-
PDF
- Split View
-
Views
-
Cite
Cite
S.-Å. Elming, M. S. D'Agrella-Filho, L. M. Page, E. Tohver, R. I. F. Trindade, I. I. G. Pacca, M. C. Geraldes, W. Teixeira, A palaeomagnetic and 40Ar/39Ar study of late precambrian sills in the SW part of the Amazonian craton: Amazonia in the Rodinia reconstruction, Geophysical Journal International, Volume 178, Issue 1, July 2009, Pages 106–122, https://doi.org/10.1111/j.1365-246X.2009.04149.x
- Share Icon Share
Summary
A new key palaeomagnetic pole (Plat. = 64.3°S, Plon. = 271.0°E, N = 14, A95 = 9.2°; Q = 5) is calculated from a primary magnetization isolated in early Neoproterozoic Aguapei basic sills and dykes hosted by 1.3–1.0 Ga sedimentary rocks from the southwestern part of the Amazon craton. The characteristic remanence carried by stable, pseudo-single domain titanomagnetite shows two antipodal polarities that pass a reversals test. Magnetic anisotropy for most sites shows fabric orientations that are typical of sills, with horizontal magnetic foliations concordant to the flat-lying bedding of the host sedimentary rocks. 40Ar/39Ar analyses for one of the sills reveal a well-defined plateau age at 981 ± 2 Myr. A tectonic reconstruction for Amazonia relative Laurentia based on this new pole ‘is consistent with’ a position of the present northwestern part of Amazonia attached with eastern Laurentia close to Greenland at ca. 981 Ma. On basis of palaeomagnetic and geological data, we propose a scenario where Amazonia moved northeastwards along the present southeast coast of Laurentia from ca. 1200 to 980 Ma. By 980 Ma, Amazonia is placed alongside Laurentia and Baltica, in a position similar to other reconstructions of Rodinia but with a significantly different orientation.
Introduction
The assembly of continents into a late Precambrian supercontinent has been suggested in a number of studies (e.g. Piper 1976; McMenamin & McMenamin 1990; Dalziel 1991; Hoffman 1991; Dalziel et al. 2000). The Rodinia hypothesis is widely acknowledged in the literature, although the configuration and timing of breakup of this supercontinent are still widely debated. The geometry of Rodinia is mostly modelled on the basis of ca. 1350–1000 Ma orogenic belts and tectonic links between mobile belts across cratonic margins. As palaeomagnetism is the only tool for reconstructing latitudinal positions and orientations of the cratons, it plays a key role for the reconstruction of Rodinia. However, reliable palaeomagnetic data are sparse for many of the continents during the time interval of assembly and breakup of the supercontinent, which reduces the possibility of testing proposed configurations (e.g. Buchan et al. 2001; Pesonen et al. 2003; Meert & Lieberman 2004). The Amazonian craton forms an important part in the Rodinia reconstruction, and distinct tectonic models of relations with Laurentia and Baltica have been suggested (e.g. Priem et al. 1989; Dalziel 1991, 1992; Hoffman 1991; Sadowski & Bettencourt 1996; D'Agrella Filho et al. 1998; Dalziel et al. 2000). However, the number of palaeomagnetic poles from Amazonia is very limited, and the database for palaeomagnetic tests is, thus, restricted to results from ca. 1.2 Ga gabbros and basalts of the Nova Floresta Formation (Tohver et al. 2002) and sedimentary rocks of the Aguapéi Group, southwestern Amazonia (D'Agrella-Filho et al. 2008), which suggests a juxtaposition with Laurentia along its present Grenville margin.
In our study of basic sills and dykes from SW Amazonia, we present new, reliable palaeomagnetic data from the early Neoproterozoic Grenville interval. We use these new data to test the models of a long-lasting tectonic relation between Amazonia, Laurentia and Baltica. Our findings indicate that the southwestern part of Amazonia may have been attached to eastern Laurentia (Greenland) at ca. 980 Ma.
Geology And Sampling
The Amazonian Craton is composed of an Archaean nucleous and Paleoproterozoic accretionary belts that young successively to the SW (e.g. Cordani & Teixeira 2007): the 1.8–1.6 Gyr old Rio Negro-Juruena province (Fig. 1a) is bordered towards the SW by the 1.5–1.3 Gyr old Rondonian-San Ignacio province and the ca. 1.3–0.9 Gyr old Sunsás province that forms the SW margin of the craton. The Sunsas belt sensu strictu has long been considered a counterpart of the Grenville belt in the tectonic reconstructions between Amazonia and Laurentia (e.g. Hoffman 1991). The Sunsas province, according to some models, comprises three separate belts: the 1.05–1.1 Gyr old Sunsas belt proper of west-central Bolivia that forms the southern border of the Paragua craton (Litherland et al. 1989; Boger et al. 2005); the intracratonic Aguapeí belt where ca. 1.15 Gyr old sediments were deformed at ca. 950 Ma (Saes 1999; Saes & Leite 1993; Ruiz 2005) and the 1.1 Gyr old Nova Brasilândia metasedimentary belt that forms the northern margin of the Paragua craton and is interpreted as a suture zone with the Amazon metaigneous basement to the north (Tohver et al. 2004a; Tohver et al. 2005a). An alternative approach (Cordani & Teixeira 2007) considers the Sunsas orogenic belt as restricted to the southern bounds of the Paraguá craton, structurally marked by shear and mylonitic zones, granitoid rocks and supracrustal sequences and reactivated basement rocks. The Sunsas belt originated in an extensional environment, consisting of a passive margin sedimentary sequence (the Vibosi and Sunsas belts) that was subsequently deformed during a continental collision and intruded by granitic suites accompanying extensive deformation along the tectonic zones, followed by the emplacement of post-tectonic plutons. According to Cordani & Teixeira (2007), the cratonic area in Brazil the Nova Brazilandia and the Aguapeí belts, dated at ca. 1160 Ma (Rizzotto et al. 2001; Santos 2003), were deformed in rift-type structures that later were affected by transpression and crustal shortening and granite intrusions due to the Sunsas collision. The related tectonic reactivation led to anorogenic magmatism (e.g. granites, sills and dykes) in the tectonically stable crust, such as the widespread anorogenic granitic magmatism of the Younger Granites of Rondonia, also referred as the Rondonia Tin Province (ages between 970 and 1100 Ma; Priem et al. 1989; Bettencourt et al. 1999), the coeval plutons in Mato Grosso as well as emplacement of the 990 Ma Rincon del Tigre mafic-ultramafic complex in Bolivia (Annels et al. 1986). Cordani & Teixeira (2007) therefore considered these intraplate tectonomagmatic events as tectonic reflections of the Sunsas orogeny over the stable foreland.
The Amazon basement itself was deformed earlier, 1.15–1.2 Ga, on basis of 40Ar/39Ar and feldspar thermometry data from a wide area of strike-slip shear active at temperatures of 450–550 °C (Tohver et al. 2005b).
The present work is focused in the Brazilian state of Mato Grosso, southeast of the 1.1 Gyr old suture zone, where Proterozoic basement rocks that crop out in the Jauru terrane are interpreted by Geraldes et al. (2001) to be a SE extension of the Rio Negro-Juruena province. The geology of the Mato Grosso region includes various rock types, with ages ranging from 1.80–1.75 Gyr for the Jauru basement to the 1.52–1.47 Gyr rocks of the Rio Alegre Complex (Fig. 1b). Intrusion of the Santa Helena batholith has been dated at 1.45-1.42 Gyr, using U-Pb analysis of zircon by Geraldes et al. (2004), who considered the Rio Branco intrusions to be coeval, mafic representatives of a bimodal suite. The Aguapei Group sediments comprise quartzose sandstone and conglomerates of the basal Fortuna Formation, overlain by pelitic rocks and fine lithic, sub-arkosic rocks of the Vale da Promissão Formation, topped by quartz sandstones of the Morro Cristalina Formation (Souza & Hildered 1980; Saes 1999). A deformational event folded and faulted these sedimentary rocks under lower greenschist conditions within the central portion of the ca. 50-km-wide Aguapei belt. The external margins of this deformed zone are found to the west along the Brazil-Bolivia frontier and to the east, close to the Brazilian town of Rio Branco, where nearly undeformed, subhorizontal layers with minimal metamorphism are observed (Fig. 1).
Basaltic dykes and sills are found intruding into the flat-lying Aguapei Group sediments, including the Fortuna Formation and Vale da Promissao Formation near the town of Rio Branco. The sills form 1 to 5 m thick beds that intrude parallel to the sedimentary bedding, with a mesocratic aspect ranging from dark grey to black. The grainsize ranges from fine to medium, and the composition varies from gabbro to quartz-monzodiorite (Ruiz 2005). Plagioclase (labradorite-andesine) occurs commonly in the matrix as tabular euhedral to subeuhedral crystals and less commonly as phenocrysts up to 5 cm. The primary mineralogy consists of plagioclase, hornblende, biotite, orthoclase, quartz and, rarely, olivine. Cumulative and ophitic textures are common, and the accessory minerals are represented by zircon, titanite, magnetite, ilmenite and pyrite. Geochemical data suggest that the sills are typical of intracratonic origin (Ruiz 2005). K-Ar dating on plagioclase and whole rock of the sills suggests ages between 1015 ± 17 and 875 ± 21 Myr (Barros et al. 1982; Ruiz 1992, 2005). Hornblende and biotite 40Ar/39Ar data from basement rocks preserve igneous crystalline ages, indicating a very low degree of exhumation (<5 km) for this region and the absence of high grade metamorphism during the Grenvillian interval (Tohver et al. 2006a). From the standpoint of paleomagnetism, this information signifies that this long-stable region has the potential to preserve ancient directions from the Grenvillian and pre-Grenvillian interval and has been part of the Amazon craton since at least ca. 1.15 Ga.
Methods
Palaeomagnetism
Basic sills have been sampled in 14 sites (Fig. 1b), using a portable rock drill. A total of 108 samples were collected and oriented with both sun and magnetic compasses. Standard alternating field (AF) and thermal demagnetization techniques were applied and the laboratory work was done in the Palaeomagnetic laboratories at the Universidade de São Paulo, Brazil, and Luleå University of Technology, Sweden. A Schonstedt TSD-1 and a magnetic measurements MMTD60 furnace (peak temperature within ±2 °C, heating time of 1 hr) were used for the stepwise thermal demagnetizations with AF demagnetization carried out in conjunction with use of the SQUID (2G-DC) magnetometer, which was also used to measure magnetic remanence. For samples with high NRM intensities, a Molspin Spinner magnetometer was used to measure remanent magnetization, and demagnetization was conducted using a Molspin AF-demagnetizer. At São Paulo, the instruments are housed in a magnetically shielded room with an ambient field <1000 nT. The anisotropy of magnetic susceptibility (AMS) was measured to define the magnetic fabric of the rocks using a Molspin Minissep instrument. The technique of Jelínek (1978) was used for the statistical analyses of the AMS data. Low field magnetic susceptibility variation with temperature was obtained using a Bartington (MS2W) instrument. The magnetic susceptibility was measured during continuous heating and cooling of specimens up to 700 °C. The Curie temperature determined from these experiments was compared with the unblocking temperatures determined from the thermal demagnetizations to identify the carriers of remanent magnetization. The hysteresis properties were measured using a Molspin Vibrating Sample Magnetometer (VSM) to determine the domain state of the remanence carriers.
Demagnetization results were analysed using orthogonal plots (Zijderveld 1967) and stereographic projections and the components of remanence were defined using principal component analysis (Kirschvink 1980). At least four demagnetization steps were used to calculate vectors in the orthogonal diagrams, and an upper limit for mean angular deviation (MAD) of 8° was used, although most vectors were very well defined with MAD generally <3°. The site and formation mean directions were calculated from AF data using Fisher statistics (Fisher 1953).
40Ar/39Ar dating
Palaeomagnetic core samples of basalt dykes and sills were used for 40Ar/39Ar analysis at the Geochronology laboratory at University of Lund, Sweden. The cores were crushed, cleaned and sieved to a 0.25–0.5 mm size fraction, and several milligrams of the whole rock material were irradiated together with the DRA-2 sanidine standard (25.26 Ma; Wijbrans et al. 1995, recalculated following Renne et al. 1998) for 35 hr at the NRG-Petten HFR RODEO facility in the Netherlands. J-values (the irradiation parameter) were calculated with a precision of 0.25 per cent. Since there is a high flux at the cadmium shielded facility at NRG-Patten, the irradiation time can be held relatively short. Frequently, an inhouse 980 Ma muscovite standard has been used to check the results from dating of rocks older than the sanidine standard. There is no indication of influence on the determined ages using the young standard.
The 40Ar/39Ar laboratory at the University of Lund employs a Micromass-5400 mass spectrometer with a Faraday cup and an electron multiplier. A metal extraction line, which contains two SAES C50-ST101 Zr-Al getters and a cold finger cooled to –155 °C by a Polycold P100 cryogenic refrigeration unit is also present. A few mg of the whole rock samples were loaded into a copper planchette that consists of several 3 mm holes. The samples were step-heated using a defocused 50W CO2 laser. Sample clean-up time was 5 minutes, using the two hot Zr-Al SAES getters and the cold finger. The laser was rastered over the samples to provide even heating of all grains. Time zero regressions were fitted to data collected from 10 scans over the mass range of 40–36. Peak heights and backgrounds were corrected for mass discrimination, isotopic decay and interfering nucleogenic Ca-, K- and Cl-derived isotopes. Isotopic production values for the cadmium lined position in the Petten reactor are 36Ar/37Ar(Ca) = 0.000270, 39Ar/37Ar(Ca) = 0.000699 and 40Ar/39Ar(K) = 0.00183. 40Ar blanks were calculated before every new sample and after every three sample steps. 40Ar blanks were between 5.0 × 10−16 and 3 × 10−16. Blank values for masses 36–39 were all less than 7 × 10−18. Blank values were subtracted for all incremental steps from the sample signal. Age plateaus were determined using the criteria of Dalrymple & Lanphere (1971), which specify the presence of at least three contiguous incremental heating steps with statistically indistinguishable ages and constituting greater than 50 per cent of the total 39Ar released during the experiment.
Results
Geochronology
40Ar/39Ar whole rock age analyses were performed on five samples (Table 1), three from site 8 and two from site 3. One of the samples from site 8 yield a plateau age of 981 ± 2 Myr (sample 8F2; Fig. 2), according to the criteria of Dalrymple & Lanphere (1971). We interpret the 981 Ma plateau age to represent the cooling age of the sill, as suggested by its primary mineralogy. Two other samples of the same site yield disturbed age spectra with a staircase pattern of increasing age from the initial fractions over the first 20 per cent of degassed 39Ar, suggestive of significant argon loss through diffusion (samples 8E3 and 8E4, Fig. 2). Pseudo-plateau ages obtained for these samples suggest a minimum age of 922 ± 2 and 908 ± 11 Myr, respectively. The two samples from site 3 (3C3 and 3C4) yield disturbed spectra with pseudo-plateau ages for these samples of 1035 ± 3 and 1025 ± 8 Myr (Fig. 2), respectively, thereby interpreted as minimum ages. The difference in minimum ages obtained for the sites suggests that the rock at site 3 is older than the rock at site 8.
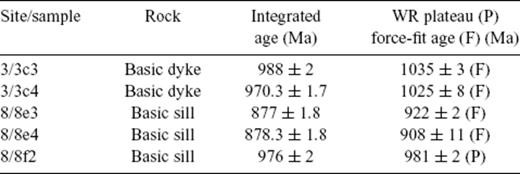
40Ar/39Ar data for the Aguapei sills and dykes, integrated and whole rock (WR) plateau force-fit ages.
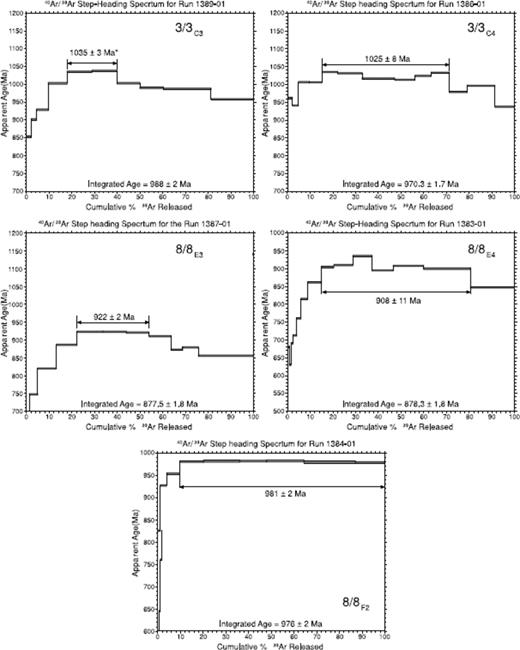
40Ar/39Ar age spectra from whole-rock basic dyke (3/3c3 and 3/3c4) and sill (8/8E3, 8/8E4 and 8/8F2). The samples are obtained from palaeomagnetic drill cores. See text for details.
Magnetic carriers
Thermal demagnetization reveals a range of unblocking temperatures (Tub) of ca. 530–550 °C (Fig. 3). However, a widely distributed Tub spectrum is observed for samples from nine of the sites, for which up to ∼70 per cent of the remanence is erased at temperatures below 525 °C. Susceptibility versus temperature measurements have been conducted on samples from all sites and the curves (Fig. 4) indicate Curie temperatures in the range 530–550 °C, suggesting titanomagnetite to be the magnetic carrier. The increase in susceptibility during cooling of samples from the site 12 could indicate titanomaghemite to be one of the magnetic minerals, as the heating-cooling curve for titanomagnetite (site 5) is expected to be reversible (Dunlop & Özdemir 1997).
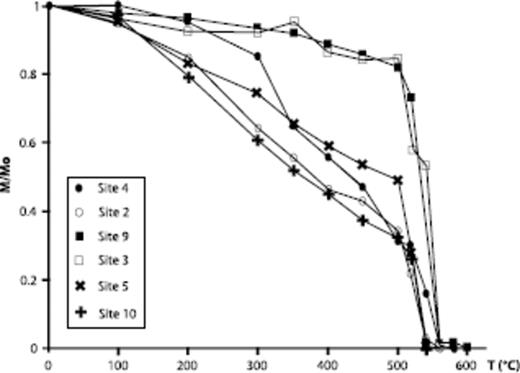
Examples of thermal demagnetization of sills and a basic dyke (site 3). The ratio M/M0 denotes the normalized intensity of remanent magnetization.
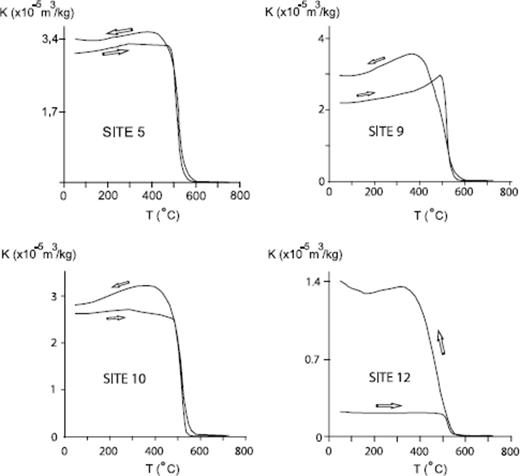
Thermomagnetic curves (susceptibility versus temperature) for sill samples from four sites. Note the increase in susceptibility during cooling of the sample from site 12, possibly indicating titanomaghemite to be one of the magnetic minerals.
The properties determined from the hysteresis measurements show a clear trend in the ratio of saturation remanent magnetization (Mrs) and saturation magnetization (Ms), Mrs/Ms, versus the ratio of coercivity of remanence (Hcr) and coercive force (Hc), Hcr/Hc. The diagram (Fig. 5) indicates grainsizes of the remanence carrying minerals trending from the transistion of single domain-pseudo-single domain (SD-PSD) to multidomain (MD) grains. However, the overall majority of samples plot well within the PSD part of the diagram (Day et al. 1977).
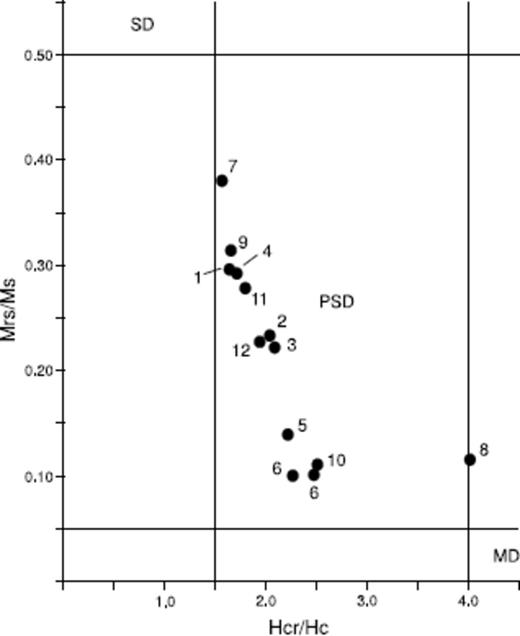
Hysteresis ratios measured for the sills and dykes and plotted in a Day-diagram (Day et al. 1977). Mrs, saturation remanent magnetization; Ms, saturation magnetization; Hc, coercive force; Hcr, coercivity of remanence; SD, single domain; PSD, pseudo single domain; MD, multidomain. The numbers refer to the site of the sample.
Anisotropy of magnetic susceptibility
The bulk mean magnetic susceptibility of the basic rocks varies from approximately 0.16 × 10−3 SI to 2.5 × 10−3, with the majority of site means higher than 1.0 × 10−3, suggesting a predominantly ferromagnetic origin of the susceptibility. The degree of anisotropy is less than 10 per cent for the rocks of our study (P< 1.08; Fig. 6), typical of igneous rocks with primary magnetic fabric (Hrouda 1982). The shape of the anisotropy ellipsoid is expressed by the shape parameter T (Jelinek 1981), for which a prolate ellipsoid is expressed by –1 < T < 0 and an oblate ellipsoid is expressed by 0 < T < 1. In most of the sites the T values are positive, indicating that the magnetic fabric of the studied rocks is predominantly oblate (Fig. 6).
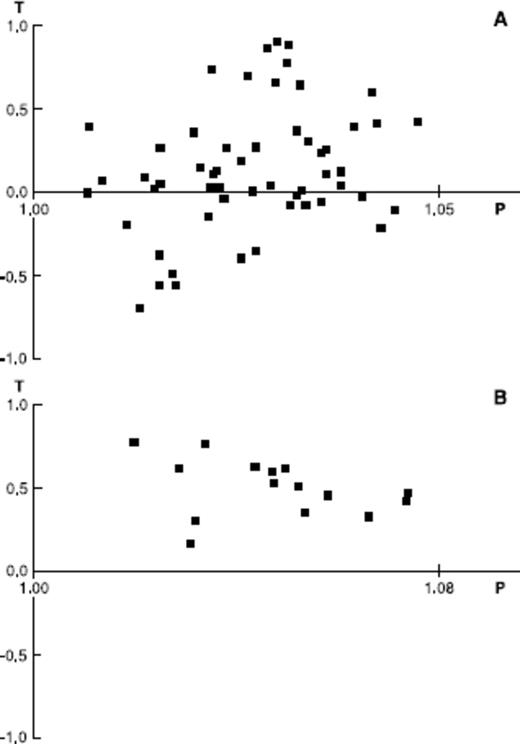
Data from anisotropy of magnetic susceptibility (AMS) plotted in a P–T diagram (Jelinek 1981) expressing the shape of the susceptibility ellipsoid. P denotes the degree of anisotropy. An oblate ellipsoid is expressed by 0 < T < 1, and a prolate ellipsoid is expressed by –1 < T < 0. A, Sills; B, Dykes. Note that for most samples, the magnetic fabric is oblate.
The majority of sites are characterized by kmin axes that cluster in subvertical directions (Fig. 7a). We interpret the AMS data as a primary flow indicator (Knight & Walker 1988; Tauxe et al. 1998), with the directions of kmax indicating the principal magmatic flow direction. The shallow, subhorizontal dip of the magnetic foliation planes suggests sill geometry for these rocks, with flow directions principally in the NE-SW and NW-SE directions. The AMS data from sites 1 and 2 reveal neither a typical sill nor a dyke pattern of the susceptibility with intermediate dips of the kmin axes.
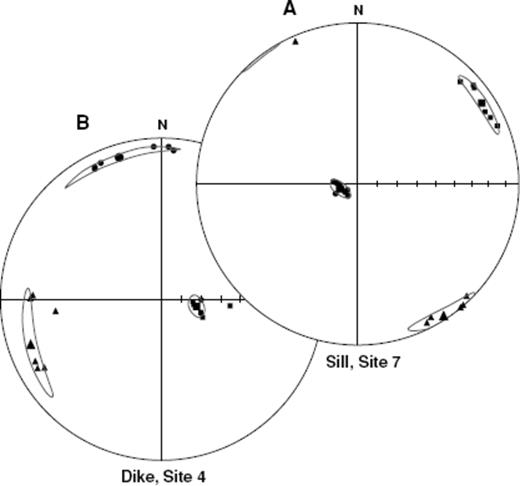
Examples of lower hemisphere equal area projection plots of directions of the maximum (▪) intermediate (▴) and minimum (•) susceptibility axes. The ovals are 95 per cent confidence limits about each axis of the site mean susceptibility ellipsoid. A typical plot for a sill is shown in panel (a), whereas a plot for a dyke is shown in panel (b).
In sites 3, 4 and 6, kmin axes are very shallow dipping ((Table 2), and two of them (3 and 4) are characterized by oblate magnetic fabrics (Figs 6b and 7b), indicating that these are dykes rather than sills. Contrary to the shallow magma flow directions that vary from between NE to NW, in the sills the magma flow direction (i.e., K1) in the dykes is subvertical. The foliation planes that express the dyke planes at sites 3 and 4 strike ENE (∼73°E; Fig. 8). The direction of the kmin axes (pole of the dyke plane) at site 6 suggests a dyke trend of 130°E. For sites 1 and 12, the directions of the kint and kmin form a girdle and the directions of the kmax axes are at dec. = 43°E, inc. = 26° and dec. = 320°E, inc. = 2°, respectively. The AMS patterns from the rock in sites 13 and 14 are more isotropic, and no preferred orientations of the axes can be defined. Aside from the light that these data shed on magmatic flow patterns, the AMS data suggest a tectonically undisturbed magmatic body, where the kmin directions for dykes, sills and flows approximate the pole of the flow plane (e.g. Knight & Walker 1988; Elming & Mattsson 2001). More importantly, the AMS data demonstrate that no tilt corrections are necessary for the paleomagnetic directions.
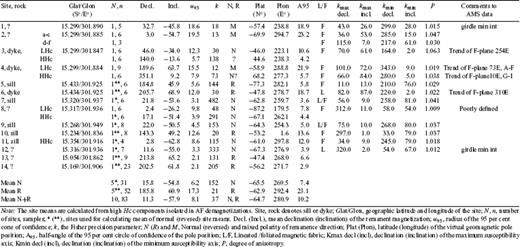
Palaeomagnetic and AMS data for basic sills and dykes in the Rio Branco area, southwestern Amazonia.
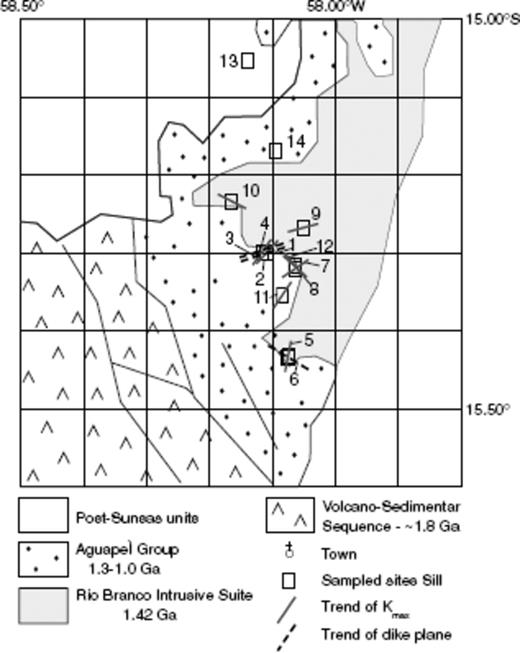
Site mean strike directions of the maximum susceptibility axes indicating flow directions in the sills and strike directions of the dyke planes defined from their magnetic foliation planes.
Palaeomagnetism
The remanent magnetization, apparently hosted by titanomagnetite and titanomaghemite, is commonly of high coercivity with median destructive fields (MDF) of the order of 15–30 mT (Fig. 9), supportive of a PSD behaviour of the remanent magnetization, which is inferred to be stable over geological time scales. The unblocking temperature (Tub) spectrum varies from wide to rather narrow (Figs 3 and 9). However, characteristic magnetizations are, in most cases, isolated in Tub of 500–580 °C.
Examples of remanence behaviour during progressive alternating field and thermal demagnetization for sites 3, 4, 5, 6 and 12. Open (closed) symbols in the vector plots denote vertical (horizontal) projections. Open (closed) symbols in the stereographic projections denote negative (positive) inclinations of the remanence vector.
In these basic sills, there are generally two components of magnetization. Thermal treatment does not completely eliminate the secondary magnetization even at temperatures close to the Curie temperature of magnetite-titanomagnetite, probably due to overlapping Tub spectra of the two components, which resolves only an intermediate direction. The characteristic magnetization is therefore best isolated by AF demagnetization.
The site mean directions are generally well defined with a precision parameter (k; Fisher 1953) generally higher than 40 (12 of 18 sites) and in the 100–500 range for 10 sites (Table 2).
Note that we exclude sites 1 and 2 from the calculation of formation mean because these sites give doubtful AMS-constrained orientations. The poor precision of the mean directions of characteristic magnetizations for these sites (α95 > 18° and k < 20) may reflect tectonic disturbances within the sites, in keeping with the anomalous AMS orientations.
For sites 3 and 4, two different components of magnetization are identified (Fig. 9), one of which isolated in low Hc and Tub ranges. This low Hc/Tub direction (Table 2) is not significantly different from normal and reversed directions of the sills but different from the expected dipolar field (dec. = 0°, incl. = –28.1°) and the International Geomagnetic Reference Field (IGRF: dec. = 345.8°, incl. = –12.4°). However, since this direction is carried by magnetizations with Hc and Tub of ≤10 mT and 500 °C, respectively, they may well be of a more recent origin, and these site means are not included in the calculation of the formation mean. The high Hc/Tub components are significantly different from the characteristic magnetizations of the sills (site 3, dec. = 140°, inc. = –13.6°; site 4, dec. = 351.1°, inc. = 9.2°). AMS data indicate that these rocks are subvertical dykes, and the 40Ar/39Ar pseudo-plateau ages (≥1035 Myr) indicate the dyke at site 3 to be older than the sills, although no good plateau age could be defined. These directions have no resemblance with the moderate to steep remanence directions obtained for the 1150 Ma (D'Agrella-Filho et al. 2008) and the 1200 Ma (Tohver et al. 2002) rocks in the region, and the only published remanence directions from Amazonia similar to those of sites 3 and 4 are from the ca. 1640 Myr old Rio Aro, Roraima and La Escalera basic dykes from the Venezuelan part of Amazonia (Hargraves 1968; Onstott et al. 1984), which were suggested to carry primary magnetizations. The disturbed age spectra of site 3 suggests that the dykes may have been reheated by the nearby sill, which resulted in an almost complete resetting of the argon system, although the temperature was not high enough to produce a magnetic overprint of the original magnetization.
The AMS data suggest that the rock at site 6 is a dyke with a direction of kmax indicating a subvertical magma flow. This dyke hosts a characteristic magnetization (high Hc and Tub) that falls into the group of reversed sill directions. We suggest that this dyke was emplaced (and magnetized) contemporaneously with the sills, that is, a feeder dyke for the sills. This may be supported by the almost perpendicular flow directions indicated for the sill at site 5, relative to the trend of the neighbouring dyke at site 6 (Fig. 8).
The samples from site 14 were collected from three different levels of a thick (10–15 m) section of the sill. The site mean directions from the three levels are well defined and not significantly different, suggesting a rapid cooling of the sill. The samples are therefore treated as collected from one single site.
The formation mean is, thus, calculated from the site means of sites 5–14. Dual polarities of the characteristic remanence are observed; a normal polarity direction with a well-defined mean of sites at dec. = 15.8°, inc. = –54.8° (α95 = 6.2°) and a reversed polarity direction with a less precise mean at dec. = 185.8°, inc. = 60.9° (α95 = 17.3°) (Fig. 10; Table 2).
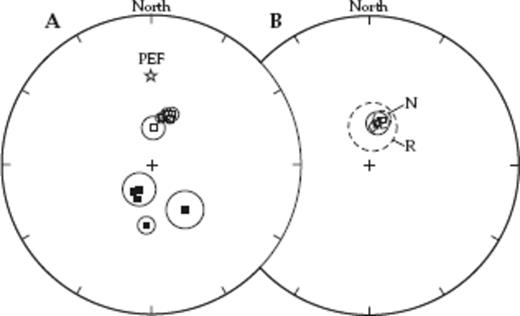
(a) Lower hemisphere equal area stereographic projection plot of site mean remanence directions isolated in the sills together with the 95 per cent cone of confidence. (b) The means of the normal (N, □) and reversed (R, ◊; here with polarity inverted to normal) site means are shown with hatched cones of confidence and the formation mean is shown with full drawn cone of confidence. Open (closed) symbols denote negative (positive) inclinations. PEF is the expected direction of the present dipole field.
Inverting the reversed polarity direction demonstrates that the mean directions of the two magnetic polarities are not significantly different, as seen from the overlapping cones of confidence (Fig. 10; McFadden & Lowes 1981). This fact justifies the calculation of a mean of site means, including both polarities (dec. = 11.3°, inc. = –57.9°, α95 = 8.1°). The positive reversal test for this result also signifies that the remanences were acquired over a substantial interval of geological time (>103 yr), enough to have averaged out the effects of secular variation. In addition, the preservation of a dual polarity result from unmetamorphosed igneous rocks suggests that the magnetic remanence was acquired when the rock formed. We argue that the characteristic magnetization of the sills is primary, and there seems to be no high temperature event in the region resetting the high Tub magnetizations of the sills and dykes. A palaeomagnetic pole position is calculated from the mean of the normal and reversed site mean directions at 280.9°E and 64.7°S (N = 10, A95 = 10.2°; Table 2). This palaeomagnetic pole position thus represents a primary magnetization with an Ar40/Ar39 age of ca. 981 Myr as determined from the sill at site 8. The pole has a quality factor (Q) of 5 (Van der Voo 1990), that is, (1) the rock is well dated; (2) the number of samples is sufficient and the statistical demands are fulfilled; (3) the samples have been adequately demagnetized; (4) there is a structural control and a tectonic coherence with the craton and (5) reversals have occured.
Discussion
There are very few Precambrian poles for the Amazonian shield. Tohver et al. (2002) presented a reliable 1200 Ma pole for Amazonia from a study of basaltic sills, the Nova Floresta Formation, in the western part of the Amazon craton. A new pole for 1150 Ma has recently been reported for the Amazon craton, based on a study of the Aguapeí Group (D'Agrella-Filho et al. 2008). These poles are the only poles for Amazonia in the interval 1200–800 Ma (see Tohver et al. 2006b). Therefore, our new pole is an important contribution to tectonic reconstructions of the Rodinia supercontinent, given the prominence of Amazonia in these reconstructions. To test models of Rodinia with respect to Amazonia, poles of similar age are needed from the three key cratons that are considered to be linked by Grenvillian belts: Amazonia; Laurentia and Baltica (e.g. Dalziel 1992, 1997; Hartz & Torsvik 2002; Tohver et al. 2002, 2006b; Meert & Torsvik 2003). Our pole dates from a time when the Grenvillian orogenesis had already peaked, followed by a long period (300–400 Ma) of tectonic quiescence, which ended with the opening of the Iapetus Ocean at the end of Precambrian times (Cawood et al. 2001; McCausland et al. 2007a).
Palaeomagnetic data have been shown to be consistent with such a connection (Tohver et al. 2002; D'Agrella et al. 2008); however, with a relative motion between the continents between 1200 and 1150 Ma. Isotopic evidence for Amazonian heritage in blocks of the present-day eastern margin of Laurentia supports this scenario of a mobile connection between these two cratons from ca. 1200 to 1150 Ma (e.g. Tohver et al. 2004b, 2006a). Because of the ongoing relative motion between the North and South American cratons during the Grenvillian epoch, palaeomagnetic tests must be made by comparison of individual poles for Amazonia and Laurentia for the 1200–980 Ma interval (Fig. 11), instead of comparing the apparent polar wander paths (APWPs) of the two continents. Reconstructions based on this technique are not geometrically unique, but they avoid the assumption of a fixed relative geometry.
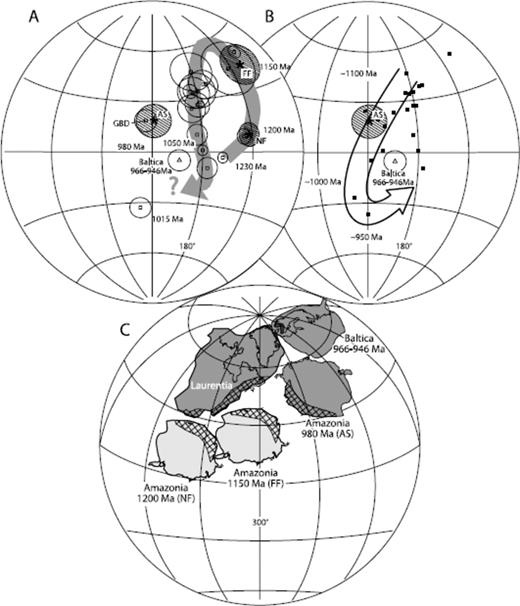
(a) The 1230–1015 Ma apparent polar wander path traced for Laurentia is shown as a shaded loop based on the poles listed in (Table 3. Poles NF, FF and AS (stars) are the Nova Floresta (1200 Ma), Fortuna Formation (1150 Ma) and Aguapeí sills (980 Ma, this work), respectively, from the Amazon Craton rotated according to the rotation parameters presented in (Table 4. Note that the APWP for Laurentia at 1.0–0.95 Ma is poorly defined and not outlined here. (b) After rotation, the AS and the Baltica poles plot in positions similar to what Weil et al. (1998, 2006) suggested for a ca. 1.10–0.95 Ma segment of the Laurentia APWP, shown here as a large arrowed loop. The black squares are Laurentia poles that range in age from 1113 to 960 Ma and refer to a selection of poles made by Weil et al. 1998 (their Table 1). (c) Tectonic reconstruction of the Amazon and Baltica cratons relative to Laurentia. Amazonia and Baltica have been rotated according to the rotation parameters of Table 4. Laurentia is shown in present coordinates. In the reconstruction at 980 Ma (Laurentia, Baltica and Amazonia in dark grey), the position of Baltica is based on a 966–946 Ma mean pole (Table 3), whereas that of Amazonia is from this work. The position of Amazonia at 1150 Ma is from D'Agrella-Filho et al. (2008) and at 1200 Ma is from Tohver et al. (2002). Rotation parameters are presented in Table 4.
The key to these reconstructions is the apparent polar wander for Laurentia between 1250 and 950 Ma. In Table 3, we have compiled a set of high-quality poles (Q ≥ 4) for Laurentia from 1230 to 1015 Ma. We use these poles to draw the APWP for Laurentia from 1230 to 1050 Ma (Fig. 11a), arranged into a counterclockwise loop (e.g. Meert & Torsvik 2003). For times after 1100 Ma, the APWP for Laurentia becomes more difficult to discern. The controversy arises from the interpretation of the ages of poles obtained on undated sedimentary rocks and from the post-collisional cooling of metamorphic rocks of the Grenville belt which define the path between 1050 and 800 Ma (see discussion in Weil et al. 2006). In addition, the younger poles (900–800 Ma) overlap well-defined Late Neoproterozoic-Cambrian poles, and their magnetization may represent a remagnetization (Warnock et al. 2000). The Haliburton pole (Buchan & Dunlop 1976), which defines the younger segment of the Mesoproterozoic Laurentia path, has recently been reassessed by Warnock et al. (2000), who suggest an age of 1015 ± 15 Myr for the Haliburton thermal remanent magnetization on the basis of thermochronological data. Recently, McCausland et al. (2007b) presented a new preliminary palaeomagnetic pole (Plat. = 18.3°N; Plon. = 146.4°E; A95 = 6.2) for the 974 ± 6 Ma (U-Pb zircon) Gilbert Bay dykes, which presents a positive baked contact test. If substantiated, these results constitute the best-defined primary magnetic remanence from Laurentia, preserved in the immediate aftermath of Grenville metamorphism.
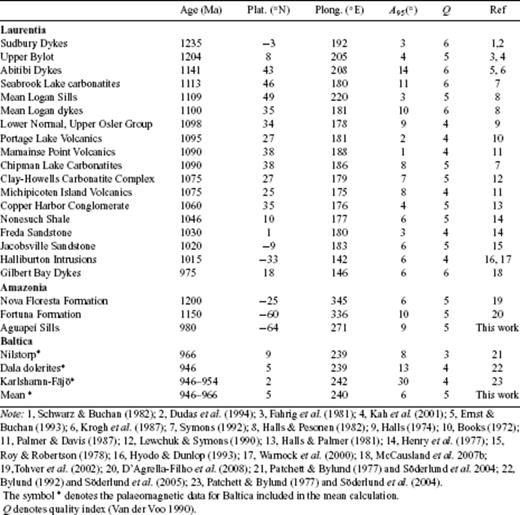
The palaeomagnetic reconstruction based on the three poles from Amazonia places Amazonia along the eastern border of Laurentia between 1200 and 980 Ma, using slightly different rotation poles (Table 4). The obtained palaeogeographic configurations is consistent with a continuous relative movement of these two cratons through a large-scale sinistral transcurrent movement (D'Agrella-Filho et al. 2008).
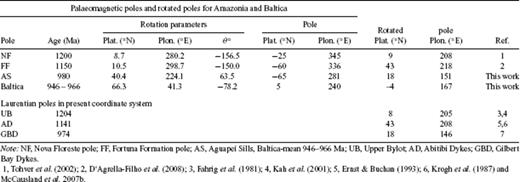
Palaeomagnetic poles and rotation parameters used in the reconstruction of Fig. 11.
In our 980 Ma reconstruction, Amazonia is rotated 63.5° into a position with its northern part attached to eastern Laurentia. This rotation is calculated using the rotation parameters of Table 4, which places the rotated Aguapeí sills pole over the Gilbert Bay pole. Note that the 980 Ma position for Amazonia relative Laurentia is similar to what has been proposed by other authors for the Rodinia reconstruction (e.g. Dalziel 1992, 1997; Torsvik et al. 1996; Weil et al. 1998; Dalziel et al. 2000). This position concludes the scenario of Amazonia moving in a present northeastward direction along the margin of Laurentia from 1200 to 980 Ma, meanwhile rotating counter-clockwise by approximately 180°. Most of this rotation is indicated to have occurred between 1150 and 980 Ma, with a rotation rate of ∼1° Myr−1. Post-collisional rotations of large plates have been reported elsewhere, for example, from the oblique collision of the Indian plate with Asia (Treloar & Coward 1991). The rotation rate for India was estimated at 0.4°–0.6° Myr−1, that is, the same order of magnitude as suggested here for Amazonia. We may speculate a similar scenario of oblique docking of Amazonia with Laurentia.
There is mismatch in the geological history between Rondonia and Eastern Bolivia, which suggests the Paraguá Craton and the Arequipa-Antofalla Basement should be regarded as allochtonous with respect to Amazonia (Loewy et al. 2004; Boger et al. 2005; Cordani et al. 2009). Various scenarios for the accretion of these terranes to Amazonia have been presented (e.g. Loewy et al. 2004; Boger et al. 2005). Ramos (2008) suggested that the Arequipa terrane was trapped during collision between Laurentia and Amazonia in the Mesoproterozoic, and Loewy et al. (2004) suggested that the Arequipa-Antofalla Basement collided with Amazonia as part of a larger continent.
If the Paragua Craton and the Arequipa Antofalla Basement separate from Amazonia, amalgamated to Laurentia at 1300–1400 Ma, as may be indicated by the Rondonia-San Ignacio collisional orogeny (e.g. Cordani & Teixeira 2007), which is expressed by several metamorphic belts, large shear zones and recurrent plutonism and magmatic arcs of similar ages in southern Laurentia (Van Schmus et al. 1996), Amazonia would have docked obliquely to Laurentia at ca. 1200 Ma. This initiated the transcurrent movement, which lasted until the collision with the Paragua craton (Tohver et al. 2004a, 2006a) and the Arequipa Antofalla Basement, at 1150–1100 Ma, manifested by the sinistral Ji-Parana shear zone (Tohver et al. 2005a) and the metamorphism in the Nova Brasilandia (NB) belt (Tohver et al. 2004a, 2005a). The oblique docking and this collision could be the reason for the rotation of Amazonia as indicated here.
It shall be pointed out that our high-quality pole for Amazonia at 980 Ma is not matched by similarly well-defined poles for Laurentia for the same age, which makes our tectonic reconstruction versus Laurentia tentative.
A palaeogeographic position of Baltica relative Laurentia at ca. 1.0 Ga can be calculated using a 966–946 Ma mean pole for Baltica (Table 3). After a rotation of –78.2° around an Euler pole (66.3°N, 41.3°E), the rotated pole falls between the 1020 Ma Jacobsville sandstone pole and the rotated Aguapei sills pole (Fig. 11). This rotation places Baltica north of the Amazon craton, and the data are compatible with an attachment of Baltica to the northeastern part of Laurentia (Greenland). A similar position for Baltica in Rodinia reconstructions has been suggested by many authors (e.g. Torsvik et al. 1996; Dalziel 1997). Such a relative position of Baltica and Laurentia is supported by a 950–930 Ma metamorphic event in central East Greenland (Watt & Thrane 2001), which is contemporaneous with high grade metamorphism in the Sveconorwegian orogenic belt in the southwestern part of Baltica (Johansson & Larionov 1999; Möller 1999). Note, however, that this part of Baltica is in our reconstruction located north of central East Greenland. Provenance studies from detrital zircon analyses made by Watt & Thrane (2001) suggest that the metamorphosed sediments in East Greenland have a Laurentian-Amazonian affinity. This supports the tectonic reconstruction presented here, with a close link between Amazonia, Laurentia and Baltica at ca. 980 Ma. The relative position of Amazonia, Laurentia and Baltica at this time is similar to the Rodinia configuration proposed by Li et al. (2008); however, the orientation of Amazonia is significantly different.
There is little evidence for a Grenville-age collision in northern Amazonia and southern-southwestern Baltica. Keppie & Ortega-Gutierrez (1999) suggest that two Central American blocks, the Oaxaquia and Chortis, originated as arcs in a Grenvillian ocean between Laurentia, Baltica and Amazonia. The high-grade collisional metamorphism in these blocks is proposed to be the result of the collisions with Laurentia, Baltica and Amazonia at ca. 1000 Ma, and in Rodinia reconstructions (Pisarevsky et al. 2003; Li et al. 2008), the Oaxaquia block is placed along the northern margin of Amazonia, within the zone of collision with Baltica. This position of the Oaxaquia block is consistent with palaeomagnetic data (Ballard et al. 1989), and Pisarevsky et al. (2003) presented a scenario where the Oaxaquia and the Chortis blocks may represent a continental arc formed on the present northern margin of Amazonia. Evidence for a Late Neoproterozoic link between Laurentia, Baltica and Amazonia (Pisarevsky et al. 2008 and references therein) suggests that the Rodinia configuration of these continents (Li et al. 2008) lasted until 610–550 Ma. This is indicated by coeval rift related mafic magmatism along the eastern margin of Laurentia and the western and southwestern margin of Baltica and mafic magmatism of this age (546 Ma) in Oaxaquia (Keppie et al. 2006). The Oaxaquia block is, in the reconstruction by Pisarevsky et al. (2008), located close to a volcanic province of similar age in western Ukraine (e.g. Elming et al. 2007).
The West African Craton is often proposed to be associated with the northeastern margin of Amazonia during the Late Precambrian (e.g. Trompette 1997; Cordani et al. 2003). The Gondwanaland Amazonia-West African fit has also been used in the Rodinia reconstructions (e.g Li et al. 2008). However, there is no reliable palaeomagnetic data available to verify that. A Gondwanaland fit with West Africa is not consistent with our reconstruction at 980 Ma, with Baltica located close to the present northeastern part of Amazonia.
Conclusion
A Late Mesoproterozoic palaeomagnetic pole for the Amazon craton is calculated from primary remanent magnetizations of basic sills in the southwestern part of the craton. The primary origin is demonstrated by a dual polarity in these unmetamorphosed rocks, and AMS data suggest that the sills and dykes are in their original position. The 40Ar/39Ar analyses revealed a well-defined plateau age at 981 ± 2 Myr for one site of the sill complex and indicate that some of the dykes are older than the sills.
On the basis of the pole to pole reconstruction presented here and geological data suggesting sinistral strike-slip shear in southwestern Amazonia in the time interval 1200–1100 Ma, we propose a scenario where Amazonia collided with Laurentia at ca. 1200 Ma and moved northeastwards along the present southeast coast of Laurentia and, meanwhile, rotated counter-clockwise by approximately 180°. At ca. 980 Ma, the palaeomagnetic data is consistent with northern-northwestern part of Amazonia attached to eastern Laurentia (Greenland), and the northeastern margin of Amazonia was facing the southern margin of Baltica, with the Oaxaquia block possibly in between. In this geometry for Rodinia, representing the end of Grenvillian orogenesis, the position of Amazonia is similar to the position in other models of Rodinia; however, the orientation is significantly different.
Since the high-quality pole for Amazonia at 980 Ma is not matched by similarly well-defined poles for Laurentia for the same age, our tectonic reconstruction is tentative. New palaeomagnetic data from well-dated 1000–950 Ma rocks from Laurentia are needed to further test the scenario presented here.
Acknowledgments
This work was financially supported by grants from FAPESP (grants 03/12802–2 and 07/59531), CNPq (grant 55.4458/05–5) and the Swedish Research Council (grant 2002–4886). The authors are greatful to Phil McCausland and an anonymous reviewer for their comments, which greatly improved the quality of this manuscript.
References