is in Europe while North America provides higher sales of microbials. Moreover, Asia and Latin America have strong growth regarding microbial inoculants (van Lenteren et al. 2018).
The main hinderance in success of biocontrol approach is the provision of conducive environmental conditions (temperature, air, dew period, moisture contents, and environmental humidity) that fluctuate under natural field conditions (Charudattan 2001, Ghorbani et al. 2005).
Efficacy of the biocontrol agent against single weed or related weed biotypes can be witnessed after control of these fluctuating conditions (Rosskopf et al. 1999). The economics of using bioherbicides (augmentative approach) suggested to utilize such agents, which have ability to control multiple weeds (broad spectrum biocontrol agent) or using multiple agents in one product to have effective control over weed populations (Chandramohan et al. 2000). For example, a bioherbicide emulsion containing fungal biocontrol agents, that is, Drechslera gigantea, Exserohilum rostratum, and E. longirostratum was used for biocontrol of seven grassy weeds under pot and field studies. Furthermore, formulation of a bioherbicide plays a critical role in the success of the product not only in application but also its bioherbicidal activity (El-Morsy et al. 2006). Requirement of higher humidity may contribute to non-effectiveness of bioherbicides, and desiccation of control agents leads to ineffectiveness due to cellular damage through exposure to dry heat and UV radiations (Patel and Patel 2015). In a recent study, Duke (2024), described different reasons that why in the past bioherbicide products are not successful; namely, narrow host range, short shelf life, unfavorable storage conditions, lower persistence, older formulations and application technologies, massive application requirement, and genetic alteration of the active microbes. These problems can be solved either by using consortium of bacteria to enhance their range of target species, adoption of the latest technologies, and the use of only active phytotoxins from the bacterial culture rather than the live bacteria (Duke 2024).
The described approaches are used in developing bioherbicides throughout the world. The research on weed biocontrol is primarily focusing on the augmentative or inundative approaches for its practicality and application in organic agriculture. Moreover, the use of phytotoxic metabolites (allelochemicals) of plant and microbial origin are good alternates of chemical herbicides for development of such bioherbicide products (Abbas et al. 2018).
Plant allelopathy
The importance of plant based phytotoxic chemicals in biological weed control increased when the term ‘allelopathy’ was coined by Molisch (1937) by describing two Greek words ‘Allelo’ and ‘Pathos’ meaning mutual sufferings by explaining the role of ethylene in the fruit ripening process. Later, allelopathy was defined by Rice (1984) as “any direct/indirect, positive/negative effect of a plant on the other by releasing harmful chemicals in the immediate environment.” More often, allelopathy is defined as growth inhibition of one plant species by another pant specie through chemicals released into the environment (Inderjit and Callaway 2003).
Classification and biosynthesis of allelochemicals
Plants and microorganisms release allelochemicals to survive in the competition and as a natural defense mechanism against invasive plant species (Mattner 2006). The allelochemicals are introduced into the environment by (i) root exudation (Inderjit and Weston 2003), (ii) leaching, (iii) volatilization, and (iv) decomposing plant debris (Jabran et al. 2015). The biosynthesis of these allelochemicals depends upon the compound, plant type, age, and environmental conditions (Latif et al. 2017). Moreover, plant allelochemicals are released from different plant tissues, that is, leaves, roots, stem, flowers, rhizomes, ripened seeds, buds, pollens, and plant bark by specified mechanisms, including leaching and volatilization from aerial plant parts and residue decomposition and root exudation in soil (Weston and Duke 2003). Allelochemicals belong to various classes on the basis of chemistry: (i) organic acids, alcohols, ketones, and aldehydes, (ii) unsaturated lactones, (iii) long-chain fatty acids and polyacetylenes, (iv) quinones (v) phenolic acids, (vi) flavonoids, (vii) tannins, (viii) cinnamic acid, (ix) coumarins, and (x) steroids/terpenoids (Li et al. 2010). The biosynthesis pathways of different allelochemical classes from shikimic acid have been described in Fig. 1.
Phenolics are a diverse class of allelochemicals/organic compounds categorized as secondary metabolites, which are biosynthesized through phenylpropanoid-acetate pathway (Haig 2008). Another class of allelochemicals is terpenoids (component of plant essential oils) comprised of five carbon isoprene. This class of allelochemicals can be biosynthesized from the mevalonic acid/isopentenyl pyrophosphate pathways (Latif et al. 2017).
Heterocyclic nitrogen-containing compounds of plant origin are classified as alkaloids possessing important pharmacological properties, common allelochemicals of Asteraceae, Boraginaceae, Apocynaceae, and Fabaceae plant families (Yang and Stöckigt 2010). Alkaloids have been classified into different classes based on their biosynthesis pathways: (i) indole alkaloids (tryptophan biosynthesis pathway), (ii) pyrrolizidine alkaloids (ornithine or arginine biosynthesis pathway), and (iii) quinolizidine alkaloids (lysine biosynthesis pathway) (Seigler 1998). Hydroxamic acids are another type of plant allelochemical having phytotoxic, antimicrobial, antifeedant, and antifungal properties. Mostly released in the soil by microbial degradation of plant residue from cereals (Chiapusio et al. 2005).
Glucosinolates are the sulfur-containing compounds (bitter) present in allelochemicals of Brassica spp. upon enzymatic hydrolyzation are converted to more harmful allelochemicals; namely, isothiocyanates, thiocyanates, and nitriles reported for effected weeds suppression (Hasan et al. 2021). The most important compound isothiocyanate upon interacting with sulfhydryl group-containing enzymes not only control several microbial pathogens but also suppress the germination of weeds like Matricaria inodora L., Sonchus asper L., Echinochloa crus-galli, Alopecurus myosuroides, and A. hybridus (Tsiamis et al. 2016).
Plant allelopathy and weed suppression
The phenomenon of allelopathy is being used widely in weed control under different cropping systems as well as cropping zones (Farooq et al. 2011, 2013). The allelochemicals released by plants have multiple modes of action, including retarded growth and development, hormonal imbalance, impairing enzyme activities, plant physiological processes (photosynthesis and respiration), and plant water relations (Kruse et al. 2000, Farooq et al. 2013). For example, higher concentrations of allelochemicals during early growth stages of the target plant may have higher suppression than at later stages (Inderjit et al. 2005) while lower concentrations may stimulate the growth of target plants called as ‘hormesis’ (Belz et al. 2005). External factors, that is, biotic or abiotic stresses cause increases in synthesis and release of allelochemicals (Einhellig 1996). However, for effective control of weed species six points denoted as Willis six points needs to be fulfilled; namely, (i) allelopathic species should have a pattern of inhibition on the other, (ii) the allopathic species should produce a toxin, (iii) the plant has a specific mode of toxin's release in the environment, (iv) the toxin can accumulate and possess means of transportation to targeted species for inhibition, (v) the host plant must imbibe the toxin, and (vi) the pattern of inhibition will not be resembled with other biotic factors (nutrient competition and predation) in soil (Willis 1985; Hickman et al. 2023). Moreover, Hickman et al. (2023) also suggested to know the biology and allelopathy of the target weed for an effective weed control rather than focusing on the allelopathic crop and managing cultural practices to overcome the noxious weeds. The mechanism of allelochemicals for weed suppression include (1) imbalance weed growth regulators, (2) alteration of membrane permeability, (3) imbalance in water and nutrients uptake, (4) impairment of antioxidants and photosynthetic activities, and (5) abnormal enzymatic functioning (Kostina-Bednarz et al. 2023).
Various food and fodder crops are known to produce allelochemicals, that is, wheat, maize, rice, oats, barley, lupins, beet, rye, brassica, sorghum, and sunflower (Saxena et al. 2016). Roots of rye produce phenolics, 2,4-dihydroxy-1,4-benzoxazin-3-one (DIBOA), 6-methoxy-benzoxazolinone (MBOA), 2-benzoxazolinone (BOA), and hydroxamic acids (Reberg-Horton et al. 2005; Jilani et al. 2008). Microbial degradation in soil converted 2-BOA to 1-azobenzene (AZOB), which is more toxic and inhibitory to plants than DIBOA (Chase et al. 1991). Several Brassica spp. have shown allelochemical potential and weed suppression attributes due to production of glucosinolates (Bialy et al. 1990). Brassica juncea seed meal was used for the weed suppression in truf and found to be effective in suppressing germination of annual bluegrass (P. annua), buckhorn plantain (Plantago lanceolata), large crabgrass (Digitaria sanguinalis), common chickweed (Stellaria media), and white clover (Trifolium repens) by 27%–50% as compared to control (Earlywine et al. 2010). Furthermore, different plant residues of black mustard (Brassica nigra L.) have potential of suppressing growth and reducing germination of wild oat, alfalfa, radish, and lentils (Rehman et al. 2013). A broad spectrum allelopathic plant rye (Secale cereale L.) reduces the Amaranthus spp. by 80%–90% through using it as cover crop (Wato 2020). Allelochemicals produced by rye include 2,4-dihydroxy-1,4-(2H)-benzoxazin-3-one (DIBOA) and 2(3H)-BOA responsible for reduction of monocotyledons and di cotyledons, respectively (Barnes et al. 1986). Multiple allelopathic plants and their target weeds data are described in Table 1.
Allelopathic plant/compound . | Target weed species . | Reported by . |
---|---|---|
Black mustard (B. nigra L.) | Avena fatua L. | Wato (2020) |
Billy goat weed (Ageratum conyzoides L.) | Echinochloa crus-galli L. | Wato (2020) |
Rye (S. cereale) | Amaranthus spp. | Wato (2020) |
White tephrosia (Tephrosia candida) | Phalaris minor Retz. | Wato (2020) |
Wheat cultivars | Phalaris minor Retz. | Kashif et al. (2015) |
Moringa oleifera, Parthenium hysterophorus, and Cannabis sativa | Avena fatua, Carthamus oxyacantha, Chenopodium album, Convolvulus arvensis, Euphorbia helioscopia, Fumaria indica, P. minor, Sonchus oleraceus | Gurmani et al. (2021) |
Urochloa ruziziensis stems and Sorghum bicolor | Euphorbia heterophylla | Novakoski et al. (2020) |
Urochloa ruziziensis | Ipomoea triloba | Foletto et al. (2012) |
Sorghum (S. bicolor L. Moench) | Weeds | Sowiński et al. (2020) |
Plant extracts of black mustard (B. nigra L.) | Alfalfa (M. sativa L.), lentil (Lens culinaris Medikus), wild oat (A. fatua L.), and radish (Raphanus raphanistrum sub sp. sativus) | Rehman et al. (2019) |
Rapeseed residues | Hairy night shade (Solanum sarrachoides Sendtn) and long sandbur [Cenchrus longispinus (Hack.) Fern.] | Rehman et al. (2019) |
Seed meal of Indian mustard | Biomass of redroot pigweed (Amaranthus retroflexus L.) and common lambs’ quarters (C. album L.) | Rice et al. (2007) |
Rapeseed, Indian mustard, and Ethiopian mustard (Brassica carinata A. Braun) | Winter weeds | Rehman et al. (2019) |
Allelopathic plant/compound . | Target weed species . | Reported by . |
---|---|---|
Black mustard (B. nigra L.) | Avena fatua L. | Wato (2020) |
Billy goat weed (Ageratum conyzoides L.) | Echinochloa crus-galli L. | Wato (2020) |
Rye (S. cereale) | Amaranthus spp. | Wato (2020) |
White tephrosia (Tephrosia candida) | Phalaris minor Retz. | Wato (2020) |
Wheat cultivars | Phalaris minor Retz. | Kashif et al. (2015) |
Moringa oleifera, Parthenium hysterophorus, and Cannabis sativa | Avena fatua, Carthamus oxyacantha, Chenopodium album, Convolvulus arvensis, Euphorbia helioscopia, Fumaria indica, P. minor, Sonchus oleraceus | Gurmani et al. (2021) |
Urochloa ruziziensis stems and Sorghum bicolor | Euphorbia heterophylla | Novakoski et al. (2020) |
Urochloa ruziziensis | Ipomoea triloba | Foletto et al. (2012) |
Sorghum (S. bicolor L. Moench) | Weeds | Sowiński et al. (2020) |
Plant extracts of black mustard (B. nigra L.) | Alfalfa (M. sativa L.), lentil (Lens culinaris Medikus), wild oat (A. fatua L.), and radish (Raphanus raphanistrum sub sp. sativus) | Rehman et al. (2019) |
Rapeseed residues | Hairy night shade (Solanum sarrachoides Sendtn) and long sandbur [Cenchrus longispinus (Hack.) Fern.] | Rehman et al. (2019) |
Seed meal of Indian mustard | Biomass of redroot pigweed (Amaranthus retroflexus L.) and common lambs’ quarters (C. album L.) | Rice et al. (2007) |
Rapeseed, Indian mustard, and Ethiopian mustard (Brassica carinata A. Braun) | Winter weeds | Rehman et al. (2019) |
Allelopathic plant/compound . | Target weed species . | Reported by . |
---|---|---|
Black mustard (B. nigra L.) | Avena fatua L. | Wato (2020) |
Billy goat weed (Ageratum conyzoides L.) | Echinochloa crus-galli L. | Wato (2020) |
Rye (S. cereale) | Amaranthus spp. | Wato (2020) |
White tephrosia (Tephrosia candida) | Phalaris minor Retz. | Wato (2020) |
Wheat cultivars | Phalaris minor Retz. | Kashif et al. (2015) |
Moringa oleifera, Parthenium hysterophorus, and Cannabis sativa | Avena fatua, Carthamus oxyacantha, Chenopodium album, Convolvulus arvensis, Euphorbia helioscopia, Fumaria indica, P. minor, Sonchus oleraceus | Gurmani et al. (2021) |
Urochloa ruziziensis stems and Sorghum bicolor | Euphorbia heterophylla | Novakoski et al. (2020) |
Urochloa ruziziensis | Ipomoea triloba | Foletto et al. (2012) |
Sorghum (S. bicolor L. Moench) | Weeds | Sowiński et al. (2020) |
Plant extracts of black mustard (B. nigra L.) | Alfalfa (M. sativa L.), lentil (Lens culinaris Medikus), wild oat (A. fatua L.), and radish (Raphanus raphanistrum sub sp. sativus) | Rehman et al. (2019) |
Rapeseed residues | Hairy night shade (Solanum sarrachoides Sendtn) and long sandbur [Cenchrus longispinus (Hack.) Fern.] | Rehman et al. (2019) |
Seed meal of Indian mustard | Biomass of redroot pigweed (Amaranthus retroflexus L.) and common lambs’ quarters (C. album L.) | Rice et al. (2007) |
Rapeseed, Indian mustard, and Ethiopian mustard (Brassica carinata A. Braun) | Winter weeds | Rehman et al. (2019) |
Allelopathic plant/compound . | Target weed species . | Reported by . |
---|---|---|
Black mustard (B. nigra L.) | Avena fatua L. | Wato (2020) |
Billy goat weed (Ageratum conyzoides L.) | Echinochloa crus-galli L. | Wato (2020) |
Rye (S. cereale) | Amaranthus spp. | Wato (2020) |
White tephrosia (Tephrosia candida) | Phalaris minor Retz. | Wato (2020) |
Wheat cultivars | Phalaris minor Retz. | Kashif et al. (2015) |
Moringa oleifera, Parthenium hysterophorus, and Cannabis sativa | Avena fatua, Carthamus oxyacantha, Chenopodium album, Convolvulus arvensis, Euphorbia helioscopia, Fumaria indica, P. minor, Sonchus oleraceus | Gurmani et al. (2021) |
Urochloa ruziziensis stems and Sorghum bicolor | Euphorbia heterophylla | Novakoski et al. (2020) |
Urochloa ruziziensis | Ipomoea triloba | Foletto et al. (2012) |
Sorghum (S. bicolor L. Moench) | Weeds | Sowiński et al. (2020) |
Plant extracts of black mustard (B. nigra L.) | Alfalfa (M. sativa L.), lentil (Lens culinaris Medikus), wild oat (A. fatua L.), and radish (Raphanus raphanistrum sub sp. sativus) | Rehman et al. (2019) |
Rapeseed residues | Hairy night shade (Solanum sarrachoides Sendtn) and long sandbur [Cenchrus longispinus (Hack.) Fern.] | Rehman et al. (2019) |
Seed meal of Indian mustard | Biomass of redroot pigweed (Amaranthus retroflexus L.) and common lambs’ quarters (C. album L.) | Rice et al. (2007) |
Rapeseed, Indian mustard, and Ethiopian mustard (Brassica carinata A. Braun) | Winter weeds | Rehman et al. (2019) |
Autotoxicity in alfalfa is also an effect of allelochemicals, that is, phenols, saponins, chlorogenic acid, and medicarpin when a new crop of alfalfa is grown on same soil, which caused reduction in seed germination, growth, and biomass production (Dornbos et al. 1990; Seguin et al. 2002). Allelopathic potential of sorghum has also been investigated by production of sorgoleon from root hairs and root tips of sorghum. It mainly consists of p-benzoquinon and three other compounds of the same family that contribute about 90% of sorghum's exudation (Dayan et al. 2007). Cheema and Khaliq (2000) reported 15%–47% reduction in weed population density and 19%–49% less dry biomass production in C. album, Rumex dentatus, and P. minor in wheat (Triticum aestivum) by using sorghum (S. bicolor L. Moench) water extract. Furthermore, allelopathic secretions of rice consisting of different classes of chemicals include phenylalkanoic acids, phenolics, fatty acids, indoles, benzoxazinoids, and terpenoids (Belz 2007, Kato-Noguchi et al. 2008). The momilactone B diterpenoid exudation by rice was the most effective chemical for weed inhibition (Kato-Noguchi and Ino 2005).
Sunflower (Helianthus annuus) has been reported for suppressing broad-leaved weeds through herbicidal effects of its allelochemicals (Bogatek et al. 2006; Anjum and Bajwa 2007a, b). The crop residues of sunflower has the ability to reduce population densities of P. minor from 42% to 100% under field and axenic conditions, respectively (Om et al. 2002). The compounds responsible for herbicidal activity include di- and triterpenes (Macías et al. 2004). The effect of these crops can be used in multiple ways to overcome weed population densities, that is, incorporation of residues, mulching of allelopathic crops, incorporation of allelochemicals in crop rotation, and using them as smoother crops (Saxena et al. 2016). Wheat has also been reported to produce allelopathic effects (Mahmood et al. 2013) while using 35 cultivars against A. fatua. Among them eleven showed strong allelopathic effects by reducing 42%–83% growth in A. fatua. Dhima et al. (2006) used barley residues against E. crus-galli and Setaria verticillata infestation in maize as mulch. The allelopathic effect of these residues reduced seedling emergence by 0%–67% in S. verticillata and 27%–80% in E. crus-galli along with 45% increase in maize yield. Similarly, sunflower water extract has been studied for weed suppression in wheat crop. These studies reported 70% growth suppression of C. album and 97% of R. dentatus (Anjum and Bajwa 2007a, Naseem et al. 2009).
Allelochemicals have limited response in field compared to laboratory bioassays without soil (Kaur et al. 2005). This limited response is attributed to various factors like soil reactions and microbial community that these chemicals must face in soil (Kobayashi 2004). Soil composition (texture) plays a significant role in the leaching of allelochemicals. Oxidation and sorption are primary factors reducing the availability and toxicity of the chemicals by binding or changing oxidation states (Tharayil et al. 2008). Another factor responsible for low availability of allelochemicals to the target plants is the indigenous microbial community. Microorganisms may metabolize allelochemicals to attain energy through extra- and intracellular enzyme reactions on these chemicals to convert them either to simpler molecules or more toxic compounds (Bhinu et al. 2006). Modification in bioavailability and phytotoxicity of allelochemicals can also be influenced by natural biogeochemical cycles through immobilization and mineralization processes of essential nutrients (Inderjit 2006). Accordingly, allelopathy has promised as an alternative weed control method, albeit hindered by a wide range of contextual factors.
Plant microbiome and allelopathy
Plant microbiome and allelopathy are closely inter-linked phenomena in plant ecological study. These involve plants and their surrounding environmental interaction, including other plants and microorganisms. Plant microbiome refers to the community of microorganisms that inhabit the surfaces and internal tissues of plants (Jara-Servin et al. 2023). These microorganisms include bacteria, fungi, archaea, and viruses. The microbiome plays an important role in plant health and development, as it helps plants to acquire nutrients, resist disease, and adapt to environmental stressors (Khoshru et al. 2020). Plants secrete allelochemicals to survive in nutrient-limited environment, deter the herbivorous predators, and enhance beneficial microbiome in the rhizosphere (Zhong et al. 2022).
The plant's allelopathy and its microbiome are closely linked with the effects created by these secretions in the environment (Mishra et al. 2013). Some of the members of the microbiome can enhance the production or release of allelochemicals, increasing their effectiveness against target plants. Moreover, indigenous microbial communities are responsible for the fate and persistence of plant allelochemicals in soil; namely, microbial detoxification, adsorption to soil matrix, and toxicity to plants (Hussain et al. 2011; Vora 2022). Microorganisms such as Pseudomonas putida and Rhodotorula glutinis are responsible for the degradation of p-coumaric acid in Bamboos rhizosphere and reduce the impact of its toxicity to weeds (Zhang et al. 2010). In another study, Mishra and Nautiyal (2012) reported P. putida for reducing the impact of phenolic acids and parthenin released by P. hysterophorus weed in the rhizosphere of wheat.
A review of Roberts et al. (2022) on achievements and development of bioherbicides for weed control described mechanism of bioherbicides for weed control by bacteria, fungi, plant extracts, and viruses and the developed products from bacterial and fungal origin. Moreover, this review provides numerous advantages of bioherbicides use over traditional herbicide like bioherbicides are eco-friendly, less toxic than chemicals, can be used against herbicide-resistant weeds and are more host-specific in selected habitats. Along provision of the benefits authors imposed the need for ongoing research to focus on improving the product development and its commercialization, finding more effective products from bacterial, fungal, plant extracts, or viral origin and understanding their modes of action and determine conducive environmental conditions for successful weed control. The retardation in weed growth by plant allelopathy is the result of reduction in root-cell division, chlorophyll production and impairment, nutritional imbalance, and phytohormonal imbalance, in contrast increase in production of reactive oxygen species (ROS), stress-mediated hormones, and irregular antioxidant activities by allelochemicals. On the other hand, excessive secretions of secondary metabolites (hydrogen cyanide: HCN), lytic enzymes, and phytotoxins (elevated phytohormones; IAA) from microbes have ability to degrade the weed seed coat, which inhibits seed germination and may impair energy generating enzymes (cytochrome c oxidase) in root electron transport chain (ETC; Radhakrishnan et al. 2018).
Microbial allelopathy
Microbial allelopathy possesses several advantages compared with plant allelopathy including the production of allelochemicals at the targeted site (weed rhizosphere) and their ready absorption at the same time. The use of plant antagonistic microorganisms that produce allelochemicals near weed roots may be an option to survive with weed infestations by minimizing crop production losses. These microbes colonize root surface of target plants and have direct or indirect roles in growth suppression, reducing population densities, and reproductive capacity of weeds that help in reducing impacts of weeds on crop production (Kremer 2013).
Any formulation of fungal pathogens primarily for controlling weeds in the field is called mycoherbicide (Chakraborty and Ray 2021). Since the 1980s, several bioherbicides have been developed with fungi from the Phoma and Colletotrichum genera as active biocontrol agents (Harding and Raizada 2015). Among 2000 identified species of genus Phoma some were plant pathogens, while others were saprophytic residing in the soil and contributing to decomposition of dead plant tissues and some had phytotoxic metabolite production ability (Graupner et al. 2003). Potential candidates as mycoherbicides from fungi include Puccinia, Sclerotina, Alternaria, Chondrostereum, and Phytophthora genera (Harding and Raizada 2015). Wood and Morris (2007) studied the effect of biocontrol through gall-forming fungi and reported an 87%–98% reduction in weed population densities during 1991–2005. Carbohydrate-degrading enzymes, proteases enzyme and ferricrocin (siderophores) by Colletotrichum species were also reported for their phytotoxicity and weed control (Ohra et al. 1995, Jayasankar et al. 1999). Whereas members of genus Phoma produce putaminoxin, phomalairdenone, epoxydonesters, and nonenolides phytotoxins, which cause bleaching and chlorosis in broad-leaved weeds (Graupner et al. 2003, Zhou et al. 2004). Phoma chenopodicola, was investigated for controlling infestation of C. album through phytotoxin chenopodolin (diterpene) causing necrotic abrasions on leaves of C. album, Setaria viridis, Cirsium arvense, and Mercurialis annua (Cimmino et al. 2013a). The data regarding allelopathic fungi and their target weeds are described in Table 2.
Allelopathic fungi . | Target weed species . | Reported by . |
---|---|---|
Alternaria cassia | Senna obtusifolia (L.) H. S. Irwin and Barneby S. occidentalis (L.) Link Crotalaria spectabilis Roth | Charudattan et al. (1986), Boyette (1988) |
Alternaria destruens | Cuscuta. spp. | Simmons (1998) |
Alternaria helianthin | Xanthium strumarium L. | Abbas et al. (2004) |
Amphobotrys ricini | Euphorbiaceae | Holcomb et al. (1989) |
Cochliobolus lunatus | Echinochloa crus-galli (L.) P. Beauv. | Scheepens (1987) |
Colletotrichum coccodes, Fusarium lateritium | Abutilon theophrasti Medik. | Hodgson et al. (1988) |
Colletotrichum dematium | Leguminosae | Cardina et al. (1988) |
Colletotrichum gloesporioides | Leguminosae, Malvaceae, Convolvulaceae (C. spp.) | Mortensen and Makowski (1997) |
Colletotrichum graminicola | Gramineae | Hoagland et al. (2007) |
Colletotrichum orbiculare | X. spinosum | Auld et al. (1988) |
Colletotrichum truncatum | Sesbania exaltata (Raf.) Rydb. ex A.W.Hill | Boyette (1991) |
Exserohilum monoceras | Echinochloa spp. | Zhang and Watson (1997) |
Fusarium lateritium | Sida spinosa L. Anoda cristata (L.) Schltdl. Potamogeton spp. | Walker (1981), Bernhardt and Duniway (1986) |
Fusarium lateritium | Ambrosia trifida L. | Hoagland et al. (2007) |
Fusarium oxysporum | Phelipanche ramosa (L.) Pomel | Kohlschmid et al. (2009) |
Phoma macrostoma | T. officinale | Smith et al. (2015) |
Phoma herbarum | Taraxacum officinale (L.) Weber ex F.H. Wigg | Neumann and Boland (1999) |
Myrothecium verrucaria | S. obtusifolia Portulaca spp. Euphorbia spp. | Boyette et al. (2007) |
Phoma chenopodicola | C. album, C. arvense (L.) Scop., S. viridis (L.) P. Beauv., M. annua L. | Cimmino et al. (2013b) |
Phyllachora cyperi | Cyperus rotundus L. | Hoagland et al. (2007) |
Pyricularia sp. | Digitaria sanguinalis (L.) Scop. | Hoagland et al. (2007) |
Sclerotinia sclerotiorum | Multiple species | Brosten and Sands (1986) |
Sclerotinia minor | T. officinale, T. repens L., Plantago minor Garsault | Riddle et al. (1991) |
Allelopathic fungi . | Target weed species . | Reported by . |
---|---|---|
Alternaria cassia | Senna obtusifolia (L.) H. S. Irwin and Barneby S. occidentalis (L.) Link Crotalaria spectabilis Roth | Charudattan et al. (1986), Boyette (1988) |
Alternaria destruens | Cuscuta. spp. | Simmons (1998) |
Alternaria helianthin | Xanthium strumarium L. | Abbas et al. (2004) |
Amphobotrys ricini | Euphorbiaceae | Holcomb et al. (1989) |
Cochliobolus lunatus | Echinochloa crus-galli (L.) P. Beauv. | Scheepens (1987) |
Colletotrichum coccodes, Fusarium lateritium | Abutilon theophrasti Medik. | Hodgson et al. (1988) |
Colletotrichum dematium | Leguminosae | Cardina et al. (1988) |
Colletotrichum gloesporioides | Leguminosae, Malvaceae, Convolvulaceae (C. spp.) | Mortensen and Makowski (1997) |
Colletotrichum graminicola | Gramineae | Hoagland et al. (2007) |
Colletotrichum orbiculare | X. spinosum | Auld et al. (1988) |
Colletotrichum truncatum | Sesbania exaltata (Raf.) Rydb. ex A.W.Hill | Boyette (1991) |
Exserohilum monoceras | Echinochloa spp. | Zhang and Watson (1997) |
Fusarium lateritium | Sida spinosa L. Anoda cristata (L.) Schltdl. Potamogeton spp. | Walker (1981), Bernhardt and Duniway (1986) |
Fusarium lateritium | Ambrosia trifida L. | Hoagland et al. (2007) |
Fusarium oxysporum | Phelipanche ramosa (L.) Pomel | Kohlschmid et al. (2009) |
Phoma macrostoma | T. officinale | Smith et al. (2015) |
Phoma herbarum | Taraxacum officinale (L.) Weber ex F.H. Wigg | Neumann and Boland (1999) |
Myrothecium verrucaria | S. obtusifolia Portulaca spp. Euphorbia spp. | Boyette et al. (2007) |
Phoma chenopodicola | C. album, C. arvense (L.) Scop., S. viridis (L.) P. Beauv., M. annua L. | Cimmino et al. (2013b) |
Phyllachora cyperi | Cyperus rotundus L. | Hoagland et al. (2007) |
Pyricularia sp. | Digitaria sanguinalis (L.) Scop. | Hoagland et al. (2007) |
Sclerotinia sclerotiorum | Multiple species | Brosten and Sands (1986) |
Sclerotinia minor | T. officinale, T. repens L., Plantago minor Garsault | Riddle et al. (1991) |
Allelopathic fungi . | Target weed species . | Reported by . |
---|---|---|
Alternaria cassia | Senna obtusifolia (L.) H. S. Irwin and Barneby S. occidentalis (L.) Link Crotalaria spectabilis Roth | Charudattan et al. (1986), Boyette (1988) |
Alternaria destruens | Cuscuta. spp. | Simmons (1998) |
Alternaria helianthin | Xanthium strumarium L. | Abbas et al. (2004) |
Amphobotrys ricini | Euphorbiaceae | Holcomb et al. (1989) |
Cochliobolus lunatus | Echinochloa crus-galli (L.) P. Beauv. | Scheepens (1987) |
Colletotrichum coccodes, Fusarium lateritium | Abutilon theophrasti Medik. | Hodgson et al. (1988) |
Colletotrichum dematium | Leguminosae | Cardina et al. (1988) |
Colletotrichum gloesporioides | Leguminosae, Malvaceae, Convolvulaceae (C. spp.) | Mortensen and Makowski (1997) |
Colletotrichum graminicola | Gramineae | Hoagland et al. (2007) |
Colletotrichum orbiculare | X. spinosum | Auld et al. (1988) |
Colletotrichum truncatum | Sesbania exaltata (Raf.) Rydb. ex A.W.Hill | Boyette (1991) |
Exserohilum monoceras | Echinochloa spp. | Zhang and Watson (1997) |
Fusarium lateritium | Sida spinosa L. Anoda cristata (L.) Schltdl. Potamogeton spp. | Walker (1981), Bernhardt and Duniway (1986) |
Fusarium lateritium | Ambrosia trifida L. | Hoagland et al. (2007) |
Fusarium oxysporum | Phelipanche ramosa (L.) Pomel | Kohlschmid et al. (2009) |
Phoma macrostoma | T. officinale | Smith et al. (2015) |
Phoma herbarum | Taraxacum officinale (L.) Weber ex F.H. Wigg | Neumann and Boland (1999) |
Myrothecium verrucaria | S. obtusifolia Portulaca spp. Euphorbia spp. | Boyette et al. (2007) |
Phoma chenopodicola | C. album, C. arvense (L.) Scop., S. viridis (L.) P. Beauv., M. annua L. | Cimmino et al. (2013b) |
Phyllachora cyperi | Cyperus rotundus L. | Hoagland et al. (2007) |
Pyricularia sp. | Digitaria sanguinalis (L.) Scop. | Hoagland et al. (2007) |
Sclerotinia sclerotiorum | Multiple species | Brosten and Sands (1986) |
Sclerotinia minor | T. officinale, T. repens L., Plantago minor Garsault | Riddle et al. (1991) |
Allelopathic fungi . | Target weed species . | Reported by . |
---|---|---|
Alternaria cassia | Senna obtusifolia (L.) H. S. Irwin and Barneby S. occidentalis (L.) Link Crotalaria spectabilis Roth | Charudattan et al. (1986), Boyette (1988) |
Alternaria destruens | Cuscuta. spp. | Simmons (1998) |
Alternaria helianthin | Xanthium strumarium L. | Abbas et al. (2004) |
Amphobotrys ricini | Euphorbiaceae | Holcomb et al. (1989) |
Cochliobolus lunatus | Echinochloa crus-galli (L.) P. Beauv. | Scheepens (1987) |
Colletotrichum coccodes, Fusarium lateritium | Abutilon theophrasti Medik. | Hodgson et al. (1988) |
Colletotrichum dematium | Leguminosae | Cardina et al. (1988) |
Colletotrichum gloesporioides | Leguminosae, Malvaceae, Convolvulaceae (C. spp.) | Mortensen and Makowski (1997) |
Colletotrichum graminicola | Gramineae | Hoagland et al. (2007) |
Colletotrichum orbiculare | X. spinosum | Auld et al. (1988) |
Colletotrichum truncatum | Sesbania exaltata (Raf.) Rydb. ex A.W.Hill | Boyette (1991) |
Exserohilum monoceras | Echinochloa spp. | Zhang and Watson (1997) |
Fusarium lateritium | Sida spinosa L. Anoda cristata (L.) Schltdl. Potamogeton spp. | Walker (1981), Bernhardt and Duniway (1986) |
Fusarium lateritium | Ambrosia trifida L. | Hoagland et al. (2007) |
Fusarium oxysporum | Phelipanche ramosa (L.) Pomel | Kohlschmid et al. (2009) |
Phoma macrostoma | T. officinale | Smith et al. (2015) |
Phoma herbarum | Taraxacum officinale (L.) Weber ex F.H. Wigg | Neumann and Boland (1999) |
Myrothecium verrucaria | S. obtusifolia Portulaca spp. Euphorbia spp. | Boyette et al. (2007) |
Phoma chenopodicola | C. album, C. arvense (L.) Scop., S. viridis (L.) P. Beauv., M. annua L. | Cimmino et al. (2013b) |
Phyllachora cyperi | Cyperus rotundus L. | Hoagland et al. (2007) |
Pyricularia sp. | Digitaria sanguinalis (L.) Scop. | Hoagland et al. (2007) |
Sclerotinia sclerotiorum | Multiple species | Brosten and Sands (1986) |
Sclerotinia minor | T. officinale, T. repens L., Plantago minor Garsault | Riddle et al. (1991) |
Besides a potent biocontrol agent in controlling economically important weeds, fungi have several limitations because of their parasitic nature (Hershenhorn et al. 2016). Most of the fungus species belonging to Colletotrichum genera are plant pathogens which cause severe economic losses by reducing crop yields and deteriorate the quality of a wide range of crops through post-harvest damages (Münch et al. 2008). Anthracnose of stem leaves and fruits in fruit trees is another example of crop loss by Colletotrichum gloeosporioides (Jayasankar et al. 1999). Furthermore, Colletotrichum graminicola is responsible for stalk rot and anthracnose of maize (Münch et al. 2008). These limitations restricted the use of fungi for biocontrol of weeds due to their non-specificity and potential disease promotion in plants.
Allelopathic bacteria
The rhizobacteria capable of producing secondary metabolites in weed rhizosphere and suppressing its growth are called “Allelopathic Bacteria (AB)” (Suslow and Schroth 1982, Kremer 2006). Another term used to describe these bacteria is “deleterious rhizobacteria (DRB)” (Li and Kremer 2006). The success of microbial weed biocontrol lies in the proper distribution, bioavailable concentration and phytotoxicity of microbial metabolites towards target weeds (Abbas et al. 2018). AB interactions with crop plants may be neutral (O'Hara 2005), harmful (Weissmann et al. 2003) or beneficial (Li and Kremer 2006). AB possesses a novel property of host specificity which makes them a suitable candidate for use as biocontrol agent (Zeller et al. 2007). Biocontrol of weeds through bacterial agents possesses multiple advantages over fungal agents, like rapid growth, simple propagation requirements, and ease in genetic modification (Li et al. 2003, Harding and Raizada 2015). Furthermore, the allelopathic activities of rhizobacteria can continue throughout the cropping season limiting germination and growth of targeted weeds (Kremer 2006, Kennedy 2019). Development of bio-based herbicides comprising AB under natural conditions is an attractive and eco-friendly option for weed management in terrestrial ecosystem.
The genus Pseudomonas is a dominant biocontrol agent for weeds and other crop pathogens, including P. fluorescence, P. trivialis, P. aeruginosa, and P. putida (Flores-Vargas and O'Hara 2006, Mejri et al. 2013, Kennedy 2018, Abbas et al. 2017a, b). Other bacterial genera like Agrobacterium, Aeromonas, Enterobacter, Bacillus, Burkholderia, Chryseomonas, Xanthomonas, and Vibrio have also been reported to hinder germination and growth of certain weed plants, that is, A. retroflexus, C. arvensis, Ipomoea spp., Setaria faberi, and X. strumarium (Li and Kremer 2006; Radhakrishnan et al. 2018). Unfortunately, only one bacterial based herbicide was registered in 20th century under brand name Camparico in 1997, and the second named as D7® was registered by US Environmental Protection Agency during 2014 against downy brome, which contained Pseudomonas fluorescens (Kennedy 2018) but could not reach the commercial production stage (Trognitz et al. 2016). A few examples of allelopathic bacteria as biocontrol agents and targeted weeds are described in Table 3.
Allelopathic bacterial strain . | Target weed species . | Reported by . |
---|---|---|
Pseudomonas fluorescens strain B11 Pseudomonas fulva strain T19 Pseudomonas thivervalensis strain T24 Pseudomonas fulva strain T75 | Avena fatua, P. minor, and R. dentatus | Dar et al. (2020), (2022) |
Pseudomonas fluorescens strain D7 | Downy brome (Bromus tectorum) Jointed goatgrass (Aegilops cylindrica) | Kennedy et al. (1991); Kennedy (2016); Kennedy (2018) |
Pseudomonas fluorescens strain BRG100 | Green foxtail (S. viridis) | Quail et al. (2002) |
Pseudomonas fluorescens strain WH6 | Most of the weeds | Banowetz et al. (2008) |
Pseudomonas putida KT2440 Pseudomonas fluorescens F113 | Wild oat (A. fatua), little seed canary grass (P. minor), and broad-leaved dock (R. dentatus) | Abbas et al. (2017a) |
Pseudomonas fluorescens SBW25 | ||
Pseudomonas aeruginosa PAO1 | ||
Pseudomonas alcaligenes NBRIC14159 | ||
Pseudomonas fluorescens G2-11 | Barnyard grass (Echinochloa spp.), Green foxtail (S. viridis), ivy leaf (Hedera spp.) morning-glory (Ipomoea purpurea), and field bindweed (C. arvensis) | Li and Kremer (2006) |
Pseudomonas syringae | Lettuce (Lactuca sativa), barnyard grass (Echinochloa spp.) | Kremer and Souissi (2001) |
Pseudomonas trivialis X33d | Downy brome (B. tectorum) | Mejri et al. (2010) |
Pseudomonas putida P. aeruginosa P. alcaligenes | Purple nut sedge (C. rotundus) and jungle rice (Echinochloa colonum) | Abbas et al. (2020b) |
Pseudomonas sp. (PS) Pseudomonas sp. (PS15) Bacillus sp. (BS21) Xanthomonas sp. (BS47) | Field bindweed (C. arvensis) and little hogweed (Portulaca oleracea) | Tawfik et al. (2019) |
Flavimonas oryzihabitans | Lettuce (L. sativa), barnyard grass (Echinochloa spp.) | Kremer and Souissi (2001) |
Flavobacterium balusitinum | Leafy spurge (Euphorbia esula) | Souissi et al. (1997) |
Xanthomonas campestris pv. poae | Annual blue grass (P. annua) | Imaizumi et al. (1997) |
Xanthomonas campestris LVA- 987 | Horseweed (Conyza canadensis) | Boyette and Hoagland (2015) |
Alcaligenes xylosoxidans | Wild radish (R. raphanistrum) | Flores‐Vargas and O'Hara (2006) |
Acidovorax delafieldii | Velvetleaf (A. theophrasti) | Owen and Zdor (2001) |
Allelopathic bacterial strain . | Target weed species . | Reported by . |
---|---|---|
Pseudomonas fluorescens strain B11 Pseudomonas fulva strain T19 Pseudomonas thivervalensis strain T24 Pseudomonas fulva strain T75 | Avena fatua, P. minor, and R. dentatus | Dar et al. (2020), (2022) |
Pseudomonas fluorescens strain D7 | Downy brome (Bromus tectorum) Jointed goatgrass (Aegilops cylindrica) | Kennedy et al. (1991); Kennedy (2016); Kennedy (2018) |
Pseudomonas fluorescens strain BRG100 | Green foxtail (S. viridis) | Quail et al. (2002) |
Pseudomonas fluorescens strain WH6 | Most of the weeds | Banowetz et al. (2008) |
Pseudomonas putida KT2440 Pseudomonas fluorescens F113 | Wild oat (A. fatua), little seed canary grass (P. minor), and broad-leaved dock (R. dentatus) | Abbas et al. (2017a) |
Pseudomonas fluorescens SBW25 | ||
Pseudomonas aeruginosa PAO1 | ||
Pseudomonas alcaligenes NBRIC14159 | ||
Pseudomonas fluorescens G2-11 | Barnyard grass (Echinochloa spp.), Green foxtail (S. viridis), ivy leaf (Hedera spp.) morning-glory (Ipomoea purpurea), and field bindweed (C. arvensis) | Li and Kremer (2006) |
Pseudomonas syringae | Lettuce (Lactuca sativa), barnyard grass (Echinochloa spp.) | Kremer and Souissi (2001) |
Pseudomonas trivialis X33d | Downy brome (B. tectorum) | Mejri et al. (2010) |
Pseudomonas putida P. aeruginosa P. alcaligenes | Purple nut sedge (C. rotundus) and jungle rice (Echinochloa colonum) | Abbas et al. (2020b) |
Pseudomonas sp. (PS) Pseudomonas sp. (PS15) Bacillus sp. (BS21) Xanthomonas sp. (BS47) | Field bindweed (C. arvensis) and little hogweed (Portulaca oleracea) | Tawfik et al. (2019) |
Flavimonas oryzihabitans | Lettuce (L. sativa), barnyard grass (Echinochloa spp.) | Kremer and Souissi (2001) |
Flavobacterium balusitinum | Leafy spurge (Euphorbia esula) | Souissi et al. (1997) |
Xanthomonas campestris pv. poae | Annual blue grass (P. annua) | Imaizumi et al. (1997) |
Xanthomonas campestris LVA- 987 | Horseweed (Conyza canadensis) | Boyette and Hoagland (2015) |
Alcaligenes xylosoxidans | Wild radish (R. raphanistrum) | Flores‐Vargas and O'Hara (2006) |
Acidovorax delafieldii | Velvetleaf (A. theophrasti) | Owen and Zdor (2001) |
Allelopathic bacterial strain . | Target weed species . | Reported by . |
---|---|---|
Pseudomonas fluorescens strain B11 Pseudomonas fulva strain T19 Pseudomonas thivervalensis strain T24 Pseudomonas fulva strain T75 | Avena fatua, P. minor, and R. dentatus | Dar et al. (2020), (2022) |
Pseudomonas fluorescens strain D7 | Downy brome (Bromus tectorum) Jointed goatgrass (Aegilops cylindrica) | Kennedy et al. (1991); Kennedy (2016); Kennedy (2018) |
Pseudomonas fluorescens strain BRG100 | Green foxtail (S. viridis) | Quail et al. (2002) |
Pseudomonas fluorescens strain WH6 | Most of the weeds | Banowetz et al. (2008) |
Pseudomonas putida KT2440 Pseudomonas fluorescens F113 | Wild oat (A. fatua), little seed canary grass (P. minor), and broad-leaved dock (R. dentatus) | Abbas et al. (2017a) |
Pseudomonas fluorescens SBW25 | ||
Pseudomonas aeruginosa PAO1 | ||
Pseudomonas alcaligenes NBRIC14159 | ||
Pseudomonas fluorescens G2-11 | Barnyard grass (Echinochloa spp.), Green foxtail (S. viridis), ivy leaf (Hedera spp.) morning-glory (Ipomoea purpurea), and field bindweed (C. arvensis) | Li and Kremer (2006) |
Pseudomonas syringae | Lettuce (Lactuca sativa), barnyard grass (Echinochloa spp.) | Kremer and Souissi (2001) |
Pseudomonas trivialis X33d | Downy brome (B. tectorum) | Mejri et al. (2010) |
Pseudomonas putida P. aeruginosa P. alcaligenes | Purple nut sedge (C. rotundus) and jungle rice (Echinochloa colonum) | Abbas et al. (2020b) |
Pseudomonas sp. (PS) Pseudomonas sp. (PS15) Bacillus sp. (BS21) Xanthomonas sp. (BS47) | Field bindweed (C. arvensis) and little hogweed (Portulaca oleracea) | Tawfik et al. (2019) |
Flavimonas oryzihabitans | Lettuce (L. sativa), barnyard grass (Echinochloa spp.) | Kremer and Souissi (2001) |
Flavobacterium balusitinum | Leafy spurge (Euphorbia esula) | Souissi et al. (1997) |
Xanthomonas campestris pv. poae | Annual blue grass (P. annua) | Imaizumi et al. (1997) |
Xanthomonas campestris LVA- 987 | Horseweed (Conyza canadensis) | Boyette and Hoagland (2015) |
Alcaligenes xylosoxidans | Wild radish (R. raphanistrum) | Flores‐Vargas and O'Hara (2006) |
Acidovorax delafieldii | Velvetleaf (A. theophrasti) | Owen and Zdor (2001) |
Allelopathic bacterial strain . | Target weed species . | Reported by . |
---|---|---|
Pseudomonas fluorescens strain B11 Pseudomonas fulva strain T19 Pseudomonas thivervalensis strain T24 Pseudomonas fulva strain T75 | Avena fatua, P. minor, and R. dentatus | Dar et al. (2020), (2022) |
Pseudomonas fluorescens strain D7 | Downy brome (Bromus tectorum) Jointed goatgrass (Aegilops cylindrica) | Kennedy et al. (1991); Kennedy (2016); Kennedy (2018) |
Pseudomonas fluorescens strain BRG100 | Green foxtail (S. viridis) | Quail et al. (2002) |
Pseudomonas fluorescens strain WH6 | Most of the weeds | Banowetz et al. (2008) |
Pseudomonas putida KT2440 Pseudomonas fluorescens F113 | Wild oat (A. fatua), little seed canary grass (P. minor), and broad-leaved dock (R. dentatus) | Abbas et al. (2017a) |
Pseudomonas fluorescens SBW25 | ||
Pseudomonas aeruginosa PAO1 | ||
Pseudomonas alcaligenes NBRIC14159 | ||
Pseudomonas fluorescens G2-11 | Barnyard grass (Echinochloa spp.), Green foxtail (S. viridis), ivy leaf (Hedera spp.) morning-glory (Ipomoea purpurea), and field bindweed (C. arvensis) | Li and Kremer (2006) |
Pseudomonas syringae | Lettuce (Lactuca sativa), barnyard grass (Echinochloa spp.) | Kremer and Souissi (2001) |
Pseudomonas trivialis X33d | Downy brome (B. tectorum) | Mejri et al. (2010) |
Pseudomonas putida P. aeruginosa P. alcaligenes | Purple nut sedge (C. rotundus) and jungle rice (Echinochloa colonum) | Abbas et al. (2020b) |
Pseudomonas sp. (PS) Pseudomonas sp. (PS15) Bacillus sp. (BS21) Xanthomonas sp. (BS47) | Field bindweed (C. arvensis) and little hogweed (Portulaca oleracea) | Tawfik et al. (2019) |
Flavimonas oryzihabitans | Lettuce (L. sativa), barnyard grass (Echinochloa spp.) | Kremer and Souissi (2001) |
Flavobacterium balusitinum | Leafy spurge (Euphorbia esula) | Souissi et al. (1997) |
Xanthomonas campestris pv. poae | Annual blue grass (P. annua) | Imaizumi et al. (1997) |
Xanthomonas campestris LVA- 987 | Horseweed (Conyza canadensis) | Boyette and Hoagland (2015) |
Alcaligenes xylosoxidans | Wild radish (R. raphanistrum) | Flores‐Vargas and O'Hara (2006) |
Acidovorax delafieldii | Velvetleaf (A. theophrasti) | Owen and Zdor (2001) |
Modes of action of allelopathic bacteria
The allelopathic bacteria adopt multiple strategies to inhibit germination and suppress growth and development of weeds (Fig. 2). The details are described below:
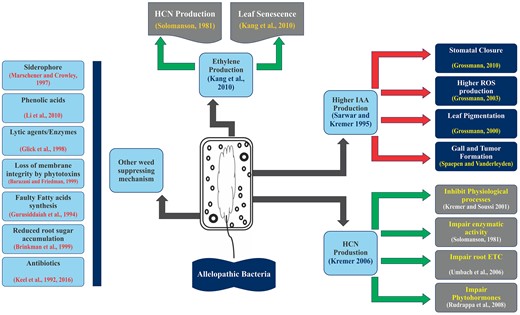
The possible modes of action for weed suppression by allelopathic bacteria. *ETC, ROS, HCN.
Cyanide production
Many researchers have reported the production of secondary metabolites by allelopathic bacteria (Abbas et al. 2017a, Mustafa et al. 2019, Rasool et al. 2019). Kremer (2006) reported the reduction of weed infestation by HCN producing bacteria in the rhizosphere of the velvetleaf and downy broom. The HCN production by bacteria and its effects on weeds are represented in Fig. 3. Glycine and methionine are the major precursors of cyanide (Gallagher and Manoil 2001, Dar et al. 2022). The membrane bound HCN synthase triggered by hcnABC gene is the driving force behind cyanogenesis through oxidative decarboxylation of glycine, the cyanide precursor (Blumer and Haas 2000, Zdor 2015). HCN is also released as a by-product in ethylene biosynthesis in plants through metabolizing 1-aminocyclopropane-1-carboxylic acid (ACC) by ACC synthase which further enhances the phytotoxicity of cyanide in the target plant (Grossmann 2003, 2010). Owen and Zdor (2001) studied the effectiveness of a Pseudomonas spp. for growth suppression in velvetleaf (A. theophrasti) by application of supplemental glycine and found 30%–50% reduction in shoot length than non-amended glycine control due to excessive production of HCN. Its production in the rhizosphere not only depends upon its precursor amino acids (glycine, methionine) but also on pH (7.3–7.8), iron as co-factor and inorganic phosphate (Schippers et al. 1990). Microbially produced cyanide usually present in the form of HCN, volatilizes in natural environments, and negatively affects root growth and development.
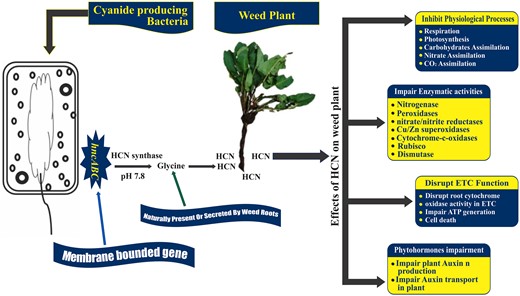
HCN production by Cyanide producing bacteria and its effects on weed growth. *ETC, ATP = Adenosine Triphosphate, HCN, Rubisco = Ribulose-1,5-bisphosphate carboxylase-oxygenase.
Pseudomonas aeruginosa and P. fluorescens are commonly reported as cyanide producers in various concentrations, usually lower than 1 mM (Blumer and Haas 2000). HCN inhibits different physiological processes in plants, that is, respiration, carbohydrates metabolism, nitrate, and CO2 assimilation, by impairing enzymes involved in these vital processes. The important enzymes sensitive to cyanide concentrations involve nitrogenase, nitrate/nitrite reductase, peroxidase, Cu/Zn-superoxidase, dismutase, cytochrome-c-oxidases, and ribulose-bisphosphate carboxylase (Solomanson 1981). Cyanide is also involved in reducing photosynthetic activity by interacting with Cu protein plastocyanin in the ETC (Kremer and Souissi 2001). HCN impairs root metabolism by limiting root cytochrome oxidases in the ETC essential for production of ATP in root cells, eventually leading towards cell death (Bakker and Schippers 1987, Umbach et al. 2006). Microbial HCN has been reported as a limiting agent for auxin production in plants and its transport by inhibiting primary root growth and development in green foxtail, lettuce, Arabidopsis thaliana and barnyard grass (E. crus-galli) (Rudrappa et al. 2008). In another study, more than two thousand isolates were analyzed for quantitative HCN production and only 32% isolates were found positive in cyanide production varying from trace to more than 30 nmoles of HCN/mg of cellular protein. Afterwards, eight isolates caused significant reduction in root lengths of barnyard grass by 15%–57% and lettuce by 35%–84% (Kremer and Souissi 2001).
Phytohormonal imbalance
Another aspect of weed biocontrol by bacteria is the higher production of phytohormones. Phytohormones at low concentrations are involved in vital physiological processes of plants and act as growth hormones, whereas higher concentrations turn their growth promotion activity to herbicidal activity/autotoxicity (Grossmann 2010, Kennedy 2019, Abbas et al. 2020a, c). It has been reported that up to 80% of rhizobacteria have ability to synthesize indole-3-acetic acid (IAA), including free living bacteria belonging to Azospirillum spp., Enterobacter cloacae, Alkaligenes faecalis, Acetobacter dizotrophicous, Xanthomonas spp., and Pseudomonas spp. at very low concentrations (Vessey 2003). Along with concentration, the negative effects are also dependent upon age, growth phase and plant species. Sarwar and Kremer (1995) suggested that the growth reduction of field bindweed (90.5%) by Enterobacter taylorae was due to the excessive production of auxin (IAA: 72 mg/L) which can be further enhanced by amending the culture with L-tryptophan. The first response of elevated levels of IAA-triggered biosynthesis of ACC synthase for inducing ethylene stress in shoots and abscisic acid accumulation. Later, root and shoot growth inhibition, reduced internode elongation, yellowish green pigmented leaves, stomatal closure, and enhanced formation of ROS are the main physiological disorders of elevated auxin levels (Grossmann 2000, 2003, 2010). Spaepen and Vanderleyden (2011) reported gall and tumor formation in response to excessive auxin production by Agrobacterium spp. and Pseudomonas savastanoi. In another study, elevated auxin levels by Enterobactor strain I-3 reduced biomass production in radish and lettuce through increasing endogenous IAA (Park et al. 2015).
Excessive production of other hormones is also lethal for plants (Kennedy et al. 1991, Nehl et al. 1997). Ethylene plays critical roles in root proliferation, inhibiting cell division, enhancing fruit ripening, leaf abscission, lower leaves wilting, and triggering synthesis of other phytohormones (Kang et al. 2010). Endogenous ethylene production is triggered by ACC synthase enzyme in the presence of ACC after reproductive stage or at fruit ripening stage (Glick et al. 2007). Oxidation of ACC by ACC-synthase leads to the synthesis of ethylene, CO2, and HCN. HCN is a potent toxin to reduce plant growth by impairing vital physiological functions (Solomanson 1981, Grossmann 2010). Furthermore, microbial production of gibberellins and cytokinin at higher concentrations also have inhibitory effect on plant growth (Gupta et al. 2015).
Other growth inhibitory substances
Microorganisms play crucial roles in balancing agro-ecosystem. They face diverse environmental conditions that affect survival in soil. While competing with microflora and fauna, they secrete a wide range of chemicals inhibitory to plants, that is, phytotoxins, exopolysaccharides, phenolic acids, siderophores, lytic enzymes, and antibiotics classically known as secondary metabolites. Phytotoxin production is a key characteristic of Pseudomonas species (Marschener and Crowley 1997, Shah et al. 1998, Bender et al. 1999, Caldwell et al. 2012). Among them, P. fluorescence was studied for phytotoxin production and impairing membrane permeability of root cells (Barazani and Friedman 1999). Tranel et al. (1993) tested P. fluorescence D7 strain for downy brome suppression and evaluated phytotoxins produced by D7 strain, which were helpful in root growth reduction. Furthermore, this root growth reduction was attributed to a metabolite complex including fatty acids, esters, peptides, and chromopeptides (Gurusiddaiah et al. 1994).
Brinkman et al. (1999) demonstrated reduced sugar accumulation in leafy spurge as a weed suppressing effect by rhizobacteria, whereas Kremer (2006) demonstrated the root growth reduction as a function of cell wall and membrane degradation by lytic enzymes. Coronatine, ferulic acids, syringopeptin, colletotrichins derivatives, and ketopiperazine were extracted from the phytotoxin profiling of two P. syringae and one Colletotrichum sp. responsible for growth reduction of Phalaris paradox, Polyogon monspeliensis, and C. arvensis (Omer et al. 2010). Phytotoxins (2-aminophenoxazone, phenazine-1-carboxylic acid and 2-aminophenol) extracted form P. syringae strain 3366 caused downy brome suppression (Gealy et al. 1996). Similar antibiotics were also extracted from P. fluorescens effective for downy brome control. Moreover, 2,4-diacetylphloroglucinol (broad-spectrum antibiotic) released by P. fluorescens strain CHA0 was toxic towards many crop plants (Kennedy 2016). Germination arrest factor was one or a complex of P. fluorescens WH6 secreted phytotoxins reducing germination of grassy weeds (Banowetz et al. 2008, 2009). Furthermore, extracellular secretions of P. fluorescens BRG100 (pseudophomin A and B along with lipodepsipeptides) were responsible for suppressing growth and development of green foxtail (Quail et al. 2002).
Microbial allelopathy and growth of infested crop
Weed suppressive allelopathic bacteria may promote growth of crops due to coincident plant growth promoting characteristics. The growth promoting characteristics include mineral solubilization especially phosphorus, hormone synthesis especially auxins, chitinases, siderophore production, induction of the systemic resistance, and stress tolerance through production of ACC-deaminase that reduces stress-induced ethylene levels in the rhizosphere of the crop (Atieno et al. 2012, Gupta et al. 2015). So, these allelopathic bacteria can be used as suitable candidates in crop production. Dual functionality of these bacteria ensures no harm to the non-target species/crop plants. Most of the scientific community agreed upon this phenomenon due to differential colonization ability of AB in crop and weed rhizosphere and AB interactions with rhizospheric exudates of the plant (Kennedy et al. 2001, Abbas et al. 2018, Kremer 2019, 2021). Selective toxicity of allelopathic bacterial metabolites might be another reason, which is ascribed to less rhizosphere colonization, change in behavior from toxicity to growth promoting and selective suppression ability of phytotoxins in the non-target plant rhizosphere alone or in combination (Owen and Zdor 2001).
Zhu and his colleagues (2020) demonstrated the host specificity of microbial biocontrol agents as they test the weeds and crop infection by Trichoderma polysporum strain HZ-31 and found that the tested strain caused severe infection on A. fatua, Egeria densa, Polygonum aviculare, Polygonum lapathifolium, C. album, and Lepyrodiclis holosteoides fresh leaves without infecting Pisum sativum and Vicia faba. Similarly, Kennedy (2019) also advocated safe and effective weed control through deleterious rhizobacteria. Begonia and Kremer (1994), and Zeller et al. (2007) demonstrated the host selectiveness as a function of presence or absence of phytotoxin precursors and reduced amount of precursor's availability in non-target plant rhizosphere required by AB to perform their suppressive activities. The outcomes due to these interactions may be beneficial or harmful for the non-target plants (Zdor et al. 2005).
Application of P. putida and Acidovorax delafieldii for suppression of A. theophrasti infestation in corn grown under field revealed less cyanide (1 and 14 mmoles/g of root biomass) production in corn rhizosphere than 53 and 68 mmoles of cyanide production/g of root biomass in A. theophrasti rhizosphere regardless of same colonization ability in both plant roots (Gurley and Zdor 2005). Similar biocontrol strains were also tested by Owen and Zdor (2001) and concluded that HCN production in corn rhizosphere is doubled than A. theophrasti rhizosphere but caused 40%–80% suppression due to the biomass differences of both plants. These results witnessed the concept of differential toxicity of the metabolites and differential sensitivity of crop plants, also supported by Piotrowska-Seget (1995). Kennedy and Stubbs in (2007), screened 2450 strains isolated from wheat against jointed goat grass (A. cylindrica) and green foxtail (S. viridis) and found 76 strains, which were suppressing these weeds but did not suppress wheat seedlings which further strengthened the idea of selective suppression by allelopathic bacteria. This selective suppression and possession of growth promotion activities by AB provides an opportunity for development and commercialization of bioherbicides containing these novel bacteria or their metabolites for sustainable weed control.
Conclusion and future directions
Besides reducing weed population densities, the primary focus of sustainable weed control strategies is to enhance crop production in economic terms and reduce damaging effects of these pests (weeds) on crops, animals, humans, and the ecosystem. The conventional approaches are expensive, labor intensive, and damage the environment by causing resistance in weeds and pollution of air, water, and soil, damaging the ecosystem microbiome, and are deleterious to human health. On the other hand, the advanced/biological means (plant and microbial allelopathy) of weed control are more ecofriendly and sustainable alternate of chemical herbicides. The selective and differential phytotoxicity of allelopathic bacteria provides us the opportunity for its adoption as sustainable weed control approach. The shift from chemical to biological weed control not only reduces weed population densities but also reduces the detrimental effects of herbicidal residues on the environment and food production. Therefore, future research should focus on:
Integration of bacterial allelopathy with reduced dose of chemical herbicide for sustainable crop production.
Chemical identification of microbial based phytotoxins and their effect on weed and crop physiology along with their impacts on microbial community structure.
Potential of integrating allelopathic bacteria into current biocontrol methods (plant allelopathy) for further diversification and effective control of weeds.
Development of a broad-spectrum bioherbicide as an integrated weed management technology.
Author contributions
Abubakar Dar (Investigation, Methodology, Writing – original draft), Zahir Ahmad Zahir (Conceptualization, Resources, Supervision, Writing – review & editing), Maqshoof Ahmad (Formal analysis, Software, Validation), Azhar Hussain (Data curation, Software, Validation), Muhammad Tauseef Jaffar (Formal analysis, Investigation, Writing – original draft), and Robert Jhon Kremer (Conceptualization, Visualization, Writing – review & editing).
Conflict of interest
All the authors hereby declare that there is no conflict either personal or financial with any person or funding firm.
Data availability
Not applicable to this review