-
PDF
- Split View
-
Views
-
Annotate
-
Cite
Cite
Dali Geng, Xiaoxia Shen, Yinpeng Xie, Yusen Yang, Ruiling Bian, Yuqi Gao, Pengmin Li, Liying Sun, Hao Feng, Fengwang Ma, Qingmei Guan, Regulation of phenylpropanoid biosynthesis by MdMYB88 and MdMYB124 contributes to pathogen and drought resistance in apple, Horticulture Research, Volume 7, 2020, 102, https://doi.org/10.1038/s41438-020-0324-2
- Share Icon Share
Abstract
MdMYB88 and MdMYB124 have been demonstrated to be responsible for lignin accumulation in apple under drought stress. In this study, using a metabolomic approach, we identified differentially accumulated phenylpropanoid and flavonoid metabolites in MdMYB88/124 transgenic RNAi plants under control and long-term drought stress conditions in apple roots. We confirmed the regulation of phenylalanine by MdMYB88 and MdMYB124 via UPLC-MS in apple roots under both control and drought conditions. Using Electrophoretic Mobility Shift Assay (EMSA) and ChIP-quantitative PCR (qPCR) analyses, we found that MdMYB88 positively regulates the MdCM2 gene, which is responsible for phenylalanine biosynthesis, through binding to its promoter region. Under long-term drought conditions, MdMYB88/124 RNAi plants consistently accumulated increased amounts of H2O2 and MDA, while MdMYB88 and MdMYB124 overexpression plants accumulated decreased amounts of H2O2 and MDA. We also examined the accumulation of metabolites in the phenylpropanoid biosynthesis pathway in the leaves of MdMYB88 and MdMYB124 transgenic apple plants after long-term drought stress. We found that metabolites responsible for plant defense, including phenylpropanoids and flavonoids, accumulated less in the RNAi plants but more in the overexpression plants under both control and drought conditions. We further demonstrated that MdMYB88/124 RNAi plants were more sensitive to Alternaria alternata f. sp. mali and Valsa mali, two pathogens that currently severely threaten apple production. In contrast, MdMYB88 and MdMYB124 overexpression plants were more tolerant to these pathogens. The cumulative results of this study provided evidence for secondary metabolite regulation by MdMYB88 and MdMYB124, further explained the molecular roles of MdMYB88 and MdMYB124 in drought resistance, and provided information concerning molecular aspects of their roles in disease resistance.
Introduction
Previous research has shown that two atypical and paralogous MYB transcription factors, namely, MdMYB88 and MdMYB124, are responsible for freezing and drought stress tolerance of apple plants1,2. In addition, MdMYB88 and MdMYB124 can also regulate the biosynthesis of lignin under drought stress by directly targeting MdMYB46 and MdVND6, two important regulators of the biosynthesis of secondary cell wall components2. In plants, lignin biosynthesis occurs via the phenylpropanoid biosynthesis pathway3; therefore, it is compelling to know whether MdMYB88 and MdMYB124 regulate lignin biosynthesis via the regulation of phenylpropanoid biosynthesis. Additionally, whether the biosynthesis of other secondary metabolites is regulated by MdMYB88 and MdMYB124 is important to further understand their roles in stress responses.
The phenylpropanoid biosynthesis pathway begins with phenylalanine, which can be converted into aromatic compounds, including benzenoids, coumarins, flavonoids, hydroxycinnamates, and lignin4. These compounds often participate in plant development5 and plant-environment interactions6. Phenylalanine is synthesized from the shikimate pathway, which requires the participation of chorismate mutase (CM)4,7. In Petunia hybrida cv. Mitchell, two CMs at different loci are directly responsible for the biosynthesis of phenylalanine7,8. In addition, the expression level of CMs can substantially influence the accumulation of phenylalanine, which further affects the biosynthesis of phenylpropanoids in plants9–13.
Many phenylpropanoids and flavonoids are involved in the responses to oxidative stress14–16, drought stress17, and disease resistance18–21. In Vitis vinifera, genes related to phenylpropanoid metabolism have been found to be less induced and/or more repressed in drought-sensitive rootstocks22. Research has also shown that phenylpropanoids and flavonoids play critical roles in the response to ozone-induced oxidative stress, as relatively high contents of phenylpropanoids and flavonoids in Fraxinus excelsior L. lead to relatively low-ozone sensitivity23. In addition, phenylpropanoids play a prominent role in the response to drought-induced oxidative stress in Platanus × acerifolia. The great investment of Platanus × acerifolia in phenylpropanoid biosynthesis complements the decrease in insufficient amounts of antioxidants during drought stress24. Phenylpropanoids and flavonoids also participate in the response to Alternaria alternata f. sp. mali. Higher contents of phenylpropanoids and flavonoids can enhance the resistance of tomatoes to A. alternata25. The defense against Valsa mali in plants also requires phenylpropanoids and flavonoids26. It has been shown that transgenic MdUGT88F1 (a key UDP-glucose:phloretin 2’-O-glucosyltransferase gene) apple plants with increased phloridzin have enhanced resistance to Valsa canker27.
Abiotic and biotic stresses, including drought and disease, have been threatening apple growth and production worldwide. Drought often results in reduced photosynthesis and solute transport, loss of turgidity, membrane lipid peroxidation, and other problems in apple27. Membrane lipid peroxidation during drought stress always leads to secondary oxidative stress28. An increase in the levels of reactive oxygen species (ROS), such as hydrogen peroxide (H2O2), is the most direct indicator of oxidative stress, and malondialdehyde (MDA) is the product of membrane lipid peroxidation28. Disease caused by A. alternata f. sp. mali and Valsa mali affect apple trees by infecting the leaves and young shoots and subsequently causing defoliation, which leads to premature fruit drop29–32. A number of studies have characterized genes important for the resistance of apple to drought and pathogens and have examined their potential use in the breeding of resistant apple33–37. MYB88 and MYB124 and their homologous genes in apple participate in the response to salt, cold, and drought stress1,2,38–41. In the current study, we revealed that MdMYB88 and MdMYB124 regulate the accumulation of metabolites involved in the phenylpropanoid pathway by directly regulating the expression of MdCM2, which contributes to pathogen defense and drought stress tolerance.
Results
MdMYB88 and MdMYB124 regulate secondary metabolite accumulation in apple roots
Previously, we determined that MdMYB88 and MdMYB124 regulate root lignin deposition under drought stress. Here, we carried out a secondary metabolic profiling analysis to identify additional secondary metabolites regulated by MdMYB88 and MdMYB124 under both control and drought conditions. We used an ultra-performance liquid chromatography-tandem mass spectrometry (UPLC-MS) method in conjunction with previously generated MdMYB88/124 RNAi plants in which both MdMYB88 and MdMYB124 were knocked down1. The results showed that a total of 678 metabolites were detected with this approach. Among these metabolites, 58 were flavone metabolites, 158 were flavonoid metabolites (including 13 catechins and catechin derivatives), 15 were anthocyanins and anthocyanin derivatives, 33 were flavonols, 29 were flavonoid glycosides, 19 were flavanones, 19 were quinic and quinic derivatives, and 17 were coumarins and coumarin derivatives (Supplementary Table 1).
We first applied principal component analysis (PCA) and orthogonal partial least squares discriminant analysis (OPLS-DA) on these metabolites to discover the differences in metabolites within and between sample groups. The PCA and OPLS-DA results showed that the metabolites of different genotypes and treatments were significantly different and could be used for further analysis (Supplementary Fig. 1A, B). We then performed a normalized clustering analysis and Pearson's correlation analysis to analyze the homogeneity of the biological replicates of each group (Supplementary Fig. 1C, D). The second biological replicate of GL-3 under the control conditions was removed due to a relatively low coefficient (<0.9).
To determine the differentially regulated metabolites, we performed a normalized clustering analysis (Fig. 1). Under the control conditions, 33 metabolites were found to be upregulated in the MdMYB88/124 RNAi plants compared to those in the GL-3 plants. Furthermore, 89 metabolites were found to be downregulated in the MdMYB88/124 RNAi plants compared to those in the GL-3 plants under the control conditions. Under drought conditions, 40 metabolites were found to be upregulated in the MdMYB88/124 RNAi plants compared to GL-3 plants, and 99 metabolites were found to be downregulated. These results suggest that MdMYB88 and MdMYB124 regulate a broad range of secondary metabolites.
Heat map of differentially regulated metabolites
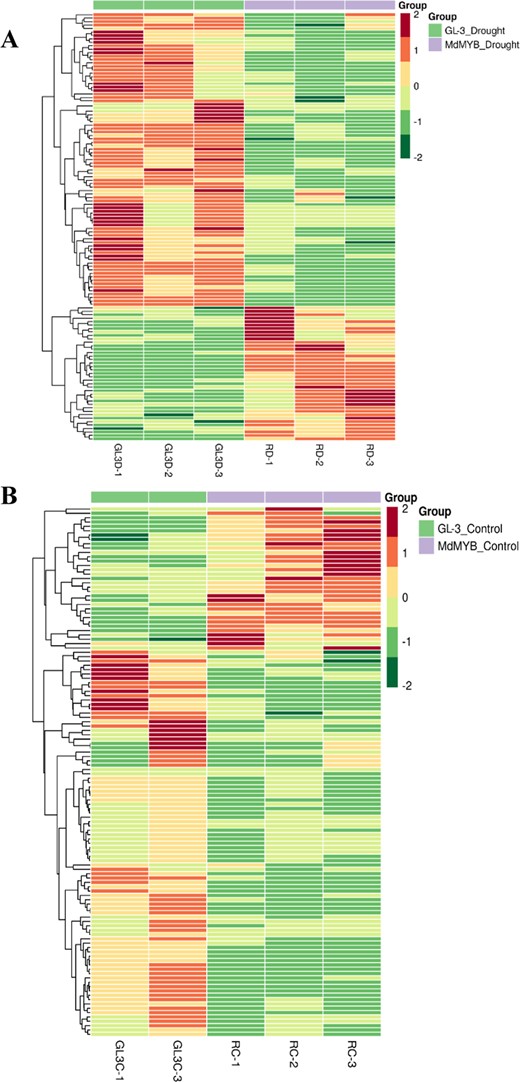
Heat map of differentially regulated metabolites in GL-3 and MdMYB88/124 RNAi plants under long-term drought stress conditions (a) and control conditions (b)
KEGG analysis was used to annotate the differentially regulated metabolites in the pathways of phenylpropanoid biosynthesis, flavonoid biosynthesis, and flavone and flavonol biosynthesis (Fig. 2 and Supplementary Figs. 2–4). All the differential metabolites in these three pathways were found to be downregulated in the MdMYB88/124 RNAi plants under drought conditions compared to those in the GL-3 plants (Fig. 2). These metabolites included 15 involved in the phenylpropanoid biosynthesis pathway, 10 in the flavonoid biosynthesis pathway, and 7 in the flavone and flavonol biosynthesis pathway (Supplementary Figs. 2–4). We also discovered that the contents of 10 differential metabolites were downregulated in the MdMYB88/124 RNAi plants under the control conditions compared to those in the GL-3 plants (Supplementary Figs. 2–4).
Differentially regulated metabolites related to the phenylpropanoid and flavonoid biosynthesis pathway under drought
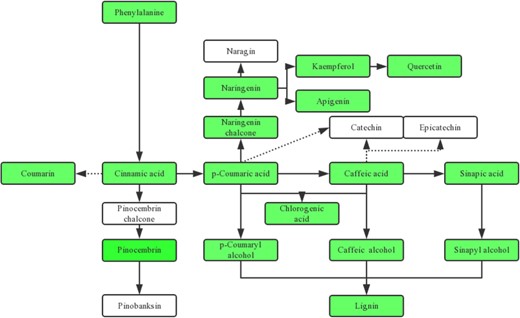
The green color indicates metabolites downregulated in MdMYB88/124 RNAi lines compared with those in GL-3 after long-term drought stress, and the white color represents metabolites that did not change in MdMYB88/124 RNAi lines compared with GL-3 under drought stress
MdMYB88 and MdMYB124 regulate the phenylalanine content in the roots
The KEGG analysis revealed a large number of differential metabolites related to the phenylpropanoid, flavonoid, flavone and flavonol biosynthesis pathways after drought stress (Fig. 2). Additionally, all these metabolites were downregulated in the roots of MdMYB88/124 RNAi lines under drought (Supplementary Table 1); however, under control conditions, a smaller number of differential metabolites were discovered (Supplementary Table 1). These results indicate that MdMYB88 and MdMYB124 play a crucial role in the regulation of the phenylpropanoid, flavonoid, flavone, and flavonol biosynthesis pathways.
Furthermore, the content of phenylalanine in MdMYB88/124 RNAi plants decreased under the control and drought conditions when compared with that in GL-3 plants (Supplementary Table 1). We therefore hypothesized that the downregulation of phenylpropanoids is the result of phenylalanine downregulation. Hence, we verified the content of phenylalanine in the roots of the MdMYB88/124 RNAi lines and GL-3 under both drought and control conditions by the use of UPLC-MS (Fig. 3). Our results showed that the contents of phenylalanine in the roots of the MdMYB88/124 RNAi lines were lower than those in GL-3 under both the control and long-term drought conditions. Alternatively, the phenylalanine content was higher in the roots of the overexpression lines than in GL-3 under both the control and drought conditions (Fig. 3). These findings were consistent with our metabolome results.
Content of phenylalanine in the roots of MdMYB88 and MdMYB124 transgenic plants after long-term drought
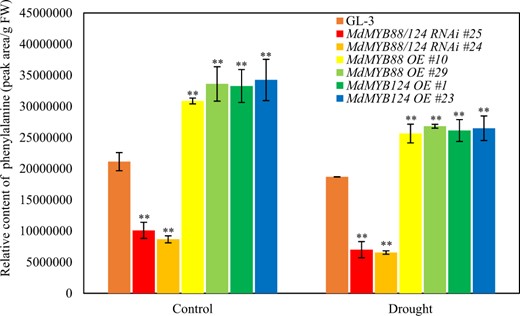
The data are the means ± SDs (n = 3). One-way ANOVA (Tukey test) was performed, and statistically significant differences are indicated by **(P < 0.01). OE overexpression
The differential content of phenylalanine is interesting. Biosynthesis of phenylalanine in plants occurs through the shikimic acid pathway. An essential reaction in phenylalanine biosynthesis is the conversion of chorismic acid into prephenic acid, catalyzed by CMs9. Three CMs have been discovered in Arabidopsis42, and six homologs in Malus x domestica were identified: MdCM1, MdCM1-like, MdCM2, MdCM2-like, MdCM3, and MdCM3-like (Supplementary Fig. 5). Sequence analysis of the MdCMs revealed a very high similarity between MdCM1 and MdCM1-like, between MdCM2 and MdCM2-like, and between MdCM3 and MdCM3-like. We also noted that MdCM1 is located on chromosome (chr.) 9, and MdCM1-like is located on chr. 16; MdCM2 is located on chr. 5, and MdCM2-like is located on chr. 8; MdCM3 is located on chr. 17, and MdCM3-like is located on chr. 13. The following pairs have homologous segments: chr. 9 and chr. 16, chr. 5 and chr. 8, and chr. 17 and chr. 1343. These results indicate that MdCM1 and MdCM1-like, MdCM2 and MdCM2-like, and MdCM3 and MdCM3-like are homologs from chromosome doubling.
The expression level of MdCMs in the roots of MdMYB88 and MdMYB124 transgenic lines treated with 20% PEG was analyzed by quantitative reverse transcription PCR (qRT-PCR) to determine whether MdCMs were regulated by MdMYB88 and MdMYB124 (Fig. 4). We found that all six homologs were downregulated in MdMYB88/124 RNAi lines and unregulated in MdMYB88 or MdMYB124 overexpression lines under control and PEG treatment conditions. Promoter analysis revealed the binding site of both MdMYB88 and MdMYB124 (AACCG) in the MdCM2 promoter (Supplementary Fig. 6). Using ChiP-qPCR and Electrophoretic Mobility Shift Assay (EMSA) approaches, we further showed that MdMYB88 can directly bind to the MdCM2 promoter (Fig. 5).
Relative expression levels of MdCMs in MdMYB88 and MdMYB124 transgenic plants under drought stress

Relative expression levels of MdCM1 (a), MdCM1-like (b), MdCM2 (c), MdCM2-like (d), MdCM3 (e), and MdCM3-like (f) in MdMYB88 and MdMYB124 transgenic plants under control and drought conditions. The data are the means ± SDs (n = 3). OE overexpression
MdMYB88 and MdMYB124 directly target the MdCM2 promoter
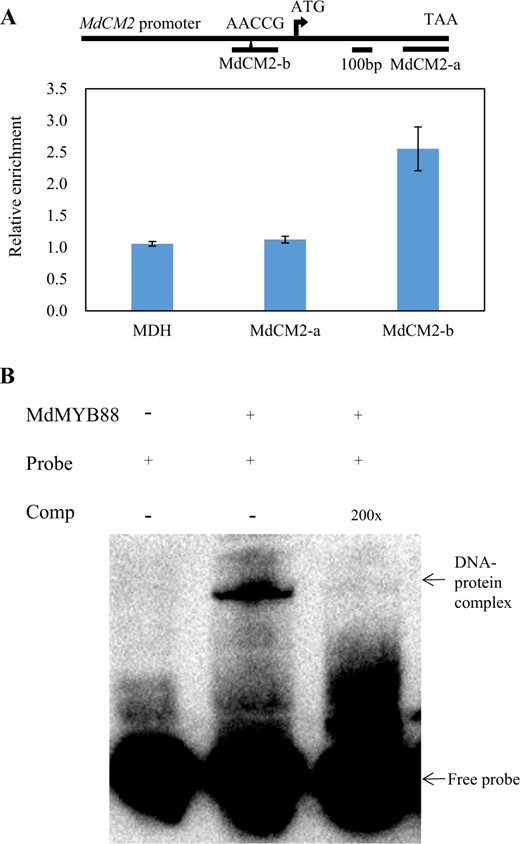
a ChiP-qPCR analysis of MdCM2 using a native antibody against MdMYB88 and MdMYB124. Fragment MdCM2-a served as a negative control. Fragment MdCM2-b contains a cis-element of AACCG. The data are the means ± SDs (n = 3). b EMSA analysis of MdMYB88-His binding to the promoter region of MdCM2. The arrowheads indicate protein-DNA complex or free probe
MdMYB88 and MdMYB124 regulate the content of different metabolites in the leaves
MdMYB88 and MdMYB124 are also expressed in leaves1. We therefore examined the accumulation of different metabolites, including flavonoids and phenylpropanoids, in the leaves of the GL-3 and MdMYB88/124 RNAi transgenic plants under both control and drought conditions via a UPLC-MS approach. Among the ten metabolites measured, the contents of chlorogenic acid, catechinic acid, quercetin, and SA (salicylic acid) decreased in the MdMYB88/124 RNAi lines and increased in the MdMYB88 or MdMYB124 overexpression lines (Fig. 6). We found no significant differences in the metabolites, including gallic acid, phlorizin, rutin, and caffeic acid (Supplementary Fig. 7).
Content of phenylpropanoids in the leaves of MdMYB88 and MdMYB124 transgentic lines under drought stress
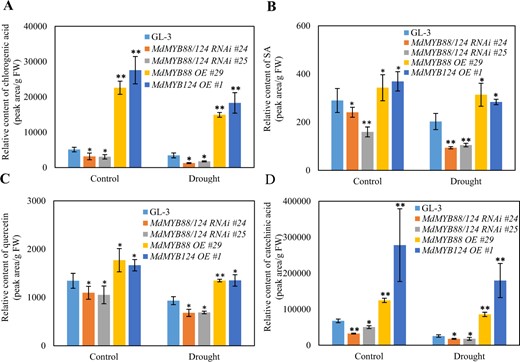
Content of chlorogenic acid (a), SA (b), quercetin (c), and catechinic acid (d) in the leaves under control and long-term drought stress conditions. The data are the means ± SDs (n = 3). One-way ANOVA (Tukey test) was performed, and statistically significant differences are indicated by *(P < 0.05) and **(P < 0.01)
Different contents of metabolites affect the occurrence of oxidative products and the resistance of pathogens
Flavonoid and phenylpropanoid metabolites have well-documented relationships with oxidative stress and pathogen resistance44. Drought stress is also well known to cause secondary stress, including oxidative stress38. We then analyzed the content of hydrogen peroxide (H2O2) and malondialdehyde (MDA) in both the roots and leaves of GL-3, MdMYB88 and MdMYB124 transgenic plants to examine the occurrence of drought-induced oxidative products. The results showed that there was no difference in the content of H2O2 or MDA between the GL-3 and transgenic lines under control conditions. There was, however, a significant increase in oxidative products in the MdMYB88/124 RNAi lines and a decrease them in the MdMYB88 or MdMYB124 overexpression plants under drought stress (Fig. 7).
Content of H2O2 and MDA in MdMYB88 and MdMYB124 transgentic lines under drought stress

Content of H2O2 (a and b) and MDA (c and d) in the roots (a and c) and leaves (b and d) under control and drought stress conditions. The data are the means±SDs (n = 5). One-way ANOVA (Tukey test) was performed, and statistically significant differences are indicated by *(P < 0.05)
Flavonoid and phenylpropanoid metabolites are part of a plant's defense system against pathogenic infection. The different contents of flavonoid and phenylpropanoid metabolites lead to differences in disease resistance45. We infected leaves using A. alternata and Valsa mali to evaluate the disease resistance of GL-3, MdMYB88, and MdMYB124 transgenic plants in vitro. Our results demonstrate that the leaves of the MdMYB88/124 RNAi lines were sensitive to A. alternata, while the leaves of the MdMYB88 and MdMYB124 overexpression lines were resistant to A. alternata compared with those of GL-3 (Fig. 8). We observed similar patterns in the leaves infected with Valsa mali (Fig. 9).
MdMYB88 and MdMYB124 positively regulate resistance to Alternaria alternata f. sp. mali
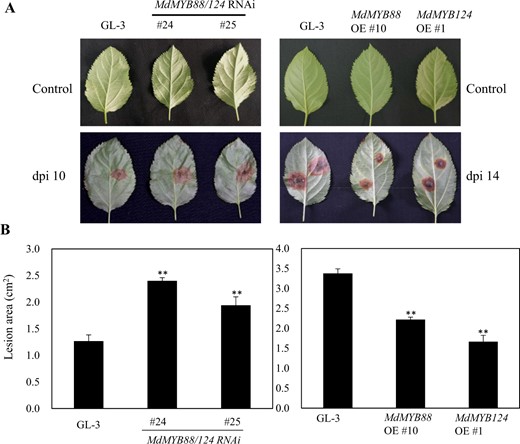
a Disease resistance of GL-3, MdMYB88/124 RNAi, MdMYB88, and MdMYB124 overexpression plants (OE). b Quantification of the data shown in a. The data are the means ± SDs (n = 8). One-way ANOVA (Tukey test) was performed, and statistically significant differences are indicated by **(P < 0.01)
MdMYB88 and MdMYB124 positively regulate resistance to Vasla mali
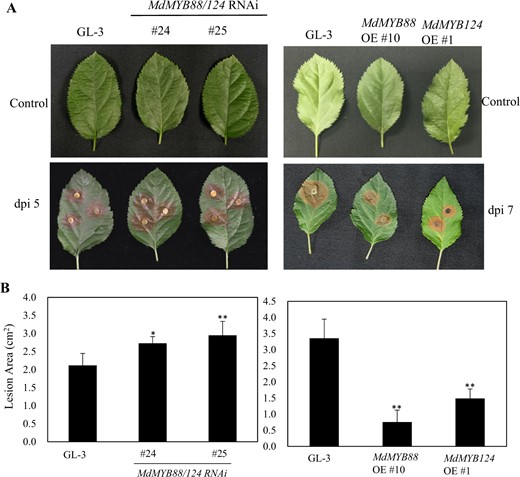
a Disease resistance of GL-3, MdMYB88/124 RNAi, MdMYB88, and MdMYB124 overexpression plants (OE). b Quantification of the data shown in a. The data are the means ± SDs (n = 8). One-way ANOVA (Tukey test) was performed, and statistically significant differences are indicated by *(P < 0.05) or **(P < 0.01)
Discussion
In previous studies, we presented evidence that MdMYB88 and MdMYB124 regulate both root vessel development and lignin deposition under drought stress in apple trees2. We also determined that MdMYB88 and MdMYB124 regulate the expression of genes related to lignin biosynthesis by associating with the MdMYB46 promoter2. Lignin is a metabolic compound produced by the phenylpropanoid and flavonoid biosynthesis pathway46. In this study, we performed a metabolomic analysis of the roots of GL-3 and MdMYB88/124 RNAi plants under control and drought conditions to understand the metabolic map of MdMYB88 and MdMYB124 and their regulatory roles in the disease resistance of apple trees.
The metabolome analysis revealed that more differentially accumulating metabolites were discovered in the roots of MdMYB88/124 RNAi lines after drought treatment compared to those after the control treatment. A total of 46 different phenylpropanoid and flavonoid metabolites were discovered in the roots of MdMYB88/124 RNAi plants under the control treatment, while 56 differential phenylpropanoid and flavonoid metabolites were discovered in the MdMYB88/124 RNAi plants under the drought treatment (Supplementary Table 1). The majority of different phenylpropanoid and flavonoid metabolites were downregulated in MdMYB88/124 RNAi plants compared to those in the GL-3 plants (Fig. 2). Furthermore, the content of lignin precursors (including p-coumaryl alcohol, coniferyl alcohol, and sinapyl alcohol) decreased in MdMYB88/124 RNAi plants (Fig. 2). A decrease in the content of all three precursors can lead to a decreased lignin content in MdMYB88/124 RNAi plants, which is consistent with our previous study2.
Many flavonoid metabolites, including chlorogenic acid47, quercetin48, kaempferol49, and pinocembrin50, are involved in a plant defense responses to fungal and bacterial infections. Derivatives of chlorogenic acid and chlorogenic acid can inhibit the growth of fungi in vivo and in vitro51,52. In response to fungal infection, chlorogenic acid in an apple plants can be hydrolyzed into 4-hydroxybenzoic acid, which has high antifungal activity, thus increasing the resistance of apple trees to fungi53. In immature peach trees and peach trees resistant to Monilinia fructicola, the chlorogenic acid content is exceptionally high54. Moreover, in vanilla plants resistant to Fusarium oxysporum, the sinapic acid and hydroxybenzoic acid content is higher than that in sensitive varieties55. Here, we discovered that these metabolites were positively regulated by MdMYB88 and MdMYB124, thus indicating that MdMYB88 and MdMYB124 may be involved in fungal resistance. We consistently found that the MdMYB88/124 RNAi plants were more sensitive to A. alternata infection than were the other plants, while the MdMYB88 and MdMYB124 overexpression plants were more tolerant (Figs. 8 and 9).
The phenylpropanoid biosynthetic pathway starts with phenylalanine. CM2 is a critical enzyme involved in phenylalanine biosynthesis42. Herein, we determined that the expression of MdCM2 and other MdCMs was positively regulated by MdMYB88 and MdMYB124 (Fig. 4). In addition, MdMYB88 and MdMYB124 could directly bind to the MdCM2 promoter region (Fig. 5). These results revealed that MdMYB88 and MdMYB124 directly regulate MdCM2. However, we did not find any binding sites of MdMYB88 and MdMYB124 in the promoters of other MdCMs, although MdMYB88 and MdMYB124 positively regulate their expression level. These findings indicate that MdMYB88 and MdMYB124 may regulate other MdCMs through an unknown pathway.
In plants, different phenylpropanoid and flavonoid metabolite contents lead to a difference in the ability of plants to scavenge reactive oxygen species (ROS)56. In this study, the H2O2 and MDA contents were higher in MdMYB88/124 RNAi plants under drought stress than in GL-3 plants; however, there was no significant difference between the GL-3 and the MdMYB88 and MdMYB124 transgenic lines under the control conditions (Fig. 6). These results indicate that the flavonoid content in the MdMYB88 and MdMYB124 transgenic lines influences the plants’ ROS scavenging abilities, which may further contribute to the drought tolerance of apple trees.
In summary, MdMYB88 and MdMYB124 can directly target and induce the expression of MdCM2 and positively regulate other MdCMs. Upregulated MdCM2 and other MdCMs enhance phenylalanine biosynthesis, thus increasing the content of free phenylalanine in both the roots and the leaves. The increased phenylalanine content affects the biosynthesis of downstream metabolites, including phenylpropanoid and flavonoid compounds, which results in increased resistance to oxidative stress and fungal infection.
Methods
Plant materials, growth conditions, and stress treatment
Tissue-cultured GL-3 [from Malus x domestica “Royal Gala” seedlings with high regeneration capabilties57], transgenic MdMYB88/124 RNAi and MdMYB888 overexpression or MdMYB124 overexpression plants were rooted and transplanted into pots (30 cm × 18 cm) filled with equal parts of local loess sand and worm cast media. The pots were placed in a greenhouse under natural illumination, with a temperature of 20–35 °C and a humidity of 35–55%. MdMYB88/124 RNAi plants were generated previously1. Owing to the high sequence similarity, both MdMYB88 and MdMYB1241 were knocked down in the MdMYB88/124 RNAi plants1.
Long-term drought was implemented according to the methods described by Geng et al.2. After drought stress, the roots were washed and harvested for UPLC-MS analysis.
For disease resistance analysis, GL-3 and MdMYB88 or MdMYB124 transgenic plants were propagated in MS culture media containing 0.2 mg/l 6-benzylaminopurine and 0.2 mg/l indole-3-acetic acid. After 1 month, the transgenic plants were transferred to rooting media containing 0.5 mg/l 3-indolebutyric acid and 0.5 mg/l indole-3-acetic acid. After 45 days, the plants were transplanted into soil and into a growth chamber with a 16/8-h light/dark photoperiod. After ~1 month, the apple plants were ready for the infection experiments.
Disease resistance analysis
The plant pathogens A. alternata and Valsa mali were isolated previously58. The fungi were grown on PDA (potato dextrose agar) media at 25 °C for ~6 days. Fifteen to twenty leaves of GL-3 and MdMYB88 or MdMYB124 transgenic plants were collected and washed with ddH2O twice, soaked in 6% sodium hypochlorite solution in the dark for approximately 5 min and then rewashed with ddH2O. The leaves were then infected using bacterial plaque obtained by a hole puncher to maintain consistency. After infection, the leaves were returned to the culture dishes and placed in an incubator (70% humidity; 25–28 °C) for two weeks. Afterward, images were taken, and the leaf spots were measured by ImageJ software. The experiments were repeated three times, and one-way ANOVA (Tukey test) was performed by SPSS 19.0.
UPLC-MS analysis
UPLC-MS analysis was performed according to the methods of Chen et al.59, with modifications. Freeze-dried roots were crushed using a mixer mill (MM 400, Retsch, German) with silica sand for 2 min at 60 Hz. One hundred milligrams of powder was then weighed and extracted overnight at 4 °C with 1.0 ml of 70% aqueous methanol. Following centrifugation at 10,000 × g for 10 min, the extracts were absorbed (CNWBOND Carbon-GCB SPE Cartridge, 250 mg, 3 ml; Anpel, Shanghai, China) and filtered by a filter membrane with a pore size of 0.22 μm. The extracts were then analyzed using an Liquid chromatography electrospray ionization tandem mass spectrometry (LC-ESI-MS/MS) system. The analytical conditions were as follows: UPLC column, Waters ACQUITY UPLC HSS T3 C18 (1.8 µm, 2.1 mm × 100 mm); solvent system, water (0.04% acetic acid):acetonitrile (0.04% acetic acid); gradient program, 95:5 v/v at 0 min, 5:95 v/v at 11.0 min, 5:95 v/v at 12.0 min, 95:5 v/v at 12.1 min, and 95:5 V/Vv/v at 15.0 min; flow rate, 0.40 ml/min; temperature, 40 °C; and injection volume, 2 μl. The effluent was alternatively connected to an ESI-triple quadrupole-linear ion trap (QTRAP)-MS. LIT and triple quadrupole (QQQ) scans were acquired by a API 6500 QTRAP LC/MS/MS triple quadrupole-linear ion trap mass spectrometer (QTRAP) system. The ESI source operation parameters were the same as those in the study by Chen et al.59. PCA and Pearson correlation analysis were performed by SPSS 19.0. OPLS-DA, discovery and KEGG annotations were performed by the R package.
Measurements of H2O2 and MDA content
The contents of H2O2 and MDA were measured by a hydrogen peroxide assay kit (#BC3590, Solarbio, Beijing, China) and a malondialdehyde assay kit (#BC0020, Solarbio, Beijing, China), respectively. Five repeats were measured for each line.
RNA extraction and RT-qPCR analysis
RNA extraction was carried out as described by Xie et al.1. RT-qPCR analysis was performed according to the methods of Guan et al.60. The primers used are listed in Supplementary Table 2.
EMSAs and ChIP-qPCR
EMSAs and ChIP-qPCR were performed as described by Xie et al.1. The probes used for the EMSAs are listed in Supplementary Table 2.
Acknowledgements
We thank Dr. Zhihong Zhang from Shenyang Agricultural University for providing the tissue-cultured GL-3 plants. We thank Dr. Lili Huang from Northwest A&F Agricultural University for providing Valsa mali. This work was supported by the National Key Research and Development Program of China (2018YFD1000100) and the National Natural Science Foundation of China (31622049, 31872080, and 31660565).
Conflict of interest
The authors declare that they have no conflict of interest.
References
Author notes
These authors contributed equally: Dali Geng, Xiaoxia Shen.
Supplementary data
supplemental figures
supplemental table 1