-
PDF
- Split View
-
Views
-
Cite
Cite
Carole Di Poi, Nicolas Brodu, Frédéric Gazeau, Fabrice Pernet, Life-history traits in the Pacific oyster Crassostrea gigas are robust to ocean acidification under two thermal regimes, ICES Journal of Marine Science, Volume 79, Issue 10, December 2022, Pages 2614–2629, https://doi.org/10.1093/icesjms/fsac195
- Share Icon Share
Abstract
Ocean acidification and warming (OAW) are pressing contemporary issues affecting marine life and specifically calcifying organisms. Here, we investigated the direct effects of OAW on life-history traits of the Pacific oyster Crassostrea gigas, the most cultivated bivalve species worldwide. We also tested whether parental conditioning history shaped the phenotypic characters of their progenies (intergenerational carryover effects). Adult oysters and their offspring were exposed to two temperatures (18°C, +3°C) under ambient pH conditions or under an end-of-century acidification scenario (−0.33 pH unit). In adults, we monitored standard biometric and reproductive parameters, stress response by quantifying neuroendocrine metabolites and gamete quality. In larvae, we measured hatching rate, size, biochemical quality, and behavior. We found that reducing pH reduced growth rate and activated the serotonin system, but increasing temperature attenuated these effects. There was no effect of pH on reproduction at either temperature, and no intergenerational carryover effects. Larval characteristics were similar between treatments, regardless of parental conditioning history. Thus, the Pacific oyster seems robust to changes in pH, and increasing temperature is not an aggravating factor. We emphasize that the use of neuroendocrine indicators holds promise for revealing sublethal impacts of environmental changes.
Introduction
Over the last 150 years, human activities have led to a massive release of carbon dioxide (CO2) into the atmosphere, thereby warming the Earth’s surface and the oceans (IPCC, 2019). When part of atmospheric CO2 is absorbed by seawater, it generates an increase in the concentration of hydrogen ions causing a decrease in pH, a process known as ocean acidification (OA) (Caldeira and Wickett, 2005; Orr et al., 2005). Concomitantly, the excess of hydrogen ions leads to a reduction in the bioavailability of carbonate ions (CO32−) that impacts growth, physiological rates, immune response, behavior, and survival of marine calcifiers such as bivalve molluscs (Orr et al., 2005; Kroeker et al., 2010, 2013; Gazeau et al., 2013; Parker et al., 2013; Figuerola et al., 2021). Bivalvia is the most studied taxonomic class because of the ecological, economic, and socio-cultural importance of this group, and they were noted as particularly sensitive to low seawater pH at all life stages (Gazeau et al., 2013; Kroeker et al., 2013; Parker et al., 2013; Gattuso et al., 2015; Waldbusser et al., 2015).
Facing the climate emergency, OA research has been one of the fastest growing areas in marine science over the past 15 years (Riebesell and Gattuso, 2015; Browman, 2016). The experimental designs conducted to address this issue have mainly consisted in exposing organisms to stable present and future seawater pH levels as projected for the open ocean (IPCC, 2019). However, for a better assessment of the impacts of OA on bivalves, we must consider the natural pH/pCO2 fluctuations found in coastal environments where these species thrive (Vargas et al., 2022). Indeed, the diurnal and seasonal variability of pH/pCO2 in coastal areas is greater than in oceanic waters and can result in present-day situations where seawater pH exceeds the OA projections for the end of the century (Melzner et al., 2013; Gobler and Baumann, 2016; Vargas et al., 2017; Saavedra et al., 2021; Torres et al., 2021). The responses of coastal organisms to OA under fluctuating pH regimes are generally different from those observed under stable conditions (Frieder et al., 2014; Mangan et al., 2017). This implies that for studies targeting coastal species, apprehending their OA responses at stable pH conditions may not be reflective of current or future environmental conditions and could lead to misleading extrapolations.
In addition, OA occurs concomitantly with other environmental changes such as water warming and deoxygenation (IPCC, 2019). Therefore, OA manipulative experiments should include other interacting factors. Among them, temperature is the most obvious and most studied parameter, as it is the other CO2-related problem that increases with acidification (Pelejero et al., 2010). Seawater warming can reduce, amplify, or not change the effects of OA depending on the species, population, and life stage (Byrne, 2011; Byrne and Przeslawski, 2013; Kroeker et al., 2013; Przeslawski et al., 2015).
Finally, OA experiments should include cross- and multigenerational dimensions to determine whether adaptation can occur rapidly enough that ecosystem functions and services remain unchanged (Riebesell and Gattuso, 2015). These experimental evolutionary studies are generally conducted on species with short generation times, e.g. phytoplankton (Lohbeck et al., 2012; Schlüter et al., 2016). Conversely, for species with longer generation times such as bivalves, we are currently limited in assessing the effects of cross generation exposure to OA, i.e. whether parental conditioning history influences offspring performance traits (Byrne et al., 2020). A pioneer study reveals positive carryover effects on offspring from parents exposed during reproductive conditioning to low pH in the Sydney rock oyster Saccostrea glomerata (Parker et al., 2012) that even persist into adulthood and subsequent generations (Parker et al., 2015). However, in the presence of multiple stressors, broodstock exposure to low pH was detrimental on larval survival (Parker et al., 2017). In contrast, parental exposure to low pH made clam Mercenaria mercenaria and scallop Argopecten irradians larvae more susceptible to OA (Griffith and Gobler, 2017), whereas there was no cross generation effect on the Eastern oyster Crassostrea virginica (Clements et al., 2021). Collectively, these results emphasize that the nature of the carryover effects, i.e. beneficial, detrimental, or neutral, is species-specific. Thus further research is needed before drawing large-scale conclusions about the adaptability of bivalves to near-future oceanic conditions.
Here, we aimed to determine whether exposure during reproductive conditioning to naturally fluctuating low pH under two thermal regimes shapes life-history traits of the Pacific oyster C. gigas, and to test whether parental exposure influences early development of the offspring (from fertilization to larval hatching) further referred to as intergenerational effects. Adult oysters and their first-generation progenies were exposed to ambient or acidified pH conditions (−0.33 pH unit relative to ambient) at a temperature of 18°C, for which reproductive development is optimal, and to +3°C relative to this optimum. These pH and temperature offsets were based on the high greenhouse gas emission scenario (Representative Concentration Pathway, RCP 8.5) predicted for the end of the century (IPCC, 2019), recently redefined as SSP5-8.5 under the CMIP6 Shared Socioeconomic Pathways (Kwiatkowski et al., 2020). Studying the effects of OA in combination with temperature rise will help predicting impacts of climate change in this ecologically and economically relevant species.
Specifically, we measured adult growth, food intake, sexual maturity, as well as larval hatching, shell size, and calcification. We also investigated new parameters that should shed new light on the potential for oyster adaptation to climate change. Among them, we quantified the concentrations of the components of the neuroendocrine system. Monoaminergic and amino acid neurotransmitters are present in the nervous system throughout the animal kingdom including in bivalves (Walker, 1996; Alavi et al., 2017b). There is accumulating evidence that, in this phylum, the neuroendocrine system plays a critical role in coordinating neurobehavioral systems as well as acts in peripheral organs to regulate physiological functions such as growth, reproduction, and development [e.g. for the serotonergic system, reviewed in Alavi et al. (2017a, b)]. It also serves to respond effectively to a wide range of environmental stresses and restores physiological homeostasis (reviewed in Liu et al., 2018).
We also evaluated gamete quality in terms of sperm motility (Boulais et al., 2015a) and egg biochemical contents. Indeed, endogenous lipid reserves invested in eggs fuel energetic needs for embryogenesis in bivalves (Whyte et al., 1990) and maternal reserves are greatly and rapidly mobilized to maintain homeostasis in larvae under acidified and warmer conditions (Talmage and Gobler, 2011; Gibbs et al., 2020, 2021). Thus, understanding how biochemical resources are constituted and consumed during early life development may be a promising avenue to further explore the energetic costs to OA and warming. Finally, we characterized swimming behavior of larvae because of its ecological relevance in survival and dispersal for invertebrate larval stages. Indeed, locomotion is a fundamental behavioral determinant for shellfish larvae that is directly affected by physiological status and shell morphology (Chan and Grünbaum, 2010; Chan, 2012). Swimming behavior has therefore recently been included in the panel of biological responses of invertebrate larvae that may be altered by OA (Chan et al., 2015, 2016; Meyer-Kaiser et al., 2019; Kavousi et al., 2022).
Material and methods
Animals
Adult oysters were produced in February 2017 at the Ifremer facilities located at Argenton (Brittany, France, Figure 1A) and grown nearby at Aber-Benoît (see Supplementary File S1 for details). On 6 February 2018, 412 oysters were transferred back to Argenton and kept at 13°C in 500-L flow-through tanks for 6 d prior to the onset of the experiment. The seawater came from a pool, which is renewed with each spring tide, filtered at 1 µm, and UV-treated (Figure 1A).
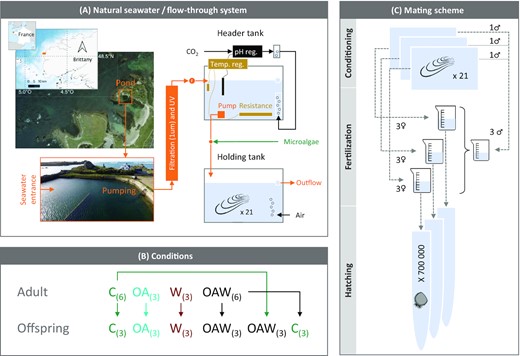
Experimental set-up (A), design (B), and mating scheme (C) during adult conditioning and larval exposure. Abbreviations: (A) reg.—regulation, (B) C—control (18°C, ambient pH), OA—ocean acidification (18°C, −0.33 pH unit), W—warming (21°C, ambient pH), OAW—ocean acidification and warming (21°C, −0.33 pH unit), and number of replicates is given in parentheses.
Experimental set-up
Natural seawater was evenly distributed to 18 independent experimental units consisting of one header tank, where seawater was acidified and/or warmed, connected to one holding tank containing the oysters (Figure 1A). Tanks were 45 L (60 × 40 × 19 cm) and the seawater was renewed every 80 min. For larvae, the holding tanks were 5 L cylindrical tube (100 × 9 cm ∅) and the seawater was renewed every 50 min. Seawater in oyster tanks (adult and larvae) was continuously aerated and mixed through air bubbling, the salinity ranged from 34.1 to 34.7 and the photoperiod was fixed at 14L:10D.
The acidified conditions were obtained by bubbling pure CO2 into the seawater of the header tank and the pH-level was controlled using a pH-controller system (JBL, Germany) allowing an accuracy of 0.05 pH unit. The controller probes were calibrated on a monthly basis throughout the experiment using NBS (National Bureau of Standards) buffer solutions at pH 4.00 and 7.00 (HANNA Instruments, France). The pH of the controllers was adjusted relative to the ambient pH twice daily to reach the targeted pH offset in the holding tanks. Each tank was equipped with a thermostat and heating resistor (Biotherm Ecco Hobby and SCHEIGO Titane 300 W, France) to maintain the seawater in the holding tanks at the targeted temperature. There was no tidal regime applied.
During the experiment, oysters were fed continuously on a mixed diet of Chaetoceros muelleri (CCAP 1010/3) and Tisochrysis lutea (CCAP 927/14) (1:1 in dry weight). Food concentration was 1500 μm3 μl−1 of microalgae at the outlet of the tank and controlled twice daily using an electronic particle counter (Multisizer 3, Beckman Coulter, USA) equipped with a 100-μm-aperture tube. Oysters did not produce pseudofaeces.
Experimental design
At the onset of the experiment on 13 February 2018, the oysters were 12-months-old [55 mm shell length (±6.1, SD), 22.5 g wet mass (±5.3, SD)], and at an undifferentiated stage of gametogenesis (histological observation; Supplementary File S2). They were evenly distributed in the holding tanks (N = 21 specimens per tank). Each tank was randomly assigned one of four possible conditions: control (C), acidified (OA), warm (W), and acidified and warm (OAW, Figure 1B). There were three replicates for OA and W and six replicates for C and OAW treatments. The control condition corresponded to the ambient seawater pH at the entry of the tanks and a standard seawater temperature for broodstock conditioning (18°C, see Fabioux et al., 2005). The OA and the warm conditions corresponded to a pH reduction of ca. 0.33 unit compared to the ambient pH and to a temperature increase of ca. 3°C, respectively, which correspond to the SSP5-8.5 climate projections (Lee et al., 2021). The OAW condition was the combination of the two factors (−0.33 pH unit, +3°C relative to the ambient conditions).
Under controlled conditions and optimal food availability, gonadal maturation is usually achieved after ca. 4.5–6.5 weeks of conditioning at 17°C (Petton et al., 2015). Ripeness was reached after 30 and 35 d for the warm (21.0 ± 0.1°C) and the ambient (18.0 ± 0.1°C) conditions, respectively, corresponding to 630° days (Mann, 1979). The gonads were incised and the gametes were recovered with filtered seawater on meshes. For each replicate, the oocytes of three random females from the same tank (ca. 250000 oocytes per female) were fertilized with the sperm pooled from three males from each tank per condition at a ratio of 30 spermatozoa per oocyte (Figure 1C). In total, 18 mating pairs were placed in 2 L jars at the same temperature and pH as the adults.
Two hours post-fertilization, the embryos were transferred to 5 L cylindrical tubes (density of 140 larvae mL−1) connected to the header tanks and exposed for 48 h until they reached the D-shaped larvae stage. For OA and W conditions, the progenies were exposed to the same condition as their parents. For C and OAW conditions, the progenies were reallocated in a reciprocal fashion to either the parental condition or to the opposite one, to investigate carryover effects (Figure 1B). There were six combinations of treatments in three replicate tanks. Seawater temperature for embryos was 1.5°C higher than that of their parents to foster development (19.5 ± 0.1°C for control and OA conditions, and 22.5 ± 0.1°C for W and OAW). Oyster larvae are endotrophic during this period, thus they were not fed.
Seawater carbonate chemistry
Seawater pHNBS, temperature, dissolved oxygen, and salinity, were monitored twice daily in the holding tanks with a multiprobe reference system (Multi 3630 IDS WT: pH Sentix 940–3; oxygen FDO 925; salinity TetraCon 925, temperature Checktemp 1 HANNA, Xylem Analytics, Germany). These measurements were used for adjusting the pH-controller settings. The accuracy of the reference pH-probe was checked once a week using Certipur® Merck NBS buffers (pH = 4.00, 7.00, and 9.00) and calibrated three times a week on the total scale (pHT) with a certified Tris/HCl buffer (salinity 35; provided by A. Dickson, Scripps University).
Total alkalinity (AT) was assessed on 0.45-μm-filtered seawater samples (180 mL) collected three times during the experiment in three randomly chosen header tanks under control, OA and OAW conditions. Seawater samples were poisoned immediately with 75 µL saturated mercuric chloride solution. AT was determined potentiometrically using an automatic titrator (888 Titrando Metrohm). Triplicate titrations were performed on 10 mL subsamples at 20°C and AT was calculated as described by Dickson (2007). Titrations of certified reference material provided by A. Dickson (Scripps Institution of Oceanography, USA; batch number 170) yielded AT values within 12 μmol kg−1 of the nominal value (SD = 16 μmol kg−1). The carbonate parameters (partial pressure of CO2, aragonite and calcite saturation states, dissolved inorganic carbon, bicarbonate, and carbonate concentrations) were determined from pHT, AT, temperature, and salinity using the CO2Sys-Excel version 2.1 originally developed by Pierrot et al. (2011).
Biological measurements
Detailed protocols for analytical and behavioral measurements are provided in Supplementary File S2.
Adults
Growth
The shell length (height in millimeters) and total body weight (shell plus flesh in grams) of each oyster were individually measured during the conditioning period after 10, 17, 24, and 30 d for all conditions, and after 35 d for the control and OA conditions. Shell and flesh weights were measured on 12–15 individuals per tank after 30 d for W and OAW, and after 35 d for C and OA.
Ingestion rate
The volume of microalgae, consumed per minute, was measured daily over the course of the experiment in each oyster tank using the following formula:
Ingestion rate (µm3 oyster−1 min−1) = ([Cellinlet]−[Celloutlet]) × waterflow; where the variables were the concentrations of microalgae at the inlet and outlet of the tank ([Cellinlet] and [Celloutlet] in μm3 of algae per μl of seawater) and the water flow in the oyster tank (mL min−1).
Reproduction
A total of four to five oysters per tank (out of the 12–15 individuals sampled for shell and flesh weights) were carefully dissected at the end of the conditioning period. A transversal section of the gonads was sampled for histological determination of sex, reproductive stage, and gonadal coverage, which is a proxy of the reproductive effort (Fabioux et al., 2005). The gonadal coverage was calculated as the gonad occupation area divided by the total transverse section area.
Neurochemical profiles
Within the nervous system, visceral ganglia were sampled, flash-frozen in liquid nitrogen and stored at −80°C for quantification of monoamine neurotransmitters dopamine, noradrenaline, serotonin (5-hydroxytryptamine, 5-HT), and its metabolite 5-hydroxyindoleacetic acid (5-HIAA), as well as amino acid neurotransmitters glutamate and gamma-amino-butyric (GABA) acid, using ultra-high-pressure liquid chromatography coupled with tandem-mass spectrometry (UHPLC-MS/MS). The contents were expressed in pmol mg−1 of tissue, and the ratio of 5-HIAA to 5-HT was used as an index of 5-HT turnover (Winberg and Nilsson, 1993; Winberg and Lepage, 1998).
Gamete quality
Gametes were sampled from the oysters used for reproduction. Extra males (one to two individuals per tank) were added to increase the sample sizes. The total number of oocytes collected was estimated for each female and the mean diameter was measured using ImageJ (Boulais et al., 2015b). Samples of ca. 20000 oocytes per female were collected, centrifuged at 4000 rpm for 1 min to remove the supernatant seawater, flash-frozen in liquid nitrogen, and stored at −80°C for fatty acid analysis. Total lipids were then extracted and transesterified using methanolic H2SO4. Fatty acid methyl esters were analysed in a gas chromatograph equipped with an on-column injector (DB-Wax capillary column) and a flame-ionization detector, with hydrogen as a carrier gas. The unsaturation index was calculated as the number of double bonds per 100 molecules of fatty acids.
Sperm concentration was determined by flow cytometry (EasyCyte Plus, Guava-Marck-Millipore) (Le Goïc et al., 2013). Sperm movement was triggered using a two-step dilution in an activating solution and analysed using the CASA software for ImageJ (Boulais et al., 2015a, 2018). The percentage of motile spermatozoa and their velocity (VAP: velocity of the average path in µm s−1) were assessed on a minimum of 30 spermatozoa. The fertilization yield is the percentage of fertilized oocytes relative to the total number of oocytes.
First-generation progenies
Biological parameters were measured at the D-shaped larvae stage, i.e. 48 h post-fertilization.
Development
Hatching rate, i.e. the number of D-larvae out of the total, and shell height, i.e. the greatest distance between the umbo and the ventral edge of the shell (minimum of 30 individuals per replicate, fixed in ethanol 70%), were measured in each tank by ImageJ software.
Shell calcification
Samples of larvae were fixed in ethanol 70% and mounted in a glycerol drop. About 50 larvae per replicate were photographed under phase contrast and polarized light. The intensity of birefringence was used as a proxy for the evaluation of calcium carbonate (CaCO3) mineralization (Wessel et al., 2018). Birefringence was assessed using ImageJ software and quantified as a greyscale score for polarized light images on a scale of 0–255.
Fatty acid composition
Fatty acid composition of the total lipids of larvae was determined on a pool of 100000 individuals per replicate. Samples were stored and analysed as previously described for oocytes.
Behavior
Larvae were transferred alive in a 96-well micro-plate filled with seawater (eight to ten animals per well; three wells per replicate) and observed under an inverted microscope connected to a camera (Leica, DMIL, MC 190HD). After a 5-min acclimation period, the mean velocity and the time moving of each animal in each well were recorded for 1 min at 30 frames per second at 1920 × 1080 dpi and quantified with EthoVision XT 13.0 (Noldus Information Technology, The Netherlands).
Statistics
General linear mixed-models were performed to assess differences in (1) pHT among conditions (4 levels) and time (38 levels); (2) the total body weight and shell length of adult oysters during conditioning depending on temperature and pH (2 × 2 conditions, main plots), time (4 levels, subplot), and initial parameter values (covariates, subplots); (3) the shell weight and flesh weight of oysters at the end of the conditioning depending on temperature and pH (2 × 2 conditions); (4) the ingestion rate of oysters during the conditioning depending on temperature and pH (2 × 2 conditions, main plots) and time (35 levels, subplots, repeated measurements); (4) the gonadal coverage and neurochemistry at the end of the conditioning depending on temperature and pH (2 × 2 conditions, main plots) and sex (2 levels, subplots); (5) oocyte and sperm quality at the end of the conditioning depending on temperature and pH (2 × 2 conditions); and (6) D-larvae quality and behavior depending on temperature and pH during the conditioning and the larval development (6 conditions). The unit of replication was the tank in which the conditions were applied (random factor). All mutual interactions among the factors were tested, and Tukey’s HSD was used as a post hoc test. The normality of residuals and homogeneity of variances were checked. Results are expressed as least-square means ± standard error (SE). All statistical analyses were conducted using SAS 9.4 software (SAS institute) and significance was set at α = 0.05. Statistical outputs are summarized in Supplementary File S3.
Results
Seawater carbonate chemistry
The pHT of natural seawater ranged from 7.94 to 8.15 throughout the experiment in the non-acidified conditions (C, W) and slightly increased over time (Figure 2, left panel). On average, pHT ranked as C > W >> OA = OAW (Figure 2, right panel). The average pHT offset between the acidified (OA and OAW) and control (C) conditions was −0.33 ± 0.04 pH unit. Overall, pCO2 levels varied over time but were always higher in the acidified conditions than in the control ones (Table 1). Alkalinity was relatively stable regardless of the conditions, varying from 2211 to 2367 µmol kg−1. Saturation states of aragonite and calcite (Ωar, Ωca) were much lower in the lowered pH conditions compared to the ambient and warmed ones, but seawater remained oversaturated (Ω > 1) in all treatments.
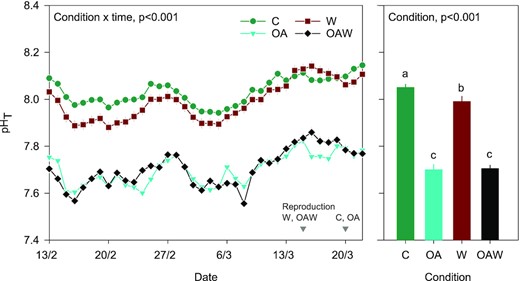
Temporal dynamics of pHT (on the total scale) in oyster tanks for each condition (left panel) and averaged values during the course of the experiment (right panel). Data are least-square means ± SE among tanks (n = 3 or 6). The dates of reproduction are indicated in grey for each condition in the left panel. Abbreviations: C—control (18°C, ambient pH), OA—ocean acidification (18°C, −0.33 pH unit), W—warming (21°C, ambient pH), and OAW—ocean acidification and warming (21°C, −0.33 pH unit).
. Parameters of the seawater carbonate chemistry over time during the adult conditioning and the larval exposure for each condition: salinity (S), temperature (T, °C), pH (pHT, total scale), total alkalinity (AT, µmol kg−1), partial pressure of carbon dioxide (pCO2, µatm), dissolved inorganic carbon concentration (CT, µmol kg−1), bicarbonate and carbonate concentrations ([HCO3−] and [CO32−], respectively, µmol kg−1), and saturation state of the seawater with respect to aragonite (Ωar) and calcite (Ωca).
Parameters measured . | Parameters estimated . | ||||||||||
---|---|---|---|---|---|---|---|---|---|---|---|
Date . | Condition . | S . | T . | pHT . | AT . | pCO2 . | CT . | [HCO3−] . | [CO32−] . | Ωar . | Ωca . |
Adult conditioning | |||||||||||
02/21/18 | C | 34.3 | 18.1 | 7.99 | 2313.1 | 490.6 | 2100.0 | 1925.0 | 158.2 | 2.5 | 3.8 |
OA | 34.3 | 18.0 | 7.72 | 2279.8 | 983.8 | 2182.5 | 2058.8 | 89.9 | 1.4 | 2.2 | |
OAW | 34.3 | 21.0 | 7.68 | 2255.6 | 1071.9 | 2156.5 | 2031.2 | 91.4 | 1.4 | 2.2 | |
03/02/18 | C | 34.7 | 18.0 | 7.97 | 2211.1 | 486.3 | 2009.8 | 1845.8 | 147.4 | 2.3 | 3.5 |
OA | 34.7 | 18.0 | 7.63 | 2288.9 | 1217.6 | 2219.2 | 2101.3 | 76.3 | 1.2 | 1.8 | |
OAW | 34.7 | 21.0 | 7.61 | 2225.0 | 1272.5 | 2151.2 | 2033.3 | 77.8 | 1.2 | 1.9 | |
48 h larval exposure | |||||||||||
03/26/18 | C | 34.1 | 21.1 | 8.22 | 2276.9 | 249.0 | 1909.6 | 1643.9 | 257.8 | 4.1 | 6.2 |
OA | 34.1 | 21.1 | 7.86 | 2272.4 | 684.8 | 2102.8 | 1949.4 | 131.8 | 2.1 | 3.2 | |
OAW | 34.1 | 23.7 | 7.84 | 2367.4 | 736.6 | 2180.9 | 2013.1 | 146.1 | 2.3 | 3.5 |
Parameters measured . | Parameters estimated . | ||||||||||
---|---|---|---|---|---|---|---|---|---|---|---|
Date . | Condition . | S . | T . | pHT . | AT . | pCO2 . | CT . | [HCO3−] . | [CO32−] . | Ωar . | Ωca . |
Adult conditioning | |||||||||||
02/21/18 | C | 34.3 | 18.1 | 7.99 | 2313.1 | 490.6 | 2100.0 | 1925.0 | 158.2 | 2.5 | 3.8 |
OA | 34.3 | 18.0 | 7.72 | 2279.8 | 983.8 | 2182.5 | 2058.8 | 89.9 | 1.4 | 2.2 | |
OAW | 34.3 | 21.0 | 7.68 | 2255.6 | 1071.9 | 2156.5 | 2031.2 | 91.4 | 1.4 | 2.2 | |
03/02/18 | C | 34.7 | 18.0 | 7.97 | 2211.1 | 486.3 | 2009.8 | 1845.8 | 147.4 | 2.3 | 3.5 |
OA | 34.7 | 18.0 | 7.63 | 2288.9 | 1217.6 | 2219.2 | 2101.3 | 76.3 | 1.2 | 1.8 | |
OAW | 34.7 | 21.0 | 7.61 | 2225.0 | 1272.5 | 2151.2 | 2033.3 | 77.8 | 1.2 | 1.9 | |
48 h larval exposure | |||||||||||
03/26/18 | C | 34.1 | 21.1 | 8.22 | 2276.9 | 249.0 | 1909.6 | 1643.9 | 257.8 | 4.1 | 6.2 |
OA | 34.1 | 21.1 | 7.86 | 2272.4 | 684.8 | 2102.8 | 1949.4 | 131.8 | 2.1 | 3.2 | |
OAW | 34.1 | 23.7 | 7.84 | 2367.4 | 736.6 | 2180.9 | 2013.1 | 146.1 | 2.3 | 3.5 |
Abbreviations: C—control, OA—ocean acidification, W—warming, and OAW—ocean acidification and warming.
. Parameters of the seawater carbonate chemistry over time during the adult conditioning and the larval exposure for each condition: salinity (S), temperature (T, °C), pH (pHT, total scale), total alkalinity (AT, µmol kg−1), partial pressure of carbon dioxide (pCO2, µatm), dissolved inorganic carbon concentration (CT, µmol kg−1), bicarbonate and carbonate concentrations ([HCO3−] and [CO32−], respectively, µmol kg−1), and saturation state of the seawater with respect to aragonite (Ωar) and calcite (Ωca).
Parameters measured . | Parameters estimated . | ||||||||||
---|---|---|---|---|---|---|---|---|---|---|---|
Date . | Condition . | S . | T . | pHT . | AT . | pCO2 . | CT . | [HCO3−] . | [CO32−] . | Ωar . | Ωca . |
Adult conditioning | |||||||||||
02/21/18 | C | 34.3 | 18.1 | 7.99 | 2313.1 | 490.6 | 2100.0 | 1925.0 | 158.2 | 2.5 | 3.8 |
OA | 34.3 | 18.0 | 7.72 | 2279.8 | 983.8 | 2182.5 | 2058.8 | 89.9 | 1.4 | 2.2 | |
OAW | 34.3 | 21.0 | 7.68 | 2255.6 | 1071.9 | 2156.5 | 2031.2 | 91.4 | 1.4 | 2.2 | |
03/02/18 | C | 34.7 | 18.0 | 7.97 | 2211.1 | 486.3 | 2009.8 | 1845.8 | 147.4 | 2.3 | 3.5 |
OA | 34.7 | 18.0 | 7.63 | 2288.9 | 1217.6 | 2219.2 | 2101.3 | 76.3 | 1.2 | 1.8 | |
OAW | 34.7 | 21.0 | 7.61 | 2225.0 | 1272.5 | 2151.2 | 2033.3 | 77.8 | 1.2 | 1.9 | |
48 h larval exposure | |||||||||||
03/26/18 | C | 34.1 | 21.1 | 8.22 | 2276.9 | 249.0 | 1909.6 | 1643.9 | 257.8 | 4.1 | 6.2 |
OA | 34.1 | 21.1 | 7.86 | 2272.4 | 684.8 | 2102.8 | 1949.4 | 131.8 | 2.1 | 3.2 | |
OAW | 34.1 | 23.7 | 7.84 | 2367.4 | 736.6 | 2180.9 | 2013.1 | 146.1 | 2.3 | 3.5 |
Parameters measured . | Parameters estimated . | ||||||||||
---|---|---|---|---|---|---|---|---|---|---|---|
Date . | Condition . | S . | T . | pHT . | AT . | pCO2 . | CT . | [HCO3−] . | [CO32−] . | Ωar . | Ωca . |
Adult conditioning | |||||||||||
02/21/18 | C | 34.3 | 18.1 | 7.99 | 2313.1 | 490.6 | 2100.0 | 1925.0 | 158.2 | 2.5 | 3.8 |
OA | 34.3 | 18.0 | 7.72 | 2279.8 | 983.8 | 2182.5 | 2058.8 | 89.9 | 1.4 | 2.2 | |
OAW | 34.3 | 21.0 | 7.68 | 2255.6 | 1071.9 | 2156.5 | 2031.2 | 91.4 | 1.4 | 2.2 | |
03/02/18 | C | 34.7 | 18.0 | 7.97 | 2211.1 | 486.3 | 2009.8 | 1845.8 | 147.4 | 2.3 | 3.5 |
OA | 34.7 | 18.0 | 7.63 | 2288.9 | 1217.6 | 2219.2 | 2101.3 | 76.3 | 1.2 | 1.8 | |
OAW | 34.7 | 21.0 | 7.61 | 2225.0 | 1272.5 | 2151.2 | 2033.3 | 77.8 | 1.2 | 1.9 | |
48 h larval exposure | |||||||||||
03/26/18 | C | 34.1 | 21.1 | 8.22 | 2276.9 | 249.0 | 1909.6 | 1643.9 | 257.8 | 4.1 | 6.2 |
OA | 34.1 | 21.1 | 7.86 | 2272.4 | 684.8 | 2102.8 | 1949.4 | 131.8 | 2.1 | 3.2 | |
OAW | 34.1 | 23.7 | 7.84 | 2367.4 | 736.6 | 2180.9 | 2013.1 | 146.1 | 2.3 | 3.5 |
Abbreviations: C—control, OA—ocean acidification, W—warming, and OAW—ocean acidification and warming.
Effects of temperature and pH changes on biometrics in adult oysters
During the conditioning period, temperature, pH, and time interacted in their effects on shell length and total body weight of adult oysters (Figure 3A and B). On average, shell length increased from 55.8 to 66.3 mm, which corresponds to an increase in total body weight from 22.7 to 32.3 g. At the end of the conditioning period, i.e. 30 d in W, OAW conditions and 35 d in C, OA conditions, oysters in acidified water at 18°C (OA) were smaller in terms of both shell length and total body weight than their counterparts reared in acidified and warmed (OAW), warmed only (W), and control conditions (C). The rise in temperature offset the negative effect of acidification alone on growth. There was no statistical difference in final shell and flesh weights between conditions (Figure 3C and D). In contrast, temperature, pH, and time interacted in their effects on ingestion rates and there was no significant difference between treatments (Figure 3E).
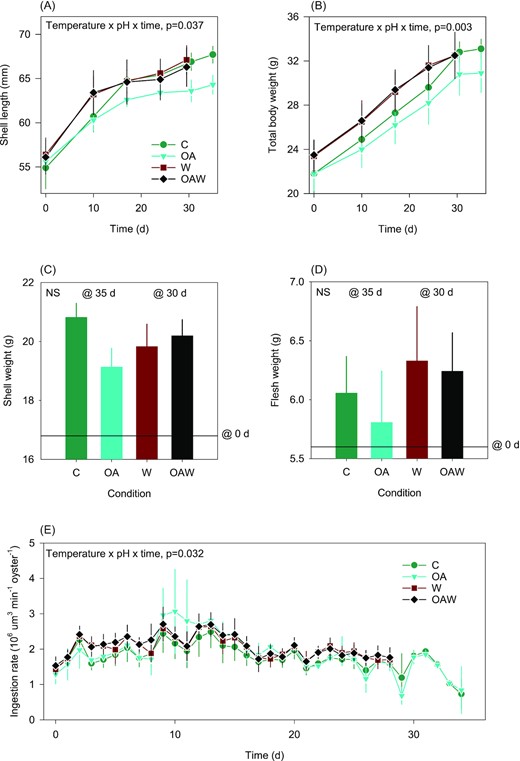
Temporal dynamics of oyster shell length (A) and total body weight (B), final shell weight (C), and flesh weight (D), as well as ingestion rate (E) during the conditioning period. Data are least-square means ± SE among tanks (n = 3 or 6). The black line in (C) and (D) indicates the average weight at the beginning of the experiment. Effects and p-values are indicated otherwise not significant (NS). Abbreviations: C—control (18°C, ambient pH), OA—ocean acidification (18°C, −0.33 pH unit), W—warming (21°C, ambient pH), and OAW—ocean acidification and warming (21°C, −0.33 pH unit).
Effects of temperature and pH changes on reproductive attributes in adult oysters
Ripeness was reached after 30 d of conditioning under warmer conditions (21°C in W, OAW) and 35 d under ambient ones (18°C in C, OA). At the end of the conditioning period, sex ratio (ca. 60/40 females/males), maturity level (majority stage 3), and gonadal coverage were similar for all treatments, while gonadal coverage was consistently higher in males (Figure 4A and B, Table 2).
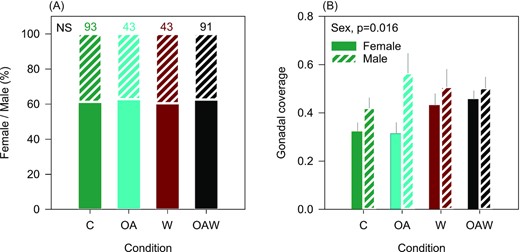
Sex ratio with numbers above bars indicating the numbers of adult oysters (A) and gonadal coverage in both sexes (B). Solid bars represent females and striated bars represent males. Data are least-square means ± SE among tanks (n = 3 or 6). Effects and p-values are indicated otherwise not significant (NS). Abbreviations: C—control (18°C, ambient pH), OA—ocean acidification (18°C, −0.33 pH unit), W—warming (21°C, ambient pH), and OAW—ocean acidification and warming (21°C, −0.33 pH unit).
Number of adult oysters at the end of the conditioning period for each gonadal stage determined according to the reproductive scale of (Steele and Mulcahy 1999) and according to the four conditions.
. | Gonadal stage . | . | |||
---|---|---|---|---|---|
Conditions | 0 | 1 | 2 | 3 | Total |
C | 0 | 3 | 5 | 19 | 27 |
OA | 0 | 3 | 2 | 9 | 14 |
W | 0 | 0 | 2 | 12 | 14 |
OAW | 0 | 1 | 1 | 26 | 28 |
. | Gonadal stage . | . | |||
---|---|---|---|---|---|
Conditions | 0 | 1 | 2 | 3 | Total |
C | 0 | 3 | 5 | 19 | 27 |
OA | 0 | 3 | 2 | 9 | 14 |
W | 0 | 0 | 2 | 12 | 14 |
OAW | 0 | 1 | 1 | 26 | 28 |
Abbreviations: C—control (18°C, ambient pH), OA—ocean acidification (18°C, − 0.33 pH unit), W—warming (21°C, ambient pH), and OAW—ocean acidification and warming (21°C, − 0.33 pH unit).
Number of adult oysters at the end of the conditioning period for each gonadal stage determined according to the reproductive scale of (Steele and Mulcahy 1999) and according to the four conditions.
. | Gonadal stage . | . | |||
---|---|---|---|---|---|
Conditions | 0 | 1 | 2 | 3 | Total |
C | 0 | 3 | 5 | 19 | 27 |
OA | 0 | 3 | 2 | 9 | 14 |
W | 0 | 0 | 2 | 12 | 14 |
OAW | 0 | 1 | 1 | 26 | 28 |
. | Gonadal stage . | . | |||
---|---|---|---|---|---|
Conditions | 0 | 1 | 2 | 3 | Total |
C | 0 | 3 | 5 | 19 | 27 |
OA | 0 | 3 | 2 | 9 | 14 |
W | 0 | 0 | 2 | 12 | 14 |
OAW | 0 | 1 | 1 | 26 | 28 |
Abbreviations: C—control (18°C, ambient pH), OA—ocean acidification (18°C, − 0.33 pH unit), W—warming (21°C, ambient pH), and OAW—ocean acidification and warming (21°C, − 0.33 pH unit).
Effects of temperature and pH changes on neurochemistry in adult oysters
The levels of all parent neurotransmitters, i.e. dopamine, glutamate, GABA, noradrenaline, and 5-HT, known as neuroendocrine regulators of physiological functions in bivalves, were not significantly affected by temperature and pH changes (Figure 5A–E). There was a significant pH effect on 5-HIAA concentrations, which were higher in the acidified conditions (OA, OAW) than in the other treatments (Figure 5F); while this effect was marginally significant on the 5-HIAA/5-HT ratio (Figure 5G). In addition, there was a significant reduction of this ratio under warmer conditions (W, OAW; Figure 5G).
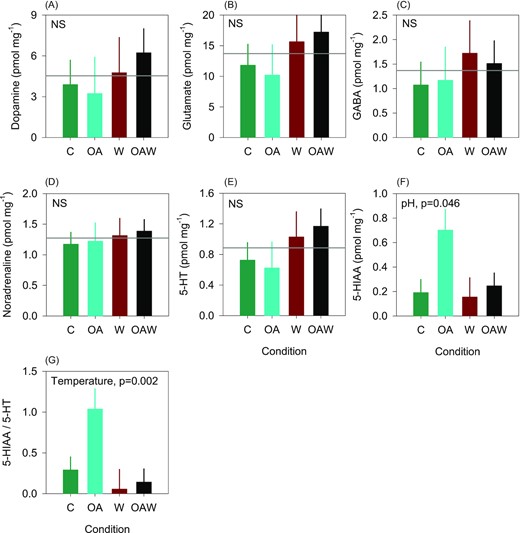
Concentration of parent neurotransmitters (A–F) in visceral ganglia of adult oysters, and 5-HT turnover expressed by the 5-HIAA/5-HT ratio (G). Data are least-square means ± SE among tanks (n = 3 or 6). Effects and p-values are shown when significant otherwise the mean is shown by the grey line when non-significant (NS). Abbreviations: 5-HT = serotonin or 5-hydroxytryptamine; 5-HIAA = 5-Hydroxyindoleacetic acid; C—control (18°C, ambient pH), OA—ocean acidification (18°C, −0.33 pH unit), W—warming (21°C, ambient pH), and OAW—ocean acidification and warming (21°C, −0.33 pH unit).
Effects of temperature and pH changes on gamete quality in adult oysters
Oocyte concentration, diameter, and fatty acid content, a proxy of total lipid reserves, were similar among treatments (Figure 6A–C). Increasing temperature decreased the unsaturation index of fatty acids and increased levels of both arachidonic (AA, 20:4n−6) and eicosapentaenoic acids (EPA, 20:5n−3, Figure 6D–F). Decreasing pH slightly decreased the level of docosahexaenoic acid (DHA, 22:6n−3) irrespective of temperature (Figure 6G). Temperature rise increased spermatozoa concentrations in oysters but not motility rate and velocity (Figure 6H–J). Fertilization rates were similar among treatments and were always higher than 90% under all conditions (data not shown).
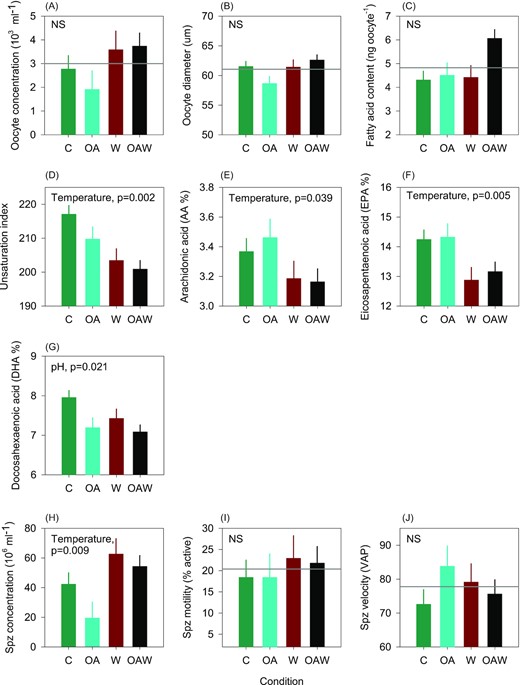
Quality of gametes produced by conditioned adult oysters. For eggs (A–G): concentration, diameter, total fatty acid content, unsaturation index, and essential fatty acid concentrations (AA, EPA, DHA). For spermatozoa (H–J): concentration, motility, and velocity. Data are least-square means ± SE among tanks (n = 3 or 6). Effects and p-values are shown when significant otherwise the mean is shown by the grey line when non-significant (NS). Abbreviations: C—control (18°C, ambient pH), OA—ocean acidification (18°C, −0.33 pH unit), W—warming (21°C, ambient pH), and OAW—ocean acidification and warming (21°C, −0.33 pH unit).
Effects of temperature and pH changes on life traits of progenies
Hatching rate, an indicator of successful embryogenesis, varied almost two-fold within each treatment, but there was no consistent pattern with temperature, pH, or parental conditioning history (Figure 7A). Although marginally significant, we found that larvae produced from parents exposed to OA during gametogenesis and then maintained under OA for 48 h were the smallest (Figure 7B). Other indicators such as birefringence level, a proxy of shell calcification, lipid reserves, and swimming behaviors (i.e. velocity) were unaffected by lowered pH and increased temperature (Figure 7C–I). Interestingly, offspring facing multistress (OAW), either directly during the larval period or via parental exposure, were less mobile, although these results were marginally significant (Figure 7J).
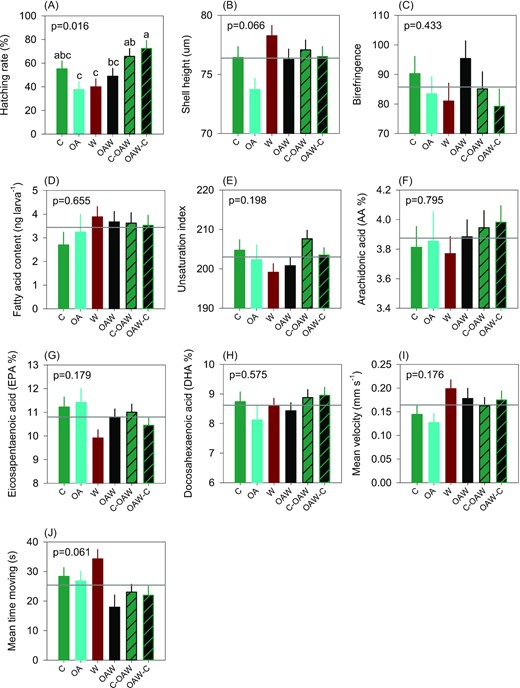
Hatching rate, shell height, birefringence (greyscale score), total fatty acid content, and essential fatty acid concentration (A–H), as well as velocity and motility (I and J). Data are least-square means ± SE among tanks (n = 3 or 6). Effects and p-values are shown when significant otherwise the mean is shown by the grey line when non-significant (NS). Abbreviations: C—control (21°C, ambient pH), OA—ocean acidification (21°C, −0.33 pH unit), W—warming (24°C, ambient pH), and OAW—ocean acidification and warming (24°C, −0.33 pH unit).
Discussion
Here, we investigated the direct effects of OAW on life-history traits of the Pacific oyster C. gigas. We also tested whether intergenerational carryover effects occurred, i.e. whether parental conditioning history shaped the phenotypic characters of the first-generation progenies. Adults were exposed to −0.33 pH unit relative to ambient seawater conditions at two temperatures during a complete reproductive cycle, and the phenotype of the offspring was evaluated at 48 h post-fertilization. Throughout conditioning, we monitored standard parameters in adults, such as growth, food intake, and sexual maturity, as well as components of the neuroendocrine system, and of gamete and larval quality (e.g. energy reserves and behavior) that have rarely been appreciated in OA studies. We found that near-future OA exposure during gametogenesis reduced growth rate and activated the serotonergic system in adult oysters, but increasing temperature buffered these effects. Other macro-physiological parameters were however not affected by temperature and pH, and we did not find any evidence of intergenerational linkage. All together, our results suggest that C. gigas is robust to near-future ocean changes.
Near-future OA reduces adult oyster growth but increasing temperature suppresses this effect
We observed that adult oyster growth, measured as shell length and total body weight, was significantly reduced under OA at a pH offset of −0.33 unit. These results contrast with what we recently observed on juveniles of the same species, i.e. the shell length and growth rate remained unchanged until a tipping point was reached at ca. pH 7.10, below which these parameters were strongly affected (Lutier et al., 2022). The overall reduction in oyster growth cannot be attributed to shell dissolution because seawater was not corrosive in our study with respect to the saturation state of calcite (Ωca > 1), the main carbonate mineral composing the adult oyster shell (Stenzel, 1963). Rather, this may reflect a decrease in calcification rate, which declined linearly with lowering pH and CO32− concentrations in C. gigas (Gazeau et al., 2007). However, as previously reported (Clark et al., 2013; Timmins-Schiffman et al., 2014), there was no statistical difference for shell weight. In fact, since growth was limited due to the relatively short experimental period, the newly formed shell was probably negligible relative to the whole shell weight.
Adult oyster growth was reduced under near-future OA but food intake was unaltered. The results are consistent with previous data showing that ingestion of juvenile oysters remained unchanged up until reaching pH 6.85 where it decreases thereafter (Lutier et al., 2022). Thus, oysters may not be physiologically responsive to the pH reduction considered in our study because they were fed ad libitum. Thus the reduced growth rate of oysters under OA was probably not an energetic issue but rather a reflection of a less favourable carbonate environment.
Interestingly, we found that increasing temperature suppressed the negative effects of OA on oyster growth. Oysters are ectothermic animals, for which temperature is one of the dominant environmental determinants that controls physiological performances (Bayne, 2017). It is therefore possible that the growth benefit of the temperature increase tested, which was within the optimal thermal window for the species, outweighed the deleterious effects of OA on individual fitness. However, this result contrasts with other studies conducted on clams and scallops that report additive negative effects of these two factors on growth, survival, and biomineralization (Talmage and Gobler, 2011; Watson et al., 2012; Ivanina et al., 2013). These contradictory results pinpoint the need for conducting more studies combining stressors in OA experiments (Riebesell and Gattuso, 2015) and for increasing knowledge on thermal tolerance of organisms to enhance our prediction capacity of species sensitivity to climate change.
Near-future OA and temperature rise have no effect on sex determination and reproductive effort, but temperature accelerates gonadal maturity
We did not observe any effect of pH and temperature, alone or in combination, on sex ratio and gonadic coverage of oysters, an indicator of reproductive effort (Fabioux et al., 2005). In contrast, in the eastern oyster C. virginica, pH reduction between 7.5 and 7.7 (−0.2 to −0.4 pH offset) increased gonadic coverage in both sexes (Clements et al., 2021) and gonadal development was delayed at pH ≤ 7.1 and inhibited at pH 6.7 (Boulais et al., 2017). In the Sydney rock oysterSaccostrea glomerata, pH reduction between 7.4 and 7.0 (−0.4 pH offset) delayed gonadal development and favoured females (Parker et al., 2018). The scientific literature reveals varying and conflicting results within and between species thus preventing generalizations.
We note, however, that sexual ripeness was reached earlier at the higher temperature. It is well known that temperature is one major driver of reproduction in C. gigas (Fabioux et al., 2005). For instance, we know that a minimum accumulation of 600°C days above ∼10°C induces spawning (Mann, 1979). Therefore, increasing seawater temperature will favour accelerated sexual maturity and earlier spawning events with possible impacts on species distribution and phenology (Gourault et al., 2019; King et al., 2021).
Temperature rise, but not OA, influences gamete characteristics
Temperature did not influence the quantity and size of the oocytes, but their fatty acid content. Indeed, the unsaturation index of fatty acids, an indicator of lipid fluidity, decreased with increasing temperature, as predicted by homeoviscous adaptation (Hazel, 1995; Pernet et al., 2007). This change in unsaturation index mainly reflected 20:5n-3, as previously reported in other bivalve species (Pernet et al., 2007). Therefore, the fatty acid composition of oocytes changes with temperature consistently with what is generally reported in other tissues. Spermatozoa concentration was enhanced in warmer conditions, whereas no change in their motility was observed. It seems that in the case of the oyster, maturation at 21°C may enhance fertilization success compared to 18°C. It is however noteworthy that the two temperatures tested in our study were within the optimal temperature window for fertilization in C. gigas (20–25°C; Bayne, 2017). While it is admitted that temperature is an important factor influencing sperm quality, an increase in temperature beyond the species optimal is generally unfavourable to sperm movement and energy metabolism. Indeed, prolonged exposure to high temperatures, unsuitable for reproductive activity in the mussel Mytilus galloprovincialis, significantly reduced the concentration of spermatozoa and furthermore disrupted their motility and mitochondrial activity (Boni et al., 2016).
In addition, we found that OA had no major effect on gamete quality. In most of the published studies, broodstock were maintained under ambient conditions and gametes are generally acutely exposed to low-pH treatments. In such conditions, a pH lowering (−0.3 to −0.4 pH offset) did not induce significant effects on sperm velocity and fertilization success in oysters and mussels (Kurihara et al., 2007, 2008; Havenhand and Schlegel, 2009; Barros et al., 2013; Falkenberg et al., 2019). In contrast, stronger pH lowering (−0.7 pH offset) substantially reduced spermatozoa motility and fertilization success in oysters (Kurihara et al., 2007, 2008; Barros et al., 2013; Boulais et al., 2017, 2018). To our knowledge, only one study has examined the effects of acidified conditions throughout the conditioning of C. gigas on sperm quality (Omoregie et al., 2019). In contrast to our study, these authors showed that a moderate pH reduction (−0.4 pH offset) reduced sperm motility in a Namibian C. gigas population. Such discrepancies in intraspecific responses may reflect effects related to the study populations or experimental conditions other than pH (e.g. food regime).
Near-future OA enhances serotonin response but increasing temperature counteracts this effect
We did not observe changes in the levels of parent neurotransmitters induced by pH and temperature, even for GABA, which is the major neurotransmission system known to be affected by OA in fish (Nilsson et al., 2012; Chivers et al., 2014; Schmidt et al., 2017). However, the 5-HIAA content, the 5-HT primary metabolite, was significantly increased in oysters exposed to OA, as was the 5-HIAA/5-HT ratio, although marginally significant. In bivalves, the serotonergic system modulates reproduction through gametogenesis, spawning induction, and sperm motility (Alavi et al., 2017a, 2017b; Canesi et al., 2022). However, we observed no effect of OA on oyster reproduction, suggesting that the observed change in 5-HIAA under OA may be associated with other biological functions. In particular, the serotonergic system is mobilized in C. gigas when exposed to acute and chronic stressors such as air exposure and chemical pollution (Dong et al., 2017; Canesi et al., 2022). Since our study is the first reporting such effects of pH on serotonergic system, it may be premature to state that OA evoked a stress response in oysters.
However, we evidenced that OA could affect 5-HT activity by presumably increasing 5-HT catabolism rather than 5-HT synthesis since 5-HT levels remained similar between conditions. In addition, it is worth noting that warming significantly reduced 5-HT turnover for all animals, including those exposed to OA, suggesting an antagonistic interaction between the drivers. To our knowledge, there are no comparable studies on the effects of environmental changes on the neuroendocrine systems in marine molluscs. Interestingly, recent papers showed that near-future changes in pH and thermal regimes affect serotonin metabolism in three fish species (Salmo salar, Gadus morhua, and Boreogadus saida) leading to a rise of the 5-HIAA/5-HT ratio (Schmidt et al., 2017; Höglund et al., 2020), with no apparent interaction between the drivers (Schmidt et al., 2017). Unlike these species whose geographical distribution is limited to the North Atlantic, the Pacific oyster is cosmopolitan and has a wide thermal optimum (Bayne, 2017) making it tolerant to a +3°C rise.
Overall, our results are of great importance because the serotonergic system is a major neuroendocrine actor mediating various physiological functions in oysters (Alavi et al., 2017b; Canesi et al., 2022). We therefore believe that the use of neuroendocrine indicators is promising in the field of climate change research, particularly the serotonergic system, which has been identified as a relevant proxy to reveal sublethal effects of OA in fish (Höglund et al., 2020). Thus, further investigations will be conducive to better understand the role of the 5-HT system in the physiology of C. gigas in order to tease the pace of individual acclimation to environmental change.
Near-future temperature rise and OA do not affect early development
When parents and offspring were kept under the same conditions, there was no significant effect of pH and temperature on larval quality (hatching rate, shell height, calcification, fatty acid content, and composition). We also found that a direct multistressor exposure (OAW) during early development, with or without prior parental exposure, had no effect on larval quality. Overall, the lack of a significant effect of near-future OA is consistent with what has already been reported in C. gigas larvae when exposed directly during embryogenesis (Kurihara et al., 2007; Barros et al., 2013; Ginger et al., 2013). Collectively, these results contrast with the findings of Harney et al. (2016) showing that OA significantly reduces larval size while warming increased both shell size and calcification level. In the latter study, the authors showed a nonadditive effect of these two drivers on larval size and proteome (Harney et al., 2016). Furthermore, our exposure conditions did not alter the swimming behavior of C. gigas larvae, which is consistent with previous observations conducted in mollusc larvae exposed to OA in combination or not with warming, such as in the Eastern oyster C. virginica at settlement (Meyer-Kaiser et al., 2019) and in the abalone Haliotis tuberculata (Kavousi et al., 2022).
Moreover, we did not observe any intergenerational carryover effects between adult and offspring at haching (i.e. 48 h post-fertilization). The quality of larvae originating from parents matured in the OAW condition and then maintained under control condition, and vice versa, were not different from those kept in the fixed OAW or control conditions throughout the experiment. In several bivalve species, carryover effects under OA are generally positive, i.e. parental exposure to OA confers on the offspring, better growth and faster development at decreasing pH from hatching, and this benefit persists throughout the larval life (Parker et al., 2012, 2015; Zhao et al., 2019) and into adulthood (Parker et al., 2015). Although in C. virginica, there is no indication of carryover effect, adult OA conditions did not modify early larval development 48 h after fertilization (Clements et al., 2021).
Interestingly, in several oyster species, when OA is combined with increased temperature, the carryover effect is unnoticeable at haching (Gibbs et al., 2021) or even negative later in the development (Parker et al., 2017). Our results are consistent with the studies by Clements et al. (2021) and Gibbs et al. (2021), which show no carryover effect in early larval development. Note, however, that the 48-h timeframe was short and we cannot rule out that carryover effects may arise later during development as suggested in C. gigas by Gibbs et al. (2021). So-called “latent carryover” effects (Pechenik, 2006; Hettinger et al., 2013), typically described when the susceptibility of juveniles to environmental stress is influenced by previous larval experience, needs to be further investigated in the context of parental effects. Although intergenerational and developmental plasticity is increasingly being considered as acclimation mechanisms in bivalves to cope with climate change, there may be limitations and differences among species. Therefore, further investigations demonstrating whether or not effects are transferred across life phases, the strength and direction of responses, and their persistence, are needed to provide a more complete understanding of acclimatory capacity of oysters.
Apparent robustness of C. gigas to climate change
Molluscs are generally considered as vulnerable to OA because of their dependance to the availability of carbonate ions to produce their shell combined with their low capacity to regulate internal acid–base status (reviewed in Melzner et al., 2009; Gazeau et al., 2013; Parker et al., 2013). Here, we suggest that the oyster C. gigas is robust to near-future OA and warming during reproduction and early development. This can be explained by the fact that this species is (1) eurythermic and the temperature levels tested here are within the thermal optimum of the species (Bayne, 2017) and (2) intertidal and thus adapted to hypercapnia and internal acidosis (Scanes et al., 2017; Parker et al., 2021). This agrees with the fact that physiological traits such as growth, net calcification, and ingestion tipped to much lower pH—between 7.3 and 6.9—than those tested here (Lutier et al., 2022).
Nevertheless, the experimental conditions may have masked some effects. Oysters were fed ad libitum and we know that food availability mitigates the impacts of OA on marine calcifiers (Melzner et al., 2011; Thomsen et al., 2013; Ramajo et al., 2016). Also, the pH of the acidified seawater was lowered by 0.3 unit but remained noncorrosive regarding calcite saturation level (Ωca > 1). In fact, it seems that larval shell development and growth are dependent on seawater saturation state, and not on pH (Gazeau et al., 2011; Waldbusser et al., 2015).
To go further, we need to consider the natural variability of pH/pCO2 experienced by the oysters prior the experiments. A recent study shows that the effect of a given pH/pCO2 scenario depends on the deviation from the upper level of pCO2 experienced locally by the populations studied (Vargas et al., 2022). This deviation is not known in our study and could explain our results. This requires the implementation of pH/pCO2 monitoring along the coastline and in oyster farms to better ground the experiments. We must also consider the importance of local biological adaptation by integrating several populations of oysters across continents and various genetic lines with different life-history traits. These repeating experiments are needed to fully understand and tease apart any differences due to experimental procedures from genetic/phenotypic differences, which may alter conclusions about the impacts of climate change and the capacity for intergenerational plasticity in oysters.
Supplementary data
The following supplementary material is available at ICESJMS online:
File S1 describes the production and maintenance of broodstock used in the experiment.
File S2 describes the methodology and protocols applied in this study.
File S3 provides the full statistical output.
File S4 is the data repository.
ACKNOWLEDGEMENTS
We thank the staff at Ifremer Argenton and Bouin for animal production and maintenance. We are grateful to Marc Suquet and Nelly Le Goïc for gamete quality analyses, Isabelle Queau for probe metrology and oyster biometry, Dominique Ratiskol for phytoplankton production, Matthias Huber and Jacqueline Legrand for zootechnical support and Claudie Quéré for lipid analyses. We thank our collaborator Stéphanie Auzoux-Bordenave (MNHN/CNRS/SU/IRD, BOREA, Station marine de Concarneau) for her help with the birefringence analyses. Finally, we thank Raphaël Delépée, manager of the PRISMM core facility (UNICAEN SF4206 ICORE, Comprehensive Cancer Centre F. Baclesse, Normandie University, Caen, France), for the development and validation of the quantification of neurotransmitters in oyster nervous tissue. We are also grateful to Gilles Le Moullac for project conception and funding.
Funding
This work was supported by the Ocean Acidification Program of the French Foundation for Research on Biodiversity (FRB—www.fondationbiodiversite.fr), the French Ministère de la Transition Ecologique, and the LabexMER Frontiers in marine research (www.labexmer.eu).
Conflict of interest
The authors declare no conflict of interest.
Author contributions
All authors designed and conducted the experiment. FP performed statistics and figures. CDP and FP wrote the paper and all authors contributed and accepted it. FP and CDP obtained the funding.
Data availability statement
The data underlying this article are available in the article and in its online supplementary material (File S4).