-
PDF
- Split View
-
Views
-
Cite
Cite
Pablo Quirós, Laura Sala-Comorera, Clara Gómez-Gómez, María Dolores Ramos-Barbero, Lorena Rodríguez-Rubio, Gloria Vique, Tula Yance-Chávez, Sergio Atarés, Sandra García-Gutierrez, Sonia García-Marco, Antonio Vallejo, Ignasi Salaet, Maite Muniesa, Identification of a virulent phage infecting species of Nitrosomonas, The ISME Journal, Volume 17, Issue 5, May 2023, Pages 645–648, https://doi.org/10.1038/s41396-023-01380-6
- Share Icon Share
Abstract
In the first and limiting step of nitrification, ammonia (NH3) is oxidised to nitrite (NO2−) by the action of some prokaryotes, including bacteria of the Nitrosomonas genus. A potential approach to nitrification inhibition would be through the application of phages, but until now this method has been unexplored and no virulent phages that infect nitrifying bacteria have been described. In this study, we report the isolation of the first phage infecting some Nitrosomonas species. This polyvalent virulent phage (named ΦNF-1) infected Nitrosomonas europaea, Nitrosomonas communis, and Nitrosomonas nitrosa. Phage ΦNF-1 has the morphology of the Podoviridae family, a dsDNA genome of 41,596 bp and a 45.1 % GC content, with 50 predicted open reading frames. Phage ΦNF-1 was found to inhibit bacterial growth and reduce NH4+ consumption in the phage-treated cultures. The application of phages as biocontrol agents could be a useful strategy for nitrification inhibition without the restrictions associated with chemical inhibitors.
Introduction
In the nitrogen (N) cycle, nitrification can be mediated by the activity of canonical ammonia-oxidizing bacteria (AOB) and archaea (AOA), which oxidize ammonia (NH3) to nitrite (NO2-), and then nitrate (NO3-) is produced by nitrite-oxidizing bacteria (NOB). Additionally, some members of the genus Nitrospira perform complete NH3 oxidation to NO3- (comammox) in a single step [1]. Among the AOB, Nitrosomonas, Nitrosospira, and Nitrosococcus are the most relevant genera [2, 3].
Nitrification occurs in soils, sediments, and aquatic environments. It plays an important role in wastewater treatment systems by contributing to excess N removal, and in agriculture, where it determines the availability of fertilizer N, required for a high plant productivity [4]. In some cases, the transformation of NH3 by ammonia-oxidizers before uptake by plants renders N fertilization inefficient and encourages the addition of excess fertilizer. This has deleterious ecological effects through the volatilization of NH3, production of N2O [5], or N leaching to water bodies [4, 6].
To improve the efficiency of N fertilization, a novel approach would be the application of phages that act against nitrifying prokaryotes. Bacteriophages (phages) have been proposed as biocontrol tools because they infect and lyse bacterial cells and can be applied in different fields [7–11] with a minimum impact on the microbial ecology of each biome. This study presents the first description of a phage that infects representatives of the genus Nitrosomonas and has potential application to suppress bacterial nitrifying activity.
Results and discussion
Cultures of Nitrosomonas europaea, Nitrosomonas communis, and Nitrosomonas nitrosa, selected for their high abundance in wastewater [2], were grown at 28 °C. As the slow-growing Nitrosomonas do not generate sufficient bacterial mass to monitor their growth spectrophotometrically, pH variations of bacterial cultures and qPCR were used instead. After 25 days in the dark (the time required to reach exponential growth), the cultures had a pH of 8.0 and were inoculated (day 0 of infection) with the phages purified from six wastewater samples collected in 2019 from four urban wastewater treatment plants in Catalonia (NE Spain) (supplementary online material). At day 4 of infection, the pH was readjusted to 8 (Fig. S1-A) to avoid bacterial growth inhibition by pH reduction and the cultures were incubated until day 7.
Control cultures exhibited a pH decrease, possibly due to bacterial growth, while the pH of phage-infected cultures remained steady and close to 8.0, particularly in those cultures infected with phage suspensions #1, #2, #4, and #5, which was attributed to phage-induced lysis of bacteria (Fig. S1-A).
As AOB do not form confluent growth on agar plates and plaques of lysis could not be generated, phages from the four suspensions were used to re-infect new cultures in five successive rounds, selecting the phage most successful in infecting each host strain. Suspension #1 (from which phage ΦNF-1 was isolated) exhibited the highest infectivity in all host strains. While the pH of the uninfected control cultures decreased 1.8–2.0 units, the pH of ΦNF-1-infected cultures remained constant for seven days (p > 0.05) (Fig. S1B-D).
The propagation of ΦNF-1 was confirmed by qPCR, which revealed an increase in phage gene copies. Accordingly, a decrease in Nitrosomonas amoB confirmed a reduction in the number of bacterial cells, presumably due to phage-induced lysis, as this was not observed in the phage-free control (Fig. 1, A–C).
Growth of Nitrosomonas cultures in the presence of phage ΦNF-1 and the effect of the phage on the NH4+ uptake in the cultures.
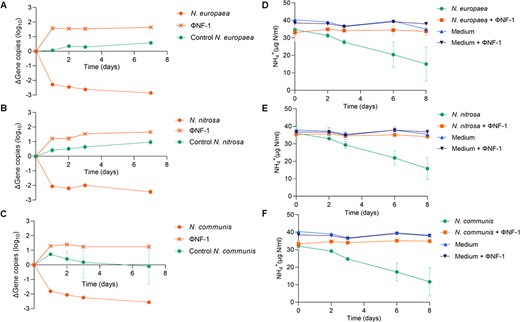
The growth of cultures in the presence of Φ NF-1 was monitored by the variation in the number of amoB gene copies in N. europaea (A), N. nitrosa (B), and N. communis (C) (orange circle). ΦNF-1 propagation was monitored by the increase in the number of copies of a non-coding fragment of the ΦNF-1 genome (orange cross). The control culture contained only bacteria (green). The NH4+ in cultures of the three strains of N. europaea (D), N. nitrosa (E), and N. communis (F) was measured in the presence (orange) or absence (green) of ΦNF-1. Controls included ΦNF-1 alone with AOB medium (black) and sterile AOB medium (blue). Results are the average of three to five independent experiments.
With the aim of evaluating the effect of ΦNF-1 phage on the host bacteria, NH3 oxidation in the cultures in the absence/presence of the phage was measured using the salicylate method [12, 13]. In the three phage-free bacterial cultures the NH4+ levels decreased by an average of 18–20 µg N/ml. In contrast, a significant (p < 0.05) lower decrease of NH4+ was observed in the three cultures infected with ΦNF-1, similar to that of the bacteria-free controls (Medium and Medium + ΦNF-1) (Fig. 1, D–F).
Phage ΦNF-1 and suspensions #2, 4, and 5 were evaluated by electron microscopy (supplementary online material). All showed icosahedral capsids of 50 ± 3 nm in diameter and very small tails typical of Podoviridae phages [14] (Fig. 2).
Morphological and genetic characterization of phage ΦNF-1.
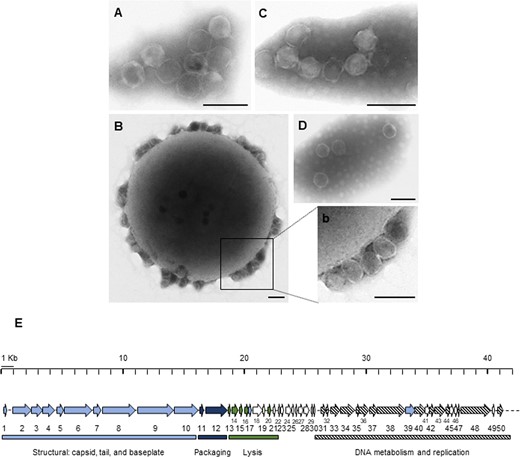
Electron micrographs of phage particles in phage suspensions: ΦNF-1 (A) and suspension #2 (B) infecting N. europaea. In (B) N. europaea cell can be seen with phage ΦNF-1 attached to the surface. In (b) a 2.5X amplification shows phage capsids in detail. C Suspension #4 infecting N. communis, and (D) suspension #5 infecting N. nitrosa. Bar = 100 nm. (E) Genetic map of phage ΦNF-1. Each arrow corresponds to an open reading frame (ORF) drawn in scale considering the total phage genome size of 41,596 bp. White arrows correspond to unidentified ORFs. Annotated ORFs have been assigned to a function within the phage genome (structural, packaging, lysis or phage replication).
A single phage genome was assembled from each suspension. Homology analysis between the four phages revealed a shared bp identity of 98.6–99.9 % (Fig. S2) and the 5 % of nucleotide variations involved replacements by similar amino acid residues (polar or basic), suggesting that all suspensions contained the same phage.
Phage ΦNF-1 (GenBank accession number OL634959) has a dsDNA genome of 41,596 bp and 45.1 % GC content, with 50 predicted open reading frames (ORFs) organized into functional modules of head-tail morphogenesis, packaging, lysis, metabolism, and replication (Fig. 2E). The closest sequence found in the databases belonged to a phage infecting Sphaerotilus natans previously assigned to the Podoviridae family (MN844877.1). Nevertheless, ΦNF-1 and Sphaerotilus phages are very different, with an average aminoacid identity of only 44.1 % and an average nucleotide identity of 60.7 %, and only 7.4 % of nucleotide alignment between both genomes (Fig. S3A). According to the ICTV (https://ictv.global/), this indicates they may not even belong to the same genus. As ΦNF-1 possesses its own DNA-dependent RNA polymerase, it has certain similarities with the Autographivirinae subfamily, which includes the auto-graphein or self-transcribing phages [14]. This is the case of Ralstonia phages, which share some similar ORF with ΦNF-1 and belong to the Autographivirinae. Genome-wide proteomic comparisons with characterized phage genomes showed that ΦNF-1 is distinct with low similarity to those infecting strains of E. coli, Burkholderia, Ralstonia, Pseudomonas, and Sphaerotiulus (Fig. S3B). Other tools used (supplementary information) to search for similarities between ΦNF-1 with other AOB phages or prophages revealed no homologies either at the protein or nucleotide level.
Phage ΦNF-1 is apparently not temperate, as genes related to lysogeny (i.e. integrase, excisionase, lysogenic module genes) were absent. In contrast, the high number of genes related to metabolism and replication indicates a phage with a considerable replicative potential, characteristic of virulent phages. The use of virulent rather than temperate phages is recommended for antimicrobial applications as the latter are less efficient at lysing the host and more likely to contribute to undesired horizontal gene transfer [10, 11]. Therefore, the virulence of ΦNF-1 is advantageous for its potential application against Nitrosomonas.
Based on our in vitro analysis evaluated with metabolically active bacterial cells grown in culture media, the maximum titer obtained for ΦNF-1 was estimated as 108 phages/ml, according to TEM observations and confirmed by qPCR, which detected the phage until dilution 10−8 (Fig. S4). Infection was not observed for dilutions beyond 10−6 (ca 100 phage/ml), indicating that below this concentration there are insufficient infectious particles to guarantee lysis.
To our knowledge, ΦNF-1 is the first Nitrosomonas-infecting virulent phage to be isolated, infecting at least three species of the Nitrosomonas genus, although temperate phages have previously been described in Nitrosospira genus [15], and infecting AOA [16].
Chemical inhibitors of nitrifying bacteria are being used to improve the efficiency of N fertilization in agriculture, but their effects on the environment and human health are still not well established. Phages could represent a more environmentally friendly alternative [17–19], as they are generally considered to be safe [20], auto-replicative, relatively specific for their hosts, and unable to propagate in their absence [21]. Further research on phages such as ΦNF-1 as a novel tool for nitrification inhibition is warranted, as this approach has the potential to enhance fertilization efficiency without harming the environment.
Acknowledgements
This work was supported by the Spanish Ministerio de Ciencia e Innovación (PID2020-113355GB-I00, the Agencia Estatal de Investigación (AEI) and the European regional fund (ERF), and the CDTI project (IDI-20190017). The study was partially supported by the Generalitat de Catalunya (2017SGR170). L. R.-R is lecturer of the Serra-Hunter program, Generalitat de Catalunya. C. G-G. has a fellowship from the University of Barcelona. L. S-C has a Maria Zambrano fellowship from the Spanish Ministerio de Universidades. M.D.R-B has a Margarita Salas fellowship from the Spanish Ministerio de Universidades.
Author contributions
PQ, LSC, CGG, GV, TYC, SGG, SGM performed the experiments, MDRB and LRR performed sequencing analysis, PQ, SA, AV, IS and MM conceived and funded the study, designed the experiments, and wrote the paper.
Data Availability
All data are available in the main text or the supplementary online materials. The phage genome is available at GenBank accession number OL634959.
Competing interests
The authors declare no competing interests.
References
Supplementary information The online version contains supplementary material available at https://doi.org/10.1038/s41396-023-01380-6.