-
PDF
- Split View
-
Views
-
Cite
Cite
Wiebke Bensmann, Veit Roessner, Ann-Kathrin Stock, Christian Beste, Catecholaminergic Modulation of Conflict Control Depends on the Source of Conflicts, International Journal of Neuropsychopharmacology, Volume 21, Issue 10, October 2018, Pages 901–909, https://doi.org/10.1093/ijnp/pyy063
- Share Icon Share
Abstract
To display goal-directed behavior, we must be able to resolve response conflicts that arise from processing various distractors. Such conflicts may be triggered by different kinds of distractor stimuli (e.g., priming and flanker stimuli), but it has remained largely unclear whether the functional and neurobiological underpinnings of both conflict types differ. We therefore investigated the functional relevance of the catecholamines dopamine and norepinephrine, which have been shown to increase the signal-to-noise ratio in neuronal processing and should therefore modulate response conflicts.
In a double-blind, randomized, placebo-controlled study design, we examined the effect of methylphenidate (0.5 mg/kg) on both flanker-induced and priming-induced response conflicts in a group of n=25 healthy young adults. We used EEG recordings to examine event-related potentials in combination with source localization analyses to identify the cognitive-neurophysiological subprocesses and functional neuroanatomical structures modulated by methylphenidate.
Compared with placebo, methylphenidate decreased flanker conflicts. This was matched by increased congruency effects in the fronto-central N2/P3 event-related potential complex and associated with modulations in the right inferior frontal gyrus. In contrast to this, methylphenidate did not modulate the size of prime-evoked conflicts.
Our results suggest that catecholamine-driven increases in signal-to-noise ratio and neural gain control do not equally benefit differently evoked conflicts. This supports the hypothesis of an at least partly different neurobiological basis for flanker- and prime-evoked response conflicts. As the right inferior frontal gyrus plays an important role in inhibition, the catecholaminergic system may reduce flanker conflicts by supporting the inhibition of distracting information.
Goal-directed behavior is vital to living a successful and self-serving life. It is however not only error-prone but may also be impaired by many different factors, including several kinds of distractors. Notably, distractors can be of different kinds. We pharmacologically modulated the catecholaminergic system with methylphenidate, which is commonly used in the treatment of various mental illnesses including ADHD. The results show that methylphenidate only partly fosters goal-directed behavior, as its beneficial effect on conflict size seems to depend on the nature of the distractor stimuli. These results provide insights into the complex neuropharmacological basis driving important aspects of goal-directed behavior in humans.
Introduction
Goal-directed behavior is expedient to living a successful life. An important aspect of goal-directed behavior is the ability to select the appropriate response out of several response alternatives. This process may however be hampered by irrelevant distracting information that generates response conflicts (Botvinick et al., 2001; Keye et al., 2013). The sources of these interferences and distraction can be different. Often, interference and conflict is induced by co-occurring stimuli flanking a target stimulus (Keye et al., 2013). However, conflicts can also be induced by temporally preceding (i.e., “priming”) stimuli (Boy et al., 2010; Stock et al., 2016, 2017). Priming and flanker effects are usually treated as different kinds of effects and were therefore investigated separately (Denton and Shiffrin, 2012). It has become evident, however, that prime- and flanker-induced conflicts may conjointly modulate interference control (Eimer and Schlaghecken, 2003; Schlaghecken and Eimer, 2004; McBride et al., 2012; Parkinson and Haggard, 2014; Ulrich et al., 2015; Gohil et al., 2017) and conflict processing (Beste et al., 2018b). The fact that priming and flanking information can conjointly modulate interference control and conflict processing (Beste et al., 2018b) suggests that both sources of conflict share a common neuronal basis (Boy et al., 2010; Stock et al., 2016, 2017). Until now, it has been debated whether the nature and neurobiological foundations of these 2 sources of conflict are the same (Vorberg et al., 2003; Boy et al., 2010; Stock et al., 2016, 2017). This is important because one main goal of treatments administered to patients with various neuropsychiatric disorders (e.g., attention deficit hyperactivity disorder) is to foster goal-directed behavior in order to reduce the impact of disturbing or distracting information. We therefore probe the nature and neurobiological foundations of these 2 kinds of conflicts, applying a neuropsychopharmacological approach investigating whether a common modulator of one of the 2 conflict types causes comparable effects in the other type of conflict.
When looking for a suitable modulator, it is important to consider the conceptual and cognitive faculties required for action selection in the face of response selection conflicts: such conditions make it necessary to enhance goal-shielding processes to stabilize and protect task goals from interference (Goschke and Dreisbach, 2008; Goschke and Bolte, 2014). This means it is important to enhance processing of task-relevant sensory information and reduce the impact of information-activating task-irrelevant response representations. Put in more technical terms, it is important to increase the system’s sensitivity towards relevant signals and/or to decrease the system’s sensitivity to noise (i.e., stimuli that interfere with or are irrelevant to the primary goal of responding). This modulation of the signal-to-noise ratio (SNR) of incoming sensory information and neural information processing can be conceptualized in the framework of neuronal gain control (Servan-Schreiber et al., 1990; Li et al., 2001; Yousif et al., 2016; Ziegler et al., 2016). Gain control has been demonstrated to be evident at sensory, cognitive (Salinas and Thier, 2000), and motor levels (Greenhouse et al., 2015; Thura and Cisek, 2016). Elevated dopaminergic (Servan-Schreiber et al., 1990; Li et al., 2001; Yousif et al., 2016; Ziegler et al., 2016) as well as norepinephrinergic signaling (Aston-Jones and Cohen, 2005; Nieuwenhuis et al., 2005) have been suggested to increase gain control, thus making catecholaminergic signaling a potentially suitable modulator of both kinds of response conflict. Both the dopamine and norepinephrine system are therefore important to consider when examining the neuropsychopharmacological basis of conflict monitoring. Based on this, we set out to investigate the effects of methylphenidate (MPH), which acts as a combined dopamine/norepinephrine transporter blocker, thus increasing postsynaptic dopamine and norepinephrine levels (Volkow et al., 1999; Skirrow et al., 2015; Faraone, 2018). It is possible that MPH increases goal-shielding processes that stabilize and protect task goals from interference via gain control mechanisms. Yet we hypothesize that MPH modulates only flanker-evoked conflicts but not prime-evoked conflicts. The reason behind this hypothesis is that previous results suggest that MPH exerts effects attributable to gain modulation principles when the incoming sensory information is salient and above a sufficient level of signal strength (Beste et al., 2018a), which may not be the case for priming stimuli. We therefore expect a neuropsychopharmacological dissociation between conflict induced by primes and flanker stimuli, with only the latter modulated by MPH.
To test this hypothesis, we used an experimental paradigm featuring flankers and a masked prime (Boy et al., 2010; Stock et al., 2016, 2017). Using electrophysiological techniques (i.e., event-related potentials [ERPs]), different cognitive-neurophysiological subprocesses involved in information processing and response inhibition can be isolated. In combination with source localization, this approach allows to examine the functional neuroanatomical network underlying the cognitive subprocesses modulated by increases in catecholaminergic signaling. Using ERPs, it has been shown that modulatory effects on flanker-type conflicts are reflected by the N2 component (van Veen and Carter, 2002; Botvinick et al., 2004; Folstein and Van Petten, 2008; Beste et al., 2010; Ullsperger et al., 2014; Stock et al., 2016 p.201). This component may, however, also reflect the influence of priming interference on response selection (Beste et al., 2018b). It further seems that there is a uniform sequence of cortical activity reflected by a fronto-central negativity-positivity (N2/P3) complex. This complex may reflect a common process of oscillatory cortical activity related to the processing of fast alarm signals indicating conflicts and thus for the necessity of increased cognitive effort and, potentially, behavioral adaptation (Ullsperger et al., 2014). Crucially, the fronto-central N2/P3 complex is modulated by the dopamine and the norepinephrine system (Warren et al., 2011; Ullsperger et al., 2014; Chmielewski et al., 2017; Mückschel et al., 2017a). Since MPH is expected to increase goal-shielding processes, thereby stabilizing and protecting task goals from interference, it is likely that response selection is less prone to interference under MPH administration. We therefore hypothesized that the fronto-central N2/P3 complex shows smaller amplitude (activity/power) modulations in response to flanker conflicts after MPH administration compared with a placebo. At the functional neuroanatomical level, these effects are expected to be reflected by modulations of activity in medial frontal regions and/or orbitofrontal and inferior frontal regions. Medial frontal regions are well-known to be involved in conflict-monitoring processes (Botvinick et al., 2004). Orbitofrontal and inferior frontal regions may also be important to consider because they are part of an inhibitory control network (Bari and Robbins, 2013; Aron et al., 2014; Allen et al., 2018). Inhibitory control is important during conflict resolution as it partly relies on the strengthening of inhibitory influences at the unwanted (incompatible) response representation (Stürmer et al., 2000; Verleger et al., 2009; Ocklenburg et al., 2011; Klein et al., 2014). Moreover, several lines of evidence put gain control mechanisms close to such mechanisms of inhibitory control (Priebe and Ferster, 2002; Mitchell and Silver, 2003; Papasavvas et al., 2015) and thus processes that are likely to be modulated by MPH (Beste et al., 2018a). Of note, the effects of primes on the fronto-central N2/P3 complex are not expected to be modulated by MPH, as we expect no behavioral effects. Likewise, we did not expect MPH modulations in lower-level perceptual and attentional selection processes, as they do not seem to play critical role in conflict monitoring (Gohil et al., 2017; Stock et al., 2017; Beste et al., 2018b).
Materials and Methods
Participants
A group of n=25 healthy young participants (mean age 23.92; SD 2.88; range 19–31 years; 15 females) took part in this study. All participants were right-handed, had normal or corrected-to-normal vision, reported no regular drug and/or medication intake, and did not consume caffeine at the day of investigation. None of the participants reported any psychiatric disorders or neurological diseases. Participants were recruited at the local University (TU Dresden). Each participant gave written informed consent and was reimbursed with either 75€ or course credits for taking part in the study. The study was conducted in accordance with the Declaration of Helsinki and approved by the ethics commission of the Medical Faculty of the TU Dresden.
MPH Administration
The study was conducted using a within-subject design where each subject was tested 2 times. In one of the 2 appointments, participants received a single dose of MPH. At the other appointment, participants were administered a placebo. The order of substance administration (MPH and placebo) was counter-balanced across subjects and blind to the experimenter. The individual MPH dosage was calculated based on the participant’s body weight (0.50 mg/kg) at the beginning of the first appointment. Because MPH plasma levels peak 1 to 3 hours after oral administration (Challman and Lipsky, 2000; Rösler et al., 2009), the investigation started approximately 2 hours after MPH administration. Participants were informed about the goals and procedure of this study and gave written consent. All participants received monetary compensation after the second appointment. The study was approved by the local ethics committee of TU Dresden and the experiment was conducted according to the Helsinki Declaration of 1975. All subjects provided written informed consent.
Task
The task was based on a paradigm by Boy et al. (2010) and identical to the experimental setup used in a previous study (Stock et al., 2016). This task allows to investigate conflicts evoked by flanker and prime distractor stimuli. Participants were seated 57 cm from a 17-inch CRT monitor and were asked to respond using a QWERTZ keyboard. To present the stimuli, record behavioral responses, and synchronize with the EEG, we used the Presentation software (Version 17.1 by Neurobehavioural Systems, Inc.). Before the experiment started, subjects practiced the task. During the practice, the experimenter answered questions to ensure that the participants understood the task. During the practice, feedback was provided about the accuracy of the response, while the experiment / data collection did not comprise response feedback.
Each trial started with the presentation of a central white fixation cross against a black background for 100 ms (Figure 1). It was followed by the prime for 30 ms, a mask stimulus for 30 ms, and the combination of target and flankers for 100 ms. The prime consisted of a single horizontal arrow pointing to the right or left. The mask was an array of randomly distributed lines. The target was a single horizontal arrow pointing either left or right, located between 2 vertically aligned flanker stimuli (arrows just like the prime and target). Participants were asked to identify the pointing direction of the central target arrow by pressing the right “Ctrl” button with the right index or middle finger when the target arrow pointed to the right and the left “Ctrl” button with the left index or middle finger when the target pointed to the left. Each trial ended when the participant’s response, which was required until 2000 ms after the onset of the target had elapsed. If no response was executed, the trial was categorized as a “miss.” The response-stimulus-interval between the participant’s response and the onset of the following trial was jittered between 1000 and 1200 ms. Whenever the prime and target pointed in the same direction, the trial was rated as compatible (and as incompatible in case they pointed in the opposite direction). In the case flankers and target pointed in the same direction, trials were classified as congruent (and as incongruent when they pointed in the other direction). There are thus 4 possible conditions: compatible-congruent, incompatible-congruent, compatible-incongruent, and incompatible-incongruent. In total, the task consisted of 384 trials, equally divided into 4 blocks. All combinations of conditions were presented equally often and were randomized within each block. In total, the experiment took approximately 15 minutes to complete.

Experimental paradigm. Each trial started with a presentation of a fixation cross for 100 ms. This was followed by a presentation of a prime (middle arrow) for 30 ms and presentation of a mask (array) for 30 ms. The target (middle arrow) plus flankers were presented for 100 ms. After target presentation, the screen turned black. Primes pointing in the same direction as the target were compatible (opposite direction=incompatible), while flankers that pointed in the same direction as the target were classified as congruent (opposite direction=incongruent).
EEG Recording and ERP Analysis
The EEG data were recorded using a QuickAmp amplifier (Brain Products, Inc.), and 60 Ag-AgCl electrodes were used at equidistant scalp positions with a sampling rate of 500 Hz. The reference electrode was located at Fpz and electrode impedances were kept <5 kΩ. The Brain Vision Analyzer 2.1 software package was used for offline data preprocessing and ERP data analyses. The recorded data were downsampled to 256 Hz, and a band-pass filter ranging from 0.5 to 20 Hz with a slope of 48 db/oct each was applied. A manual raw data inspection was then implemented to remove technical or muscular artifacts, while periodically occurring artifacts such as eye blinks, saccades, or pulse were subsequently detected and corrected using an independent component analysis (infomax algorithm). Lastly, we conducted another raw data inspection to remove any residual artifacts. The preprocessed data were then segmented in a target-locked fashion. Segments started 500 ms prior to the target stimulus onset (set to time point zero) and ended 1000 ms thereafter. Only correct trials were included in the data analysis. Next, an automated artifact rejection procedure was applied to remove all segments in which amplitudes were < -100 μV or >100 μV, had a value difference of >200 μV in a 200-ms interval, or in which segments revealed activity <0.5 μV in a 100-ms interval. After that, a current source density transformation was applied to eliminate the reference potential from the data. The current source density operates as a spatial filter identifying the electrodes that best reflect activity related to the respective ERP (Perrin et al., 1989; Nunez and Pilgreen, 1991; Kayser and Tenke, 2015). A baseline correction was then set to a time interval from -500 to -200 ms to obtain a prestimulus baseline. Finally, the different conditions were averaged at the single-subject level. Electrodes for data quantification were chosen on the basis of a literature-driven visual inspection of the ERPs and corresponding topography maps. This visual inspection was validated and confirmed by a procedure described in (Mückschel et al., 2014): within each of the corresponding search intervals, the peak amplitude was extracted for all 60 electrodes. Each electrode was subsequently compared against the average of all other electrodes using Bonferroni-correction for multiple comparisons. Only electrodes that showed significantly larger mean amplitudes than the remaining electrodes were selected. According to this procedure, the amplitudes of the prime-and target-evoked visual P1 and N1 were quantified at electrodes P7 and P8 (prime P1: 45–65 ms; prime N1: 95–150 ms; target P1: 155–200 ms) and at electrodes P9 and P10 (target N1: 245–290 ms). The fronto-central N2/P3 ERP-complex was quantified in a time window of 275 to 475 ms at electrode Cz. All ERP components were quantified relative to the prestimulus baseline. Peaks of all these ERPs were quantified using the area under the curve. The main reason for this was that longer quantification intervals (like the one used for the N2/P3 complex) usually encompass both negative and positive deflections that might cancel each other out when a simple mean value is formed. All ERP components were quantified on the single-subject level. Whenever the quantification took place at more than one electrode, amplitudes were averaged over all quantified electrodes.
Source Localization Analysis
To identify functional neuroanatomical structures that are differentially modulated by MPH or placebo administration, a source localization analysis was conducted. For this analysis, standardized low resolution brain electromagnetic tomography (sLORETA; Pascual-Marqui, 2002) was used. This procedure reveals high convergence with fMRI data and neuronavigated EEG/TMS studies, which underlines the validity of the estimated sources (Hoffmann et al., 2014; Dippel and Beste, 2015). sLORETA gives a single linear solution to the inverse problem based on extra-cranial measurements without a localization bias (Pascual-Marqui, 2002). For sLORETA, the intracerebral volume is partitioned into 6239 voxels at 5 mm spatial resolution. Then, the standardized current density at each voxel is calculated in a realistic head model (Fuchs et al., 2002) based on the MNI152 template (Mazziotta et al., 2001). The voxel-based sLORETA images were compared across conditions using the sLORETA-built-in-voxel-wise randomization tests with 2000 permutations based on statistical nonparametric mapping. Voxels with significant differences (P<.01, corrected for multiple comparisons) between contrasted conditions were located in the MNI-brain.
Statistics
Only correct trials with response times (RTs) between 100 and 1000 ms after the target onset were included in behavioral and neurophysiologic analyses to exclude trials with premature responses and reduce the effect of outliers on mean hit RTs. The data were analyzed using repeated-measures ANOVAs. The factors prime compatibility (compatible vs incompatibility), flanker congruency (congruent vs incongruent), and drug/placebo (MPH vs placebo) were included as within-subject factors. The degrees of freedom were adjusted using Greenhouse-Geisser correction and were Bonferroni corrected whenever necessary. For all descriptive statistics, the mean and SEM are given as a measure of variability.
Results
Behavioral Data
Accuracy
The analysis of the percentage of hits revealed a main effect of drug/placebo [F(1,24)=9.28, P<.006, =.279]. Participants responded less accurately under placebo administration (95.35%±0.68) than MPH administration (97.00%±0.41). Moreover, there was a main effect of prime compatibility [F(1,24)=47.27, P<.001, =.663] with a higher percent of hits for compatible (98.99%±0.17) than for incompatible trials (93.35%±0.89) and a significant main effect of flanker congruency [F(1,24)=32.95, P<.001, =.579], showing that participants responded less accurately in incongruent (95.13%±0.62) than in congruent trials (97.21%±0.40). Importantly, there was an interaction of flanker congruency and drug/placebo [F(1,24)=5.35, P=.030, =.182] (Figure 2A).
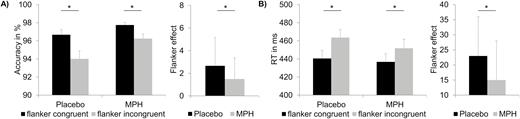
Behavioral data. (A) Accuracy data (mean percentage of correct responses) are shown for the different conditions (left) and also the flanker effect (congruency effect) is shown in the methylphenidate (MPH) and the placebo condition (right). (B) Reaction time (RT) data (mean reaction times) are shown for the different conditions (left), and the flanker effect (congruency effect) is shown in the MPH and placebo conditions (right). Significant results (P≤.05) are denoted with an asterisk. Error bars show the SEM as a measure of variability.
Posthoc tests indicated that the flanker congruency effect (i.e., congruent - incongruent) was larger under placebo administration (2.66%±2.50) than MPH administration (1.49%±1.86) [t(24)=2.31; P=.030]. The placebo-MPH effect was larger in the incongruent condition [t(24)=3.12; P=.002; MPH: 96.25%±0.52; placebo: 94.01%±0.87] than in the congruent condition [t(24)=2.36; P=.026]. All other main effects and interactions of the accuracy analyses were not significant (all F≤3.45; P≥.075). The only exception was the prime compatibility x flanker congruency interaction, shown in the supplemental material.
Response Times (RTs)
The repeated-measures ANOVA for RTs in correct trials revealed a main effect of prime compatibility [F(1,24)=287.34, P<.001, =.923] (Figure 2). Participants responded faster when prime and target were compatible (415 ms±9) than when they were incompatible (480 ms±10). Additionally, a main effect of flanker congruency was found [F(1,24)=86.45, P<.001, =.783], as participants responded faster when prime and target pointed in the same direction (438 ms±9) then when they pointed in incongruent directions (457 ms±9). Importantly, there was also a flanker congruency x drug/placebo interaction [F(1,24)=6.90, P=.015, =.223] (Figure 2B). Posthoc tests indicated that the flanker congruency effect (i.e., congruent - incongruent) was larger under placebo administration (23.1 ms±12.8) than under MPH administration (15.0 ms±12.9) [t(24)=2.62; P=.015]. However, the placebo-MPH effect did not differ between the incongruent and the congruent condition [t(24)=0.62; P > .4]. All other main effects and interactions of the accuracy analyses were not significant (all F≤1.15; P≥.293), except a prime compatibility x flanker congruency interaction, shown in the supplemental Material.
In summary, the behavioral data show that the congruency effect (i.e., the flanker effect) was smaller with MPH than with placebo and that MPH had larger effects on incongruent than congruent trials. The RT data patterns show a similar effect even though the placebo-MPH effect was not different for congruent and incongruent trials. The behavioral data show that MPH modulates flanker-related conflicts but not prime-related conflicts.
Neurophysiological Data
Prime P1 and Prime N1
The prime- and target-elicited P1 and N1 are illustrated in Figure 3.
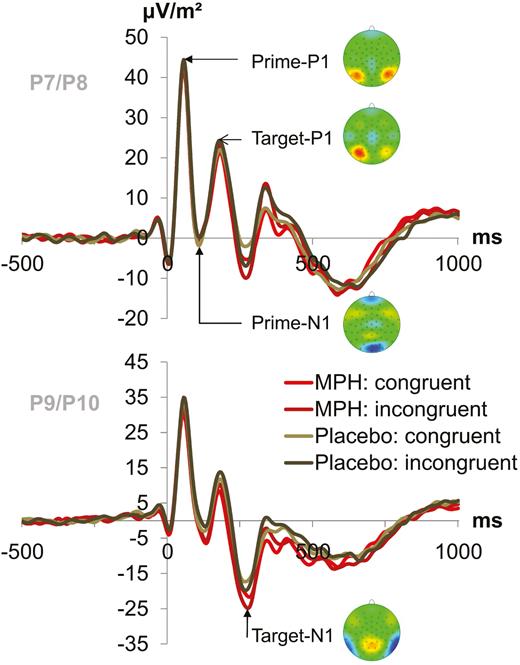
P1 and N1 event-related potential (ERP)-components. (A) Target-locked P1 and N1 ERPs elicited by the prime stimulus and target stimulus (onset at time point zero) at electrodes P7 and P8 (pooled) for the prime P1, prime N1, and target P1 and at electrodes P9 and P10 (pooled) for the target N1. Each combination of drug/placebo and flanker congruency is depicted separately (green denotes the placebo condition and red the MPH condition while lighter shades of the respective color denote congruent flankers and darker shades denote incongruent flankers). Topography maps of the peaks are depicted next to the respective peak names. Red colors denote positive values, blue colors negative values.
None of the investigated factors or interactions reached significance for the prime-elicited P1 (all F≤1.59; P≥.218) and prime-elicited N1 (all F≤.15; P≥.699).
Target P1 and Target N1
For the target-elicited P1 (pooled across electrodes P7 and P8), there was a significant main effect of prime compatibility [F(1,24)=13.46, P<.001, =.359], with larger areas in incompatible (997.66 μV*ms±188.58) than in compatible (912.79 μV*ms±194.12) trials. Also, there was a main effect of flanker congruency [F(1,24)=13.33, P<.001, =.357] with smaller areas in congruent (917.33 μV*ms±188.46) than incongruent (993.12 μV*ms±194.10) trials. Furthermore, an interaction of prime compatibility x flanker congruency [F(1,24)=8.97, P=.006, =.272] was found, which is detailed in the supplemental Material. All other target P1 main effects and interactions were not significant (all F≤2.00; P≥.170).
The analysis of the target-elicited N1 (pooled across electrodes P9 and P10) revealed a significant main effect of prime compatibility [F(1,24)=22.77, P<.001, =.487], showing smaller areas in compatible (-738.29 μV*ms±157.96) than in incompatible trials (-1058.59 μV*ms±180.28). A main effect of flanker congruency [F(1,24)=7.70, P=.011, =.243] was also found. Target-elicited N1 areas were smaller in trials with congruent flankers (-795.43 μV*ms±158.12) than in trials with incongruent flankers (-1001.45 μV*ms±181.54). All other target N1 main effects and interactions were not significant (all F≤3.34; P≥.080).
Fronto-Central N2/P3 Complex
The fronto-central N2/P3 complex at electrode Cz is shown in Figure 4.
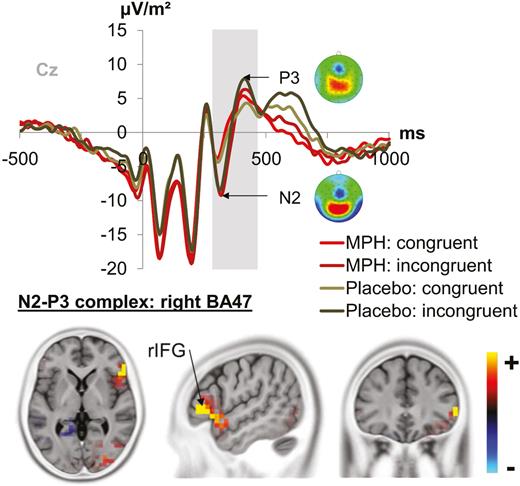
Fronto-central N2-P3 complex. The graph separately depicts the N2-P3 complex (marked by a grey bar) at electrode Cz. Each combination of drug/placebo and flanker congruency (green denotes the placebo condition and red the methylphenidate (MPH) condition, while lighter shades of the respective color denote congruent flankers and darker shades denote incongruent flankers) are depicted. Topography maps of the peaks are depicted next to the respective peak names. Red colors denote positive values, blue colors negative values. The sLORETA plots (corrected for multiple comparisons using statistical nonparametric mapping) show the source of the differential modulations induced by MPH vs placebo depending on flanker congruency. The source was in the right inferior frontal gyrus (rIFG; BA47). The standardized low resolution brain electromagnetic tomography (sLORETA) color scale denotes critical t values.
There was only an interaction of flanker congruency x drug/placebo [F(1,24)=5.59, P=.027, =.196]. Posthoc t tests showed that the congruency effect (i.e., congruent - incongruent) was larger under MPH administration (294.21 μV*ms±135.01) than under placebo administration (-89.96 μV*ms±152.71) [t(24)=2.1; P=.023]. It is further shown that only in the MPH condition incongruent and congruent trials differed in the amplitude (i.e., AUC) of the N2/P3 complex, with smaller areas in incongruent (172.02 μV*ms±601.76) than congruent trials (466.24 μV*ms±610.35). No such effect was evident under placebo administration [t(24)=.58; P=.561]. Source localization via sLORETA revealed that these effects, driven by the incongruent flanker condition, were associated with activation differences in the right inferior frontal gyrus (rIFG, BA47).
Except for a prime compatibility x flanker congruency interaction [F(1,24)=7.75, P=.010, =.244] detailed in the supplemental Material, no other interaction effects (including the factor prime compatibility) were evident in the N2/P3 complex (all F≤3.00; P≥.096).
In summary and similar to the interaction of flanker congruency and drug/placebo observed at the behavioral level, we found corresponding effects in the N2/P3 period that were associated with the rIFG. Area differences (congruent minus incongruent) were more pronounced under MPH administration than placebo.
Discussion
In the current study, we examined the role of dopaminergic and norepinephrinergic signaling for prime- and flanker-evoked stimuli. While the 2 kinds of conflicts usually occupy 2 different scientific niches (Denton and Shiffrin, 2012), there is increasing evidence that both types of conflict may share a common neuronal basis, as they have been found to interact (Boy et al., 2010; Stock et al., 2016, 2017). To further examine this, we assessed the effects of MPH administration on the 2 kinds of response conflicts using an electrophysiological (ERP) approach, combined with source localization analyses. Despite the potential neuronal overlap, we hypothesized that especially conflicts induced by flanker stimuli, but not conflicts due to priming, should be modulated by MPH.
The behavioral data were in line with this hypothesis: MPH did not cause a general increase in response accuracy but also decreased the size of flanker conflicts in both behavioral measures. Posthoc analyses further suggested that at least for accuracy, this effect was more strongly driven by improvements in the conflict condition (incongruent flankers) than in the nonconflict condition (congruent flankers). As MPH did not modulate the size of priming conflicts, the behavioral data already suggest that the neurobiological or neuropsychopharmacological basis for these processes should be at least partly different.
The ERP data were in line with the behavioral results and only showed MPH-associated modulations of flanker conflicts, but not for priming conflicts. Both the prime and flankers initially induce stimulus-stimulus conflicts as the participants are asked to ignore those distractor stimuli and instead focus on the target (Mullane et al., 2009; van Gaal et al., 2010; Larson et al., 2014). It is well-known that those conflicts may already show the early stages of stimulus processing, as reflected by the P1 and N1 amplitudes (Luck et al., 2000; Zhang et al., 2017). But while we reliably observed this phenomenon, we found no MPH effects on early attentional processing ERPs. This suggests that lower-level perceptual and attentional selection processes reflected by the P1/N1 ERP components (Herrmann and Knight, 2001; Luck and Kappenman, 2013) are not modulated by MPH during the processing of response conflicts. Only the fronto-central N2/P3 complex, which is known to be modulated by the dopamine and the norepinephrine system (Warren et al., 2011; Ullsperger et al., 2014; Chmielewski et al., 2017; Mückschel et al., 2017a), reflected the MPH effects observed on the behavioral level. Here, MPH produced a larger flanker congruency effect with smaller AUC in incongruent than congruent trials. Remarkably, we found that after MPH administration, the fronto-central N2/P3 complex was less pronounced in the incongruent (flanker) condition. This imposes high demands on cognitive control and triggers fast alarm signals indicating conflicts and thus the necessity of increased cognitive effort and, potentially, behavioral adaptation (Ullsperger et al., 2014). MPH acts as a mixed dopamine/norepinephrine transporter blocker, thus increasing dopamine and norepinephrine levels (Volkow et al., 1999; Skirrow et al., 2015; Faraone, 2018). It seems that MPH reduces the necessity of processing fast alarm signals, indicating the potential need for action adaptation, and may also reduce the investment of effort. Increased DA and NE levels have been suggested to increase gain control (Servan-Schreiber et al., 1990; Li et al., 2001; Yousif et al., 2016; Ziegler et al., 2016) (Aston-Jones and Cohen, 2005; Nieuwenhuis et al., 2005). It is therefore possible that the modulations observed in the fronto-central N2/P3 complex reflect the effect of altered gain-control mechanisms that can occur during higher-level cognitive control processes (Salinas and Thier, 2000). In this context, it is important to consider that conflict resolution also relies on the strengthening of inhibitory influences on unwanted (incompatible) response representations (Stürmer et al., 2000; Verleger et al., 2009; Ocklenburg et al., 2011; Klein et al., 2014). This assumption has not only received support from studies in humans (Taylor et al., 2007; Tandonnet et al., 2011) and monkeys (Cisek and Kalaska, 2005), but several lines of evidence have put gain control mechanisms close to such mechanisms of inhibitory control (Priebe and Ferster, 2002; Mitchell and Silver, 2003; Papasavvas et al., 2015). It is hence possible that inhibitory control tendencies reflected by the fronto-central N2/P3 complex during conflict monitoring (Chmielewski et al., 2014) are needed less, as gain control is enhanced by an MPH-induced increase in DA and NE signaling. This could also explain the reduction of the AUC of the fronto-central N2/P3 complex. Intriguingly, and supporting this interpretation, the source localization analysis showed that the modulations observed in the fronto-central N2/P3 complex were associated with the rIFG (BA47). The rIFG has been shown to be part of an inhibitory control network (Bari and Robbins, 2013; Aron et al., 2014; Allen et al., 2018) and is assumed to fulfill a “braking function” in the context of inhibitory control (Aron et al., 2003, 2014, 2015; Mückschel et al., 2017b; Chmielewski et al., 2018). Remarkably, it was shown that activity of the locus coeruleus NE system modulates inhibitory control processes (Dippel et al., 2017) also via the rIFG (Chmielewski et al., 2017). It may be speculated that it is especially the MPH-induced modulation of the NE system that may underlie the observed modulations. Supporting this, a different study using the same experimental paradigm has shown that microstructural striatal alterations, which affect dopaminergic neural transmission, modulate priming and flanker-induced conflicts (Beste et al., 2018b). It is therefore possible that the DA and NE system play dissociable roles during conflict monitoring, depending on the source of conflict. The rIFG has furthermore been suggested to facilitate attention allocation to conflicting stimuli (Hampshire and Sharp, 2015). Yet, this is a rather unlikely explanation for the current findings, because we did not find any MPH-related effects in the P1 and N1 ERPs, which are thought to reflect attentional processes (Herrmann and Knight, 2001; Luck and Kappenman, 2013). Other studies have suggested that MPH may enhance response to conflicting (salient) stimulus, which may be regarded as contradicting the current results and the ERP level (Farr et al., 2014; Manza et al., 2016; Rosenberg et al., 2016). It should however be noted that these studies examined performance in stop signal tasks, which assess reactive motor inhibition and are therefore not directly comparable to the cognitive inhibition processes required in flanker-like conflict tasks (Stürmer et al., 2000; Verleger et al., 2009; Ocklenburg et al., 2011; Klein et al., 2014). Moreover, the MPH administration protocols of those studies likely resulted in substantially different MPH levels at the time point of testing compared with our study.
In summary, we examined the effects of MPH on the processing of different sources of conflicts and found that the improved SNR associated with catecholaminergic signaling only decreased flanker-evoked response conflicts. This was most likely due to better conflict monitoring / resource allocation associated with modulations in the rIFG, which is an integral part of the inhibitory network and might hence have helped suppress incorrect responses more efficiently. Our results suggest that catecholamine-driven increases in SNR/gain control only benefit flanker-induced conflicts but not priming-induced conflicts. Importantly, this supports the hypothesis of an at least partly different neurobiological basis for these sources of response conflicts. It is also in line with the claim that MPH might only improve gain modulation when the incoming sensory information is sufficiently salient / above a certain signal strength threshold.
Supplementary Materials
Supplementary data are available at International Journal of Neuropsychopharmacology online.
Acknowledgments
This work was supported by a grant from the Deutsche Forschungsgemeinschaft SFB 940 project B8.
Statement of Interest
None.
References
Author notes
A.-K.S. and C.B. contributed equally (shared senior authorship).