-
PDF
- Split View
-
Views
-
Cite
Cite
Caroline A Spike, Tatsuya Tsukamoto, David Greenstein, Ubiquitin ligases and a processive proteasome facilitate protein clearance during the oocyte-to-embryo transition in Caenorhabditis elegans, Genetics, Volume 221, Issue 1, May 2022, iyac051, https://doi.org/10.1093/genetics/iyac051
- Share Icon Share
Abstract
The ubiquitin-mediated degradation of oocyte translational regulatory proteins is a conserved feature of the oocyte-to-embryo transition. In the nematode Caenorhabditis elegans, multiple translational regulatory proteins, including the TRIM-NHL RNA-binding protein LIN-41/Trim71 and the Pumilio-family RNA-binding proteins PUF-3 and PUF-11, are degraded during the oocyte-to-embryo transition. Degradation of each protein requires activation of the M-phase cyclin-dependent kinase CDK-1, is largely complete by the end of the first meiotic division and does not require the anaphase-promoting complex. However, only LIN-41 degradation requires the F-box protein SEL-10/FBW7/Cdc4p, the substrate recognition subunit of an SCF-type E3 ubiquitin ligase. This finding suggests that PUF-3 and PUF-11, which localize to LIN-41-containing ribonucleoprotein particles, are independently degraded through the action of other factors and that the oocyte ribonucleoprotein particles are disassembled in a concerted fashion during the oocyte-to-embryo transition. We develop and test the hypothesis that PUF-3 and PUF-11 are targeted for degradation by the proteasome-associated HECT-type ubiquitin ligase ETC-1/UBE3C/Hul5, which is broadly expressed in C. elegans. We find that several GFP-tagged fusion proteins that are degraded during the oocyte-to-embryo transition, including fusions with PUF-3, PUF-11, LIN-41, IFY-1/Securin, and CYB-1/Cyclin B, are incompletely degraded when ETC-1 function is compromised. However, it is the fused GFP moiety that appears to be the critical determinant of this proteolysis defect. These findings are consistent with a conserved role for ETC-1 in promoting proteasome processivity and suggest that proteasomal processivity is an important element of the oocyte-to-embryo transition during which many key oocyte regulatory proteins are rapidly targeted for degradation.
Introduction
The oocyte-to-embryo transition (OET) initiates when oocytes exit meiotic prophase and enter the first meiotic metaphase, a cell cycle and developmental event also known as meiotic resumption or meiotic maturation. The OET is complete when zygotic gene transcription begins after fertilization in the early embryo. In many mammals and in the nematode Caenorhabditis elegans, zygotic gene transcription begins early at the 2- or ∼4-cell stage, respectively (Clegg and Pikó 1982; Latham et al. 1991; Edgar et al. 1994; Seydoux and Fire 1994; Hamatani et al. 2004). In some animals, such as Drosophila and Xenopus, zygotic gene activation predominantly begins later—at approximately the eighth nuclear division in Drosophila (Pritchard and Schubiger 1996; reviewed by Harrison and Eisen 2015) and at the midblastula transition in Xenopus (Newport and Kirschner 1982a, 1982b; Bachvarova et al. 1966; reviewed by Blitz and Cho 2021). Because the late-stage oocytes of most animals are transcriptionally quiescent, the regulation of protein translation by RNA-binding proteins represents a dominant mode of developmental control during oocyte meiotic maturation and the OET (reviewed by Kotani et al. 2017; Huelgas-Morales and Greenstein 2018; Teixeira and Lehmann 2019). In fact, genomic analyses suggest that mammalian oocytes contain more than 200 RNA-binding proteins (Luong and Conti 2019). The presumption is that many of these RNA-binding proteins regulate protein translation in oocytes and preserve the dowry of maternal mRNA contributed to the early embryo.
In C. elegans oocytes, the TRIM-NHL RNA-binding protein LIN-41 is a key component of a large translational regulatory RNP complex that associates with more than 1,000 mRNAs (Spike et al. 2014b; Tsukamoto et al. 2017). Many of these mRNAs encode protein components of LIN-41-containing RNPs and function as translational regulators during oogenesis or early embryonic development. LIN-41 also mediates the 3′-untranslated region (UTR)-mediated translational repression of some of the transcripts that associate with LIN-41 in oocytes and has an essential function to promote the extended meiotic prophase of oocytes (Spike et al. 2014a, 2014b; Tsukamoto et al. 2017). lin-41 null mutants are sterile because they produce pachytene-stage oocytes that exit meiotic prophase prematurely; these oocytes inappropriately disassemble the synaptonemal complex, cellularize, activate the CDK-1/cyclin B cyclin-dependent kinase, and enter M phase (Spike et al. 2014a). Hypomorphic lin-41 mutant alleles reduce oocyte quality and cause nondisjunction during female meiosis (Spike et al. 2014a). LIN-41 prevents the premature activation of the CDK-1/cyclin B during early oogenesis, in part, through the 3′-UTR-mediated translational repression of the CDC-25.3 phosphatase, a CDK-1 activator (Spike et al. 2014a, 2014b). Premature CDK-1 activation in lin-41 mutant oocytes drives precocious M-phase entry and causes oocytes to abnormally transcribe and express genes that are normally expressed zygotically after the OET (Allen et al. 2014; Spike et al. 2014a; Tocchini et al. 2014; Matsuura et al. 2016). Thus, a major function of LIN-41 appears to be to preserve mRNAs that are maternally inherited and translationally activated late in oogenesis or during early embryonic development. Many LIN-41-associated transcripts are translated only after LIN-41 is inactivated and degraded via ubiquitin-mediated degradation upon the onset of meiotic maturation (Spike et al. 2018). For example, LIN-41 associates with and represses the translation of the ORC-1 subunit of the origin recognition complex during meiotic prophase, and its degradation permits ORC-1 expression for DNA replication in the embryo (Tsukamoto et al. 2017).
LIN-41 is highly conserved and its mammalian ortholog, LIN-41/TRIM71, is required for embryonic viability and neural tube closure in mice (Maller Schulman et al. 2008; Chen et al. 2012; Cuevas et al. 2015; Mitschka et al. 2015). This defect appears to be related to a requirement for LIN-41/TRIM71 for maintaining pluripotency in neural progenitor cells in the embryo. LIN-41/TRIM71 has also been found to promote the pluripotency of embryonic stem cells in the mouse (Chang et al. 2012; Worringer et al. 2014; Mitschka et al. 2015; Liu et al. 2021). The defects in pluripotency, which underlie the neural tube defects observed in LIN-41/TRIM71 deficient mice, may be highly relevant to the finding of de novo heterozygous LIN-41/TRIM71 missense mutations in congenital hydrocephalus cases in humans (Furey et al. 2018; Jin et al. 2020). Germ cell-specific depletion of LIN-41/TRIM71 in mice causes germ cell loss and sterility in both sexes (Torres-Fernández et al. 2021a). Interestingly, male mice with LIN-41/TRIM71-deficient germ cells exhibit a Sertoli cell-only phenotype, which resembles the infertility phenotype of human males with heterozygous LIN-41/TRIM71 mutations (Torres-Fernández et al. 2021a). The essential role of LIN-41/TRIM71 in female germ cells remains to be more fully explored. Thus, in mammals, LIN-41/TRIM71 mutations produce distinct phenotypes in germ cells and somatic cells, which highlights the flexibility of LIN-41/TRIM71 proteins for deployment in developmental cell fate decisions. Indeed, LIN-41 was first discovered as a target of the let-7 microRNA in the genetic pathway controlling the developmental timing of somatic cells in C. elegans (Reinhart et al. 2000; Slack et al. 2000). The regulation of LIN-41/TRIM71 orthologs by let-7 microRNAs appears to be highly conserved in animals (Kloosterman et al. 2004; Kanamoto et al. 2006; Lin et al. 2007; O’Farrell et al. 2008; Rybak et al. 2009; Worringer et al. 2014). This mode of regulation is not observed in the C. elegans germline, as the let-7 microRNA appears to be only expressed in somatic cells (Lau et al. 2001). A new twist is that recent studies suggest that mammalian LIN-41/TRIM71 can inhibit the accumulation of let-7 microRNAs (Liu et al. 2021; Torres-Fernández et al. 2021b), suggesting its involvement as a component of a bistable developmental switch. Although it is not known whether LIN-41 can inhibit the let-7 pathway in C. elegans, it is clear that LIN-41 is a component of a bistable switch regulating oocyte meiotic maturation in this organism—LIN-41 inhibits CDK-1 activation, and in turn, active CDK-1 promotes the ubiquitin-mediated degradation of LIN-41 upon the onset of meiotic maturation (Spike et al. 2018).
The rapid elimination of LIN-41 upon the onset of meiotic maturation is emblematic of the highly choreographed and exquisitely timed patterns of degradation of RNA-binding proteins during late oogenesis and the OET in many organisms (Nishi and Lin 2005; Stitzel et al. 2006; Shirayama et al. 2006; Sha et al. 2017; Kisielnicka et al. 2018; Cao et al. 2020, 2022; Zavortink et al. 2020; reviewed by Robertson and Lin 2015). Previous results suggested that an SCF-type E3 ubiquitin ligase containing the FBW7/Cdc4 ortholog SEL-10 promotes the degradation of LIN-41 using a bi-partite degron located near the N-terminus of LIN-41 (Spike et al. 2018). The ubiquitin proteasome pathway utilizes an E1-E2-E3 enzymatic relay to transfer ubiquitin to mark proteins for degradation by the 26S proteasome. E3 ubiquitin ligases, comprising multisubunit enzymes or single chain proteins, function as substrate specificity factors to conjugate multiple ubiquitin moieties to target proteins. SEL-10 is a highly conserved and well-studied substrate-recognition subunit (Hubbard et al. 1997; de la Cova and Greenwald 2012). After substrate proteins are recognized and ubiquitinated, they are received by the 19S regulatory particles of the proteasome, which bind the ubiquitin chains, unfold the substrate, and processively translocate it into the 20S particle for degradation (reviewed by Finley 2009; Collins and Goldberg 2017).
In this work, we strengthen our understanding of LIN-41 degradation and begin to investigate how additional RNA-binding proteins in LIN-41 RNPs are degraded upon the onset of meiotic maturation. First, we show that SEL-10 is expressed in oocytes and use photoconversion of DENDRA::LIN-41 in maturing oocytes to demonstrate unequivocally that SEL-10 is required to degrade LIN-41 during the OET. Next, we identify the Pumilio/FBF-family RNA-binding proteins PUF-3 and PUF-11 as proteins that are degraded soon after meiotic maturation. PUF-3 and PUF-11 are components of LIN-41-containing RNPs in oocytes (Tsukamoto et al. 2017) that are 89% identical at the amino acid level and play important roles in germline stem cell and embryonic development (Hubstenberger et al. 2012, Haupt et al. 2020). Although both PUF-3 and PUF-11 are degraded soon after meiotic maturation in a CDK-1-dependent fashion, the degradation of both proteins occurs independently of SEL-10. Collectively, these results indicate that PUF-3 and PUF-11 are not degraded as a consequence of LIN-41 degradation and that LIN-41 RNPs are dismantled in a concerted fashion during the OET. Finally, we find that several different GFP-tagged fusion proteins that are degraded during the OET, including fusions with PUF-3, PUF-11 and LIN-41, are incompletely degraded when the function of the conserved ETC-1/UBE3C/Hul5 HECT-domain ubiquitin ligase is reduced, indicating that ETC-1 is important for proteasome processivity. We provide evidence that the GFP tag causes this proteolysis defect, re-evaluate key aspects of a previous report that assigned a different role to ETC-1 (Wang et al. 2013), and propose that proteasomal processivity is critical for the rapid and efficient targeting of a plethora of oocyte regulatory proteins for degradation during the OET.
Materials and methods
Strains and genetic analysis
The genotypes of strains used in this study are reported in Supplementary Table 1. All analyses were conducted at 20°C, unless specified otherwise. mat-1(ax161ts) animals are susceptible to even brief observations and manipulations at room temperature. Thus, strains containing the mat-1(ax161ts) mutation were handled as little as possible (<15 min at room temperature) and prechilled (15°C) media was used to establish cultures. The following mutations were used: LGI—rnp-8(tn1860[rnp-8::gfp::tev::3xflag]), mat-1(ax161ts), lin-41(tn1541[gfp::tev::s::lin-41]), lin-41(tn1892[mscarlet::tev::3xflag: lin-41]), lin-41(tn1894[dendra::tev::3xflag::lin-41]), lin-41(tn2054[gfp::tev::3xflag::lin-41]), lin-41(tn2055[gfp::tev::3xflag::lin-41]), and fog-3(q443). lin-41(tn2054) and lin-41(tn2055) were generated independently but are identical at the DNA sequence level. LGII—dpy-10(tn2076), etc-1(gk5182), etc-1(tn1919[gfp::tev::3xflag::etc-1]), etc-1(tn1920[gfp::tev::3xflag::etc-1]), etc-1(tn2077), and unc-4(e120). LGIII—plk-1(or683ts), emb-30(tn377ts), and cul-2(or209ts). LGIV—puf-11(tn1824[puf-11::gfp::tev::3xflag]), puf-11(q971), cyb-1(tn1806[cyb-1::gfp::tev::3xflag]), unc-22(e66), puf-3(tn1820[puf-3::gfp::tev::3xflag]), and puf-3(q966). LGV—lon-3(e2175), sel-10(ar41), sel-10(ok1632), sel-10(tn1816[sel-10::gfp::tev::3xflag]), sel-10(tn1817[sel-10::gfp::tev::3xflag]), sel-10(tn1875[gfp::tev::3xflag::sel-10]) and fog-2(oz40). sel-10(tn1816) and sel-10(tn1817) were generated independently but are identical at the DNA sequence level. The following balancers were used: tmC18[dpy-5(tmIs1236)] I (Dejima et al. 2018), mnC1[dpy-10(e128) unc-52(e444) umnIs32] II, nT1[qIs51] (IV; V). The following transgene insertions were used: itIs37[pie-1p::mcherry::histoneH2B::pie-1 3′UTR, unc-119(+)] IV (McNally et al. 2006) and ddIs128[ify-1::2xty1::egfp::3xflag(92C12) + unc-119(+)] (Sarov et al. 2012).
mScarlet and Dendra repair templates
The repair templates used to create lin-41(tn1892[mscarlet::tev::3xflag::lin-41]) and lin-41(tn1894[dendra::tev::3xflag::lin-41]) were generated by Gibson assembly using the NEBuilder HiFi DNA Assembly master mix (New England Biolabs, Ipswich, MA) and 5 different PCR products: (1) a lin-41 5′ homology arm (∼0.6 kb), (2) a codon-optimized mScarlet-I or a Dendra coding sequence with introns (∼0.9 kb), (3) self-excising cassette (SEC)-containing sequences (∼5.7 kb), (4) a lin-41 3′ homology arm (∼0.8 kb), and (5) vector backbone sequences (∼2.6 kb). lin-41 homology arms (1 and 4) were amplified from genomic DNA prepared from the wild type. SEC and vector sequences (3 and 5) were amplified from ClaI and SpeI-digested pDD268 plasmid DNA (Dickinson et al. 2015). mScarlet-I and Dendra were amplified from the plasmids pMS050 (Addgene plasmid #91826), a gift from Bob Goldstein, and pEG545 (Griffin et al. 2011), a gift from Geraldine Seydoux. Primer sequences are presented in Supplementary File 1. All PCR products were initially column purified (Qiagen, Valencia, CA) and most of the plasmids recovered after assembly lacked the SEC fragment; only 10% and 5% of the mScarlet-I and Dendra repair constructs were complete, respectively. While making different lin-41 repair constructs using the same method and many of the same PCR fragments, we found that gel purifying the SEC PCR product increased the inclusion of the SEC fragment to 80% of the assembled plasmids.
Constructs created by Griffin et al. (2011) that contain DENDRA-expressing sequences optimized for use in C. elegans (Gallo et al. 2010) are annotated in Addgene as containing DENDRA2 (e.g. pEG545, Addgene plasmid #40116 and pEG345, Addgene plasmid #40077). DENDRA2 is a modified form of DENDRA that has an important single amino acid change near the C-terminus (A224V) as well as a 5-amino acid insertion near the N-terminus (Gurskaya et al. 2006; Chudakov et al. 2007). The relationship between DENDRA and DENDRA2 is most easily visualized using the fluorescent protein database www.fpbase.org (Lambert 2019). We sequenced the pEG545 plasmid and confirmed that it encodes DENDRA; the DENDRA-coding sequence lacks both the A224V amino acid change and the 5-amino acid insertion found in DENDRA2. Since we obtained pEG545 directly from the Seydoux lab, we also analyzed the Addgene-deposited sequences of pEG545 and pEG345. Although those sequences are incomplete, they confirm that each plasmid encodes DENDRA[A224]. As both plasmids have been used to generate many different fusion proteins labeled as containing DENDRA2 [e.g. Herrera et al. (2016) and Rosu and Cohen-Fix (2017)], this annotation error may be relatively common in the C. elegans literature.
Genome editing
Plasmids that express guide RNAs (gRNAs) under the control of the U6 promoter were generated as described (Arribere et al. 2014). Repair templates used to tag genes with gfp were also generated as described (Dickinson et al. 2015). Repair templates used to tag genes with mSCARLET-I and DENDRA were generated as described in the previous section. Genome editing was performed by injecting wild-type adult gonads with a DNA mix containing a repair template (10 ng/µl), one or more gRNA plasmids (25 ng/µl each), Cas9-expressing plasmid (pDD162, 50 ng/µl), and injection marker (pMyo2::Tdtomato, 4 ng/µl) and selecting for repairs and selection cassette excisions using standard methods (Dickinson et al. 2015). Null mutations in lin-41 are both Dumpy (Dpy) and sterile (Slack et al. 2000; Spike et al. 2014a). None of the animals homozygous for the mScarlet^SEC^lin-41, Dendra^SEC^lin-41, and GFP^SEC^lin-41 repairs were Dpy, but some were sterile, consistent with the interpretation that the insertion of each SEC at the 5′ end of the lin-41 gene created reduction of function rather than null alleles of lin-41. Each lin-41 repair was balanced with tmC18 and the SEC was removed from the lin-41 locus in balanced heterozygotes. Fluorescently tagged lin-41 alleles were homozygous fertile after SEC excision. All edited loci were validated by sequencing the repair junctions using PCR products as templates. At least 2 alleles derived from independently injected parents were isolated and assigned allele names. A 4,240-bp deletion in the etc-1 locus, which removes the entire open reading frame was constructed using the dpy-10 coconversion method (Arribere et al. 2014). The injection mix contained pJA58 (7.5 ng/μl), AF-ZF-827 (500 nM), etc-1 sgRNA2 (pCS629) (25 ng/μl), etc-1 sgRNA4 (pCS633) (25 ng/μl), the repair template etc-1_RT1 (500 nM), and pDD162 (50 ng/μl). Correct targeting was verified by conducting PCR with primer pairs eif-2Bepsilon_F2 and hgap-2_F2 followed by Sanger sequencing. The DG5379 dpy-10(tn2076) etc-1(tn2077) strain was isolated after screening the progeny of 267 F1 Roller animals. The etc-1(tn2077) null mutation was separated from the dpy-10(tn2076) mutation by recombination by seeking non-Dpy non-Rol non-Unc animals from dpy-10(tn2076) etc-1(tn2077)/unc-4(e120) heterozygotes.
Antibody staining
Dissected gonads stained with the rabbit anti-GLD-1 primary antibody (1:200 or 1:300 dilution; Jan et al. 1999) were fixed in 1% paraformaldehyde for 10 min, with a 5-min post fix step in ice-cold methanol as described previously (Rose et al. 1997; Spike et al. 2018). Primary antibodies were detected using Cy3-conjugated donkey anti-rabbit secondary antibodies (1:500 dilution; Jackson ImmunoResearch, West Grove, PA).
Microscopy and image analysis
Microscope images in Fig. 4 and Supplementary Fig. 2 were acquired on a Carl Zeiss motorized Axioplan 2 microscope with a 63× Plan-Apochromat (numerical aperture 1.4) objective lens using an AxioCam MRm camera and AxioVision software (Carl Zeiss, Thornwood, NY). Microscope images in Supplementary Figs. 4, 5i–l, 6, 8, and 9 were acquired on a Nikon Ni-E microscope with either a Plan Apo λ 60× (numerical aperture 1.4) objective or a Plan Fluor 40× Oil (numerical aperture 1.3) objective using NIS elements (Nikon Inc., Melville, NY). All the remaining microscope images were acquired on a Nikon Ti2 inverted confocal microscope with a Plan ApoIR 60× objective (numerical aperture 1.27), motorized stage and Galvano scanner using NIS elements (Nikon Inc., Melville, NY). When using the Nikon Ti2 microscope, the large image function of NIS elements was used to acquire images that show the entire germline. We quantified the average fluorescence intensity in selected areas of images using the measure function of ImageJ (Schneider et al. 2012). Each measurement was background corrected using the average fluorescence intensity of a nearby area outside the body of the worm. Multiple images were analyzed per genotype, as specified in the text.
Photoconverting GreenDENDRA::LIN-41 to RedDENDRA::LIN-41
Worms were mounted without anesthetic on 10% agarose pads in a 1:1 solution of M9 buffer and 0.1 µM polystyrene beads (Polysciences, Inc., Warrington, PA) essentially as described (Rosu and Cohen-Fix 2020). Photoactivation and imaging were performed using the Nikon Ti2 inverted confocal microscope equipped as described in the previous section. A region of interest encompassing the −1 oocyte was selected for photoactivation using the 405-nm laser at 10% power for 31.25 s (125 loops at 4 frames per second) and a large pinhole (173.69 µm) to promote DENDRA photoconversion throughout the oocyte. After photoactivation, animals were allowed to recover on NGM plates seeded with OP50-1 bacteria for either 60 or 100 min and then mounted a second time. Confocal settings optimized to image oocytes after photoconversion were used to collect images of the GreenDENDRA::LIN-41 and RedDENDRA::LIN-41 in oocytes and embryos at all stages of the experiment: before photoactivation, after photoactivation, and after recovery.
Although the conditions used for photoactivation were generally compatible with oocyte meiotic maturation and ovulation, our experimental manipulations sometimes interfered with these events. For example, 1 lin-41(tn1894) animal failed to move after recovery, suggesting that it might have been damaged while mounting on or recovering from the agarose pad. RedDENDRA::LIN-41 was still detectable in the −1 and −2 oocytes of the photoconverted arm after a 100-min recovery period, indicating that the converted oocytes in this damaged animal had not matured or ovulated. The other lin-41(tn1894) animals (n = 5) survived the experimental manipulations and exhibited exclusively green oocytes in the −1 and −2 positions in each photoconverted arm (n = 6 arms, the −1 oocytes of both arms were converted in 1 animal), confirming that oocytes had matured and been ovulated into the uterus during the recovery period [60 min (n = 2) or 100 min (n = 4)]. All lin-41(tn1894); sel-10(ok1632) animals survived our experimental manipulations (n = 4 animals), and most of the photoconverted arms had exclusively green oocytes in the −1 and −2 positions (n = 4/5 arms, the −1 oocytes of both arms were converted in 1 animal) and 1 or 2 red embryos in the uterus after the recovery period. The sole exception was in an animal that laid only two eggs during the 100-min recovery period and appeared to be sickly. This animal had a bright red 1-cell stage embryo in the +1 position and a fainter red oocyte in the −1 position. The −1 oocyte converted in this gonad arm must have experienced a substantial temporal delay prior to oocyte maturation and ovulation, as a shorter (60 min) recovery period was sufficient for 2 ovulation events in the healthier lin-41(tn1894); sel-10(ok1632) animals.
itIs37 puf-3(tn1820) and puf-11(tn1824) puf-3(tn1820) double mutants
Crosses with puf-11(tn1824) and puf-3(tn1820) established that the itIs37[pie-1p::mcherry::histoneH2B::pie-1 3′UTR, unc-119(+)] insertion on chromosome IV is tightly linked to puf-11 and more loosely linked to puf-3. 0 of 72 progeny from itIs37 +/+ puf-11(tn1824) parents carried a recombinant itIs37 puf-11(tn1824) chromosome, while 5 of 64 progeny from itIs37 +/+ puf-3(tn1820) parents carried a recombinant itIs37 puf-3(tn1820) chromosome. Together, these observations suggested that itIs37 is ∼7.8 map units to the left of puf-3, in good agreement with the relative genetic positions of puf-11 and puf-3 described in WormBase (http://www.wormbase.org, last accessed April 6, 2022, release WS278; Davis et al. 2022). We utilized unc-22(e66), which causes a homozygous Uncoordinated (Unc) phenotype and is located in-between puf-11 and puf-3, to construct the puf-11(tn1824) puf-3(tn1820) double mutant. Animals carrying itIs37 puf-3(tn1820) and puf-11(tn1824) unc-22(e66) recombinant chromosomes were mated with each other. Animals with 1 copy of each chromosome were selected and allowed to self-fertilize. In the next generation, non-Unc-22 animals were examined for itIs37-derived mCHERRY::HISTONE expression using fluorescence microscopy. A single itIs37-negative animal was identified among the 168 non-Unc animals examined, and this animal segregated approximately one-quarter Unc progeny in the next generation. Based on the mapping data, we anticipated that this rare animal carried either a puf-11(tn1824) puf-3(tn1820) or a puf-11(+) puf-3(tn1820) recombinant chromosome in trans to the parental puf-11(tn1824) unc-22(e66) chromosome. Animals homozygous for the recombinant chromosome were analyzed using primers that produce allele-specific PCR products (Supplementary File 1) and found to carry both puf-11(tn1824) and puf-3(tn1820).
RNA interference
Gene-specific RNA interference (RNAi) was performed by feeding C. elegans with double-stranded RNA (dsRNA)-expressing E. coli (Timmons and Fire 1998) at 22°C using the RNAi culture media described by Govindan et al. (2006). RNAi clones were obtained from Source BioScience (Nottingham, UK), and the identities of important RNAi clones were verified by DNA sequencing (see Supplementary File 1 for details). Exposure to dsRNA-expressing E. coli was initiated during the fourth larval stage and animals were examined and imaged after 2 days. Under these RNAi conditions and at the time-point analyzed, all cdk-1(RNAi) animals produced arrested 1-cell embryos and therefore had a strong cdk-1(RNAi) phenotype (see Boxem et al. 1999). Using the same RNAi conditions, the gonad arms of wee-1.3(RNAi) animals exhibited a range of oocyte phenotypes. Gonads with a mild wee-1.3(RNAi) phenotype have relatively large and normal-appearing oocytes that occupy approximately normal positions with respect to each other and to the spermatheca. Gonads with strong wee-1.3(RNAi) phenotype have small, sometimes indistinct oocytes or a mass of oocyte-like material near the spermatheca. Burrows et al. (2006) also noted that prolonged RNAi results in small oocytes, consistent with the idea that this phenotype is caused by a strong response to wee-1.3(RNAi). GFPS::LIN-41 is prematurely degraded in animals with both weak and strong wee-1.3(RNAi) phenotypes (Spike et al. 2014a, 2018). In the screen for E3 ubiquitin ligases that degrade PUF-3::GFP, we did not exclusively use RNAi to test the SCF subunit CUL-2. Since our cul-2(RNAi) clone does not trigger a good RNAi response (Spike et al. 2018), we also examined cul-2(or209ts); puf-3(tn1820[puf-3::gfp::tev::3xflag]) adults 1 day after they were upshifted to 25°C as L4-stage larvae. All other ubiquitin ligase subunits were screened using RNAi alone.
Western blots
Each lane was loaded with a protein lysate made from adult worms picked into 10 µl of phosphate-buffered saline containing proteinase inhibitors (2× complete EDTA-free; Millipore-Sigma, St. Louis, MO). Whole-worm lysates were frozen at –80°C and prepared for electrophoresis by adding LDS sample buffer supplemented with a reducing agent (Invitrogen, Carlsbad, CA). Immediately after adding the sample buffer, the samples were heated at 70°C and vortexed multiple times prior to loading on a gel. Lysates were made from 40 Day 1 adult worms (24 h past the L4 stage at 20°C) with a few exceptions. Some lysates expressing CYB-1::GFP were made from 50 instead of 40 worms (hermaphrodites in Fig. 7a and Supplementary Fig. 7b; males in Fig. 7b and Supplementary Fig. 7d). In addition, the mated females used for lysates were not developmentally staged (Supplementary Fig. 7c). Instead, gravid females were selected from mixed-stage plates. Proteins were separated using 3–8% Tris-Acetate gels (Invitrogen, Carlsbad, CA) and visualized after western blotting. Blots were blocked with 5% nonfat dried milk. The primary antibodies used were mouse anti-FLAG M2 (Millipore-Sigma) at a 1:2,000–1:4,000 dilution and rabbit anti-GFP NB600-308 (Novus Biologicals, Littleton, CO) at a 1:4,000 dilution. The secondary antibodies used were peroxidase-conjugated goat anti-mouse (Thermo Scientific, Waltham, MA) at a 1:30,000 dilution or peroxidase-conjugated donkey anti-rabbit (Thermo Scientific) at a 1:5,000 dilution. Detection was performed using SuperSignal West Femto Maximum Sensitivity Substrate and CL-XPosure film (Thermo Scientific).
eGFP, mSCARLET-I, and DENDRA fusion protein tag sequences
The predicted amino sequence and molecular mass of each protein tag is presented in Supplementary File 2 for reference. All GFP tags express the GFP[S65C] enhanced GFP variant commonly used in C. elegans (Green et al. 2008). Most also contain the apparently neutral GFP[Q80R] change likely introduced into the original GFP cDNA sequence by PCR error (Tsien 1998).
Results
SEL-10 is present in the cytoplasm and nucleoplasm of oogenic germ cells
SEL-10/Cdc4/FBW7 is a highly conserved F-box protein; it is the substrate-recognition subunit of a Skp, Cullin, F-box (SCF)-containing E3 ubiquitin ligase complex that promotes the ubiquitination and degradation of specific substrates (reviewed in Deshaies and Ferrell 2001; Welcker and Clurman 2008). In C. elegans, SEL-10 functions in the oogenic germline and during the OET to promote the timely elimination of the cytoplasmic RNA-binding proteins GLD-1 and LIN-41 at different stages of oogenesis (Kisielnicka et al. 2018; Spike et al. 2018). Consistent with this idea, sel-10 mRNA is expressed throughout the oogenic germlines of adult hermaphrodites and the sel-10 3′-UTR is permissive for the translation of green fluorescent protein (GFP) in premeiotic and meiotic cells during oogenesis (Kisielnicka et al. 2018). However, the expression of SEL-10 has not been reported in oocytes or in any other region of the C. elegans germline, possibly because SEL-10 expression constructs were examined in the context of extrachromosomal arrays (Ding et al. 2007; Dorfman et al. 2009), which can silence the expression of germline genes in C. elegans (Kelly et al. 1997). To avoid this technical issue, we used genome editing to generate new alleles of sel-10 that have a GFP-tag fused to the SEL-10 protein at both the amino-terminus (N-terminus, GFPF::SEL-10 in Fig. 1) and carboxyl-terminus (C-terminus, SEL-10::GFPF in Fig. 1). In the figures, and where needed for textual clarity, we will indicate the presence and relative position of a 3 × FLAG or an S epitope tag in a fusion protein with an F or S subscript, respectively. The alleles that express GFP::SEL-10, SEL-10::GFP, and many of the other fusion proteins used in this work, are shown in Supplementary Fig. 1.
![SEL-10 is expressed in oocytes and embryos and promotes the degradation of mSCARLET::LIN-41 during the OET. mSCARLET::LIN-41 (a, b, d, f, i, and l), GFP::SEL-10 (c and h), and SEL-10::GFP (e and k) fluorescence in C. elegans germlines (solid outlines) and early embryos (dashed outlines). mSCARLET::LIN-41 is rapidly degraded after oogenesis in the wild type (a) and sel-10(tn1875[gfp::3xflag::sel-10]) (d) hermaphrodites but persists in early embryos in the sel-10(ok1632) deletion (b) and sel-10(tn1817[sel-10::gfp::3xflag]) (f) hermaphrodites. Embryos with an increased amount of mSCARLET::LIN-41 relative to sel-10(+) embryos are outlined in magenta (b and f). Magnified image insets of the selected regions (c–f) are shown for GFP::SEL-10 (g–i) and for SEL-10::GFP (j–l), respectively. Differential interference contrast microscopic images are shown (g and j) to highlight germ cell nucleoli (arrows). The circled nucleoli (g–l) lie in the middle focal plane of a germ cell nucleus, illustrating that functional GFP::SEL-10 is enriched in the nucleoplasm but largely excluded from nucleoli (g–i). Nonfunctional SEL-10::GFP is also excluded from nucleoli but is not enriched in the nucleoplasm relative to the cytoplasm at this stage of oocyte development (j–l). mSCARLET::LIN-41 is exclusively cytoplasmic and excluded from nuclei (i and l). Landmark structures such as the distal mitotic region of the germline (arrow in a), the proximal-most oocytes (arrowheads in a) and the spermatheca (sp), are indicated for reference. Scale bars: 100 µm (a–f) and 10 µm (g–l), respectively.](https://oup.silverchair-cdn.com/oup/backfile/Content_public/Journal/genetics/221/1/10.1093_genetics_iyac051/1/m_iyac051f1.jpeg?Expires=1750164566&Signature=BXwCBYin-eWOnXI2Ygy6ehEK6I4Lgs0yEOHCrJkRS62T-EGdg6YvHxzl4noQXsD-4R7VuobanrkZhq4We2DG48YCTiJ3T1GxF9SrtAopdTEfpmV99oZTfHefz0vgw9tVxVSmurjCLD9r3mbHtw7XGRLP0auZA~fXV3kCb26EShXWtUVg-Di3y4j3oM5eOhtZEdopHr7tfxKxINS4qYuvvar~~wVzRZ~MvQjokBGvip4CWd6QgYsPFW9NF3sxiaRY53bM3LwK8AJD0rCVaz3P5HlD9mXDme4FJzJ97GiPXqqoxQ26X6VXem2EhfWMtj7jlyHSQKzXz26DZnZA3awwRw__&Key-Pair-Id=APKAIE5G5CRDK6RD3PGA)
SEL-10 is expressed in oocytes and embryos and promotes the degradation of mSCARLET::LIN-41 during the OET. mSCARLET::LIN-41 (a, b, d, f, i, and l), GFP::SEL-10 (c and h), and SEL-10::GFP (e and k) fluorescence in C. elegans germlines (solid outlines) and early embryos (dashed outlines). mSCARLET::LIN-41 is rapidly degraded after oogenesis in the wild type (a) and sel-10(tn1875[gfp::3xflag::sel-10]) (d) hermaphrodites but persists in early embryos in the sel-10(ok1632) deletion (b) and sel-10(tn1817[sel-10::gfp::3xflag]) (f) hermaphrodites. Embryos with an increased amount of mSCARLET::LIN-41 relative to sel-10(+) embryos are outlined in magenta (b and f). Magnified image insets of the selected regions (c–f) are shown for GFP::SEL-10 (g–i) and for SEL-10::GFP (j–l), respectively. Differential interference contrast microscopic images are shown (g and j) to highlight germ cell nucleoli (arrows). The circled nucleoli (g–l) lie in the middle focal plane of a germ cell nucleus, illustrating that functional GFP::SEL-10 is enriched in the nucleoplasm but largely excluded from nucleoli (g–i). Nonfunctional SEL-10::GFP is also excluded from nucleoli but is not enriched in the nucleoplasm relative to the cytoplasm at this stage of oocyte development (j–l). mSCARLET::LIN-41 is exclusively cytoplasmic and excluded from nuclei (i and l). Landmark structures such as the distal mitotic region of the germline (arrow in a), the proximal-most oocytes (arrowheads in a) and the spermatheca (sp), are indicated for reference. Scale bars: 100 µm (a–f) and 10 µm (g–l), respectively.
The pattern of GFP expression from each sel-10 allele confirms that SEL-10 is expressed throughout the oogenic germline of adult hermaphrodites and in early embryos (Fig. 1c and e). GFP::SEL-10 and SEL-10::GFP are present in the nucleoplasm and cytoplasm of oocytes and distal germ cells, but show different patterns of localization, likely reflecting the fact that GFP::SEL-10 is functional, whereas SEL-10::GFP is not, as described in the subsequent section. GFP::SEL-10 is enriched in the nucleoplasm relative to the cytoplasm at all stages of germ cell development (Fig. 1c). GFP::SEL-10 localization throughout the distal region of the gonad appears to be “ring-like” because it is enriched in the nucleoplasm relative to the cytoplasm and largely excluded from nucleoli (Fig. 1c and g–l). SEL-10::GFP is also excluded from nucleoli at all stages of germ cell development (Fig. 1e and j–l). However, SEL-10::GFP is present at similar levels in the nucleoplasm and cytoplasm of distal germ cells and only becomes enriched in the nucleoplasm of growing oocytes (Fig. 1e). Using confocal images collected with identical settings, we measured 23% and 43% increases in background-corrected cytoplasmic fluorescence in the rachis and the −3 oocyte, respectively, in SEL-10::GFP animals relative to GFP::SEL-10 animals (P < 0.01, n = 7 images per genotype). However, nuclear fluorescence in the –3 oocyte of each strain was not significantly different (P > 0.1). We conclude that, at least in the oogenic germline, SEL-10::GFP appears to be more abundant than GFP::SEL-10 and more likely to reside in the cytoplasm.
GFP::SEL-10 is functional but SEL-10::GFP is not
The different patterns of GFP::SEL-10 and SEL-10::GFP localization might reflect the disruption or alteration of SEL-10 function by the inserted protein tags. We examined the accumulation of LIN-41 and GLD-1 in animals homozygous for each GFP-tagged allele of sel-10 to address this possibility. LIN-41 was monitored using lin-41(tn1892[mscarlet::3xflag::lin-41]), a new allele of lin-41 that produces a fusion protein with an mSCARLET-tag fused to the N-terminus of LIN-41 (Supplementary Fig. 1b; mSCF::LIN-41). Endogenous GLD-1 levels were analyzed by immunostaining. mSCARLET::LIN-41 is strongly expressed in wild-type and sel-10(ok1632) loss-of-function mutant oocytes (Fig. 1a and b). mSCARLET::LIN-41 is downregulated in wild-type embryos (Fig. 1a), but persists at elevated levels in the embryos produced by sel-10(ok1632) mutant animals (Fig. 1b). The downregulation of mSCARLET::LIN-41 protein expression during the OET is therefore SEL-10 dependent, as described for endogenous LIN-41 and a GFP-tagged LIN-41 fusion protein (Supplementary Fig. 1b; GFPS::LIN-41; Spike et al. 2018). mSCARLET::LIN-41 is downregulated in the embryos of GFP::SEL-10-expressing animals [sel-10(tn1875), Fig. 1d], but persists at elevated levels in the embryos of SEL-10::GFP-expressing animals [sel-10(tn1817), Fig. 1f]. Similar results were observed with GLD-1 (Supplementary Fig. 2). GLD-1 is downregulated as developing oocytes exit pachytene near the loop region (Supplementary Fig. 2a and c), and this process is impaired in sel-10(lf) mutants, resulting in elevated levels of GLD-1 in proximal oocytes (Kisielnicka et al. 2018; Spike et al. 2018). GLD-1 is downregulated in the oocytes of GFP::SEL-10-expressing animals [sel-10(tn1875), Supplementary Fig. 2b], but persists at elevated levels in the oocytes of SEL-10::GFP-expressing animals [sel-10(tn1816), Supplementary Fig. 2d]. We conclude that at least 2 of the normal functions of SEL-10 are compatible with the insertion of a GFP tag at the N-terminus of SEL-10 but are disrupted by the insertion of a GFP tag at the SEL-10 C-terminus. Furthermore, these observations suggest that the nucleoplasm-enriched localization pattern of GFP::SEL-10 shown in Fig. 1c likely represents the correct localization pattern of SEL-10 in the oogenic germline. Although known targets of SEL-10 in the C. elegans germline are predominantly cytoplasmic (e.g. LIN-41, GLD-1 and CPB-3; Kisielnicka et al. 2018; Spike et al. 2018), orthologs of SEL-10 are present in the nucleoplasm in S. cerevisiae (Cdc4p) (Choi et al. 1990) and in both the nucleoplasm and cytoplasm in humans (FBW7α and FBW7β isoforms, respectively) (Welcker et al. 2004).
SEL-10 is required to degrade LIN-41 during the OET
LIN-41 disappears rapidly upon the onset of meiotic maturation. We previously hypothesized that LIN-41 is phosphorylated at this stage and recognized by a SEL-10-containing E3 ubiquitin ligase that targets LIN-41 for proteolysis (Spike et al. 2018). To confirm that SEL-10 promotes the degradation of LIN-41 during the OET, we generated lin-41(tn1894[dendra::3xflag::lin-41]), a new allele of lin-41 that produces a protein with a DENDRA-tag fused to the LIN-41 N-terminus (Supplementary Fig. 1b; DNDF::LIN-41) and crossed it into a sel-10(ok1632) mutant background. Photoactivation irreversibly converts the fluorescence emitted by DENDRA from green to red (GreenDENDRA and RedDENDRA, respectively) (Gurskaya et al. 2006). The GreenDENDRA::LIN-41 present in the −1 oocytes of sel-10(ok1632) and sel-10(+) animals was targeted for conversion to RedDENDRA::LIN-41 using a 405-nm laser. Robust photoconversion was observed in the −1 oocyte, with a small amount of red fluorescence sometimes visible in the adjacent cytoplasm of the −2 oocyte (Fig. 2a, b, f, and g). After a suitable recovery period to permit oocyte maturation, ovulation, and some embryonic development, bright RedDENDRA::LIN-41 was evident in a single embryo in the uterus of sel-10(ok1632) animals (Fig. 2c–e; n = 5/5). In general, we noted that the next-youngest embryo (or −1 oocyte in 1 animal, see Materials and Methods) also contained RedDENDRA::LIN-41 (n = 4/5), but at a much lower level. We did not observe bright RedDENDRA::LIN-41 in any of the embryos in the uteri of sel-10(+) animals (n = 6), although we sometimes saw an embryo with a slight haze of red fluorescence that we hypothesized might be derived from the converted RedDENDRA::LIN-41 − 1 oocyte (Fig. 2h and i). Relative to these sel-10(+) embryos, we measured a 6- to 10-times higher level of background-corrected RedDENDRA::LIN-41 fluorescence in the brightest sel-10(ok1632) embryos (n = 4 of each genotype). Together, these results indicate that the fluorescently tagged RedDENDRA::LIN-41 observed in sel-10(ok1632) embryos originates in oocytes and confirm that LIN-41 is degraded during the OET in wild-type animals.
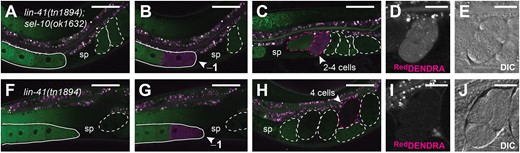
SEL-10 degrades oocyte-expressed DENDRA::LIN-41. Red and green channel fluorescence (a–c and f–h) in DENDRA::LIN-41-expressing sel-10(ok1632) (a–e) and sel-10(+) (f–j) hermaphrodites. The positions of oocytes (solid outlines), embryos (dashed outlines), and spermathecae (sp) are indicated for reference. Images were collected either before photoactivation (a and f), immediately after photoactivation of the −1 oocyte (arrowheads, b and g), or after a 100-min recovery period (c–e and h–j). Embryos with above-background levels of red channel fluorescence are outlined in magenta; the embryo that most likely developed from the photoactivated −1 oocyte is indicated with an arrowhead (c and h). Magnified red channel fluorescence (d and i) and DIC images (e and j) of this embryo are shown. Scale bars: 50 µm (a–c and f–h) and 20 µm (d, e, i, and j).
PUF-3 and PUF-11 are degraded during the OET
PUF-3 and PUF-11 are nearly identical Pumilio-family RNA-binding proteins that function redundantly with respect to normal embryonic development in C. elegans (Hubstenberger et al. 2012; Haupt et al. 2020). PUF-3 and PUF-11 proteins and mRNAs copurify with LIN-41-containing ribonucleoprotein (RNP) complexes (Tsukamoto et al. 2017). Furthermore, LIN-41 and PUF-3/11 were each identified as factors important for the 3′-UTR-mediated translational repression of the Rbfox-related RNA-binding protein SPN-4 in oocytes (Hubstenberger et al. 2012; Tsukamoto et al. 2017). These observations suggest that LIN-41, PUF-3, and PUF-11 function together in developing oocytes. We confirmed that PUF-3 and PUF-11 are expressed in oocytes, as described (Haupt et al. 2020), and also examined the expression patterns of PUF-3 and PUF-11 during the OET. We created new alleles of puf-3 and puf-11 that fuse a GFP-tag to the C-terminus of each PUF protein (Supplementary Fig. 1c; PUF-3::GFPF and PUF-11::GFPF) and found that PUF-3 and PUF-11 are eliminated during the OET in a manner that resembles LIN-41 (Fig. 3). PUF-3::GFP and PUF-11::GFP are abundant in oocytes, but the expression of each protein is strongly diminished soon after oocyte meiotic maturation and ovulation (Fig. 3a and d; Supplementary Fig. 3a and b). Consequently, PUF-3::GFP and PUF-11::GFP appear to be largely absent from embryos (Fig. 3a) or limited to a single young embryo (Fig. 3d and Supplementary Fig. 3a and b). A similar pattern is observed with GFP::LIN-41 and mSCARLET::LIN-41 (Spike et al. 2018; Fig. 1a). Moreover, PUF-3::GFP or PUF-11::GFP and mSCARLET::LIN-41 identify the same young embryos (if present, as in Fig. 3d and e; n ≥ 7 animals of each genotype imaged as shown). To determine when PUF-3 is eliminated from embryos, we examined PUF-3::GFP in oocytes and embryos that express an mCHERRY::HISTONE fusion protein. Relative to the adjacent −1 oocyte, PUF-3::GFP levels are substantially reduced in meiotic embryos that are in metaphase I (Fig. 3g–j) and most PUF-3::GFP appears to be eliminated by the end of the first meiotic division (Fig. 3k). This roughly corresponds to the time window during which LIN-41 is degraded; time-lapse imaging previously demonstrated that GFP::LIN-41 is dramatically reduced by the end of the first meiotic division (Spike et al. 2018).
![PUF-11::GFP and PUF-3::GFP are degraded during the OET. (a–f) PUF-11::GFP (a), PUF-3::GFP (d and g), and mSCARLET::LIN-41 (b and e) fluorescence in C. elegans germlines (solid outlines) and early embryos (dashed outlines). The arrow in (a) and (d) indicates the distal mitotic region of each germline. Merged images of (a) and (b) and (d) and (e) are shown in (c) and (f), respectively. (g–j) Images of itIs37[pie-1p::mcherry::histoneH2B::pie-1 3′UTR, unc-119(+)] puf-3(tn1820[puf-3::gfp::3xflag]) oocytes and embryos reveal that PUF-3::GFP (g) is strongly reduced in a meiosis I stage embryo (MI) relative to the −1 oocyte. Chromosomes are marked with mCHERRY: HISTONE (h), and the oocyte-derived chromosomes in this MI embryo appear to be aligning on the metaphase plate. PUF-3::GFP is at background levels in the adjacent postmeiotic 1-cell embryo (pn); this embryo has 2 polar bodies and maternal and paternal pronuclei at opposite ends of the embryo. Merged and DIC images of the same oocytes and embryos are shown in (i) and (j), respectively. The fluorescent images in this series (g–i) are 3D projections of a Z-stack. The DIC image (j) shows a single focal plane. Embryos with slightly higher levels of GFP and mSCARLET relative to older embryos are outlined in green and magenta, respectively (d, e, and g–j). Scale bars: 100 µm (a–f) and 50 µm (g–j). (k) PUF-3::GFP levels decrease after meiotic resumption. Mean GFP fluorescence was measured in images of itIs37[pie-1p::mcherry::histoneH2B::pie-1 3′UTR] puf-3(tn1820) oocytes and embryos and background corrected. Fluorescence intensity is expressed relative to the amount of GFP fluorescence in the oldest oocyte with an intact nucleus; this is the −2 oocyte if the −1 oocyte has initiated nuclear envelope breakdown (NEBD) or is starting to move into the spermatheca (early ovulation stage). Embryonic meiotic stage (e.g. metaphase I, telophase I, and metaphase II) was classified based on the arrangement and organization of maternal chromosomes.](https://oup.silverchair-cdn.com/oup/backfile/Content_public/Journal/genetics/221/1/10.1093_genetics_iyac051/1/m_iyac051f3.jpeg?Expires=1750164566&Signature=n5Z~jb~uaxAvi3T3Si3XlHZg9m-4RNynL9SuP9xStU2ouG1KBzg-5b8-Oni377W5D-GnBLPz0WGCt3Cm6XzD~-WyR4YaXb8wiwsKdyjjdqENKC2VHbn6ECaKpUUHtVOkXeUCS0bMnzyF5neve8ByUgBhBW5oJSKnWCRXID9snaYriXf6ke3AJ-SXqTgLTNx2ZDJ5vtGBf6MVRa8GeehZDXFVBCFiyJCE8r2XqvOTkjP9urojPv2rm9poxYkEH2zNYBlke3DCKBFS4mIFf43JvYaJ7-BempR7azF6wLoP0qVBBXbNXEx3SW4b~FEkv3XvjTXvywoaQwk6ZIotCWXZtA__&Key-Pair-Id=APKAIE5G5CRDK6RD3PGA)
PUF-11::GFP and PUF-3::GFP are degraded during the OET. (a–f) PUF-11::GFP (a), PUF-3::GFP (d and g), and mSCARLET::LIN-41 (b and e) fluorescence in C. elegans germlines (solid outlines) and early embryos (dashed outlines). The arrow in (a) and (d) indicates the distal mitotic region of each germline. Merged images of (a) and (b) and (d) and (e) are shown in (c) and (f), respectively. (g–j) Images of itIs37[pie-1p::mcherry::histoneH2B::pie-1 3′UTR, unc-119(+)] puf-3(tn1820[puf-3::gfp::3xflag]) oocytes and embryos reveal that PUF-3::GFP (g) is strongly reduced in a meiosis I stage embryo (MI) relative to the −1 oocyte. Chromosomes are marked with mCHERRY: HISTONE (h), and the oocyte-derived chromosomes in this MI embryo appear to be aligning on the metaphase plate. PUF-3::GFP is at background levels in the adjacent postmeiotic 1-cell embryo (pn); this embryo has 2 polar bodies and maternal and paternal pronuclei at opposite ends of the embryo. Merged and DIC images of the same oocytes and embryos are shown in (i) and (j), respectively. The fluorescent images in this series (g–i) are 3D projections of a Z-stack. The DIC image (j) shows a single focal plane. Embryos with slightly higher levels of GFP and mSCARLET relative to older embryos are outlined in green and magenta, respectively (d, e, and g–j). Scale bars: 100 µm (a–f) and 50 µm (g–j). (k) PUF-3::GFP levels decrease after meiotic resumption. Mean GFP fluorescence was measured in images of itIs37[pie-1p::mcherry::histoneH2B::pie-1 3′UTR] puf-3(tn1820) oocytes and embryos and background corrected. Fluorescence intensity is expressed relative to the amount of GFP fluorescence in the oldest oocyte with an intact nucleus; this is the −2 oocyte if the −1 oocyte has initiated nuclear envelope breakdown (NEBD) or is starting to move into the spermatheca (early ovulation stage). Embryonic meiotic stage (e.g. metaphase I, telophase I, and metaphase II) was classified based on the arrangement and organization of maternal chromosomes.
While LIN-41, PUF-3 and PUF-11 are strongly reduced soon after meiotic maturation and ovulation, each protein has a distinct pattern of expression in the adult germline (Fig. 3a–f). For example, the accumulation of PUF-3::GFP and PUF-11::GFP appears to peak at a later stage of oogenesis than mSCARLET::LIN-41 (Fig. 3c and f). In addition, PUF-11::GFP is distinctly more abundant than PUF-3::GFP in the distal germline (compare Fig. 3a and d; Supplementary Fig. 3d). Haupt et al. (2020) also observed higher levels of PUF-11 in the distal germline using alleles that express V5 epitope-tagged PUF-3 and PUF-11 proteins. Because puf-3 and puf-11 are redundant, to assess the function of the fusion proteins, we screened for and identified a recombinant that expresses both PUF-3::GFP and PUF-11::GFP using standard genetic methods (see Materials and Methods). As expected from the individual expression patterns, GFP expression was approximately 2-times higher in the oocytes of these animals [puf-11(tn1824) puf-3(tn1820) genotype] than in the oocytes of either parent [puf-11(tn1824) or puf-3(tn1820) alone], and the level of GFP expression in the distal germline was similar to the PUF-11::GFP-expressing strain (Supplementary Fig. 3a–e). puf-11(tn1824) puf-3(tn1820) animals are overtly wild-type; they are homozygous viable and fertile, with a brood size similar to the wild type (333 ± 40 larvae, n = 15 animals at 20°C). In addition, most of the embryos produced by puf-11(tn1824) puf-3(tn1820) animals hatch (∼1% embryonic lethality). Since animals that are doubly homozygous for deletion alleles of puf-11 and puf-3 [puf-11(q971) puf-3(q966) animals] exhibit maternal-effect embryonic lethality (Haupt et al. 2020), the GFP-tagged alleles of puf-11 and puf-3 must retain some wild-type function. As a more stringent test, we crossed homozygous puf-11(tn1824) puf-3(tn1820) males to balanced puf-11(q971) puf-3(q966)/nT1[qIs51] hermaphrodites to generate puf-11(tn1824) puf-3(tn1820)/puf-11(q971) puf-3(q966) animals. Animals with this genotype produced many embryos that were viable (n = 25 animals). We conclude that the GFP tag inserted at the C-terminus of PUF-3 and PUF-11 is largely compatible with the function of each protein.
PUF-3 and PUF-11 are degraded in response to active CDK-1
LIN-41 is degraded in response to meiotic resumption and the activation of the cyclin-dependent kinase CDK-1 (Spike et al. 2014a, 2018). Likewise, we find that using RNAi to strongly reduce CDK-1 activity causes PUF-3::GFP and PUF-11::GFP to persist in embryos (Fig. 4a and e; n > 65 for each PUF::GFP with 100% penetrance). Furthermore, a strong RNAi-mediated reduction in the activity of the WEE-1.3 kinase, a negative regulator of CDK-1 (Burrows et al. 2006), causes oocytes to downregulate PUF-3::GFP and PUF-11::GFP prematurely (Fig. 4b and f). The latter phenotype was partially penetrant; it was observed in 32/46 of the PUF-3::GFP and 25/44 of the PUF-11::GFP-expressing wee-1.3(RNAi) gonads and mainly seen in wee-1.3(RNAi) animals with highly abnormal oocytes. GFP::LIN-41 is also degraded prematurely after wee-1.3(RNAi), but this can be seen in gonads with relatively normal oocytes (Spike et al. 2014a), suggesting that LIN-41 might be more sensitive to CDK-1 activity. We tested this idea by treating animals that coexpress mSCARLET::LIN-41 and PUF-11::GFP with wee-1.3(RNAi) and selectively examining gonads with large, well-organized oocytes. In these animals, mSCARLET::LIN-41 was downregulated in younger (more distal) oocytes (Fig. 4i) and, as a consequence, prematurely eliminated from oocytes more frequently than PUF-11::GFP (in 11/12 instead of 6/12 gonads, respectively). Collectively, these results indicate that active CDK-1 promotes the elimination of PUF-3 and PUF-11 and suggest that LIN-41 might be more sensitive to the dysregulatory effects of wee-1.3(RNAi).
![The elimination of PUF-3::GFP and PUF-11::GFP requires active CDK-1 but does not require SEL-10. PUF-3::GFP (a–d) and PUF-11::GFP (e–h) fluorescence in C. elegans germlines (solid outlines) and early embryos (dashed outlines) in cdk-1(RNAi) (a and e), wee-1.3(RNAi) (b and f), lon-3(e2175) (c and g), and lon-3(e2175) sel-10(ar41) (d and h) hermaphrodites. For each GFP fusion protein, the images of the lon-3(e2175) and lon-3(e2175) sel-10(ar41) animals were collected using the same settings (e.g. c and d). All embryos appear GFP-positive in (a and e) and are outlined. The slight reduction of PUF-3/11::GFP levels in more proximally located (i.e. older) arrested embryos in the uterus (a and e) might reflect CDK-1-independent turnover or it could result from residual CDK-1 activity following the RNAi treatment. The position of the spermatheca (sp) is indicated for reference. Arrowheads indicate the most proximal oocyte in each wee-1.3(RNAi) gonad that is strongly GFP-positive. (i) This graph illustrates the relative position of the most proximal mSCARLET or GFP-positive oocyte in 12 different lin-41(tn1892[mScarlet::3xflag::lin-41]); puf-11(tn1824[puf-11::gfp::3xflag]); wee-1.3(RNAi) animals. wee-1.3(RNAi) animals with well-formed and organized oocytes were scored. Oocyte position was measured relative to other oocytes and the spermatheca (position 0). Each animal is represented by a different symbol (e.g. filled diamond or open circle). The selection of a filled or open symbol is arbitrary and made to depict the 12 animals scored with 6 symbol shapes. The vertical bar indicates the median. Scale bars: 50 µm (a–h).](https://oup.silverchair-cdn.com/oup/backfile/Content_public/Journal/genetics/221/1/10.1093_genetics_iyac051/1/m_iyac051f4.jpeg?Expires=1750164566&Signature=RfHa34DLQbbLizLGI1GsXaeqWCqXpwe~--T~D5AkRCZ74NjkqPgBLFEdMm7cJ8PqsUUCs0n~ZBQ0ZDf5dg6PGzAYxIqcf896rV2qHERkwXPE~RI5gS8ig1GnDd-t9Ri4XUCMVDfqcXZpVBl7~idqcHZ7pmPofUIS7K94us7mJJxwce3qj1oM3Yx9jFW2mLKcDUsfrEfp0LdY85awYxqA4k0qNaHrxKjx5noreDVEn21aHZsnFZZk0MQ7W5i1y4RFD-M~5pHYK20Mjc3SQH4Lu-e54SasXibVlIZFDo0h3-YObGaD7Rar1RGc3h3ejnQb99nDEQ32pWmqrMO8PdUCvg__&Key-Pair-Id=APKAIE5G5CRDK6RD3PGA)
The elimination of PUF-3::GFP and PUF-11::GFP requires active CDK-1 but does not require SEL-10. PUF-3::GFP (a–d) and PUF-11::GFP (e–h) fluorescence in C. elegans germlines (solid outlines) and early embryos (dashed outlines) in cdk-1(RNAi) (a and e), wee-1.3(RNAi) (b and f), lon-3(e2175) (c and g), and lon-3(e2175) sel-10(ar41) (d and h) hermaphrodites. For each GFP fusion protein, the images of the lon-3(e2175) and lon-3(e2175) sel-10(ar41) animals were collected using the same settings (e.g. c and d). All embryos appear GFP-positive in (a and e) and are outlined. The slight reduction of PUF-3/11::GFP levels in more proximally located (i.e. older) arrested embryos in the uterus (a and e) might reflect CDK-1-independent turnover or it could result from residual CDK-1 activity following the RNAi treatment. The position of the spermatheca (sp) is indicated for reference. Arrowheads indicate the most proximal oocyte in each wee-1.3(RNAi) gonad that is strongly GFP-positive. (i) This graph illustrates the relative position of the most proximal mSCARLET or GFP-positive oocyte in 12 different lin-41(tn1892[mScarlet::3xflag::lin-41]); puf-11(tn1824[puf-11::gfp::3xflag]); wee-1.3(RNAi) animals. wee-1.3(RNAi) animals with well-formed and organized oocytes were scored. Oocyte position was measured relative to other oocytes and the spermatheca (position 0). Each animal is represented by a different symbol (e.g. filled diamond or open circle). The selection of a filled or open symbol is arbitrary and made to depict the 12 animals scored with 6 symbol shapes. The vertical bar indicates the median. Scale bars: 50 µm (a–h).
SCFSEL-10 and APC ubiquitin ligases do not target PUF-3 or PUF-11 for degradation
PUF-3 and PUF-11 are components of LIN-41 RNPs and appear to be eliminated in response to the same signal and at a similar time as LIN-41. We therefore asked whether they are targeted for elimination by the same SCF E3 ubiquitin ligase. LIN-41 persists in early embryos after the RNAi-mediated knockdown of the highly similar Skp-related genes skr-1 and skr-2, the Cullin cul-1, the F-box substrate recognition subunit sel-10 and in the sel-10(lf) mutants sel-10(ok1632) and sel-10(ar41) (Spike et al. 2018). However PUF-3::GFP and PUF-11::GFP are eliminated normally from the embryos of lon-3(e2175) sel-10(ar41) mutant animals (Fig. 4c, d, g, and h) and after skr-1/2(RNAi) (Supplementary Fig. 4c and i, n > 40 animals for each PUF::GFP fusion protein) and cul-1(RNAi) (Supplementary Fig. 4b and h, n ≥ 20 animals for each PUF::GFP fusion protein). A distinct, SEL-10-independent mechanism must therefore promote the elimination of PUF-3 and PUF-11 from early embryos. Furthermore, this indicates that the degradation of PUF-3 and PUF-11 during the OET is not a consequence of the SEL-10-dependent degradation of LIN-41, consistent with our data suggesting that LIN-41 is more sensitive to wee-1.3(RNAi) and the idea that multiple mechanisms remodel and take apart oocyte RNPs during the OET.
An interesting feature of LIN-41 degradation is that it occurs even when the metaphase-to-anaphase transition of the first meiotic division is blocked by disrupting the function of the anaphase-promoting complex (APC) (Spike et al. 2014a). This feature distinguishes LIN-41 from proteins such as IFY-1/Securin (Kitagawa et al. 2002; Wang et al. 2013) and CYB-1/Cyclin B1 (Liu et al. 2004), which are degraded slightly later than LIN-41 in an APC-dependent fashion. Therefore, we tested whether the metaphase-to-anaphase transition regulates the elimination of PUF-3::GFP by impairing the function of 2 key members of the APC, mat-1/Apc3 and emb-30/Apc4 (Furuta et al. 2000; Shakes et al. 2003). mat-1(RNAi) caused fully penetrant 1-cell embryonic arrest, but did not delay or prevent the elimination of PUF-3::GFP from embryos (Supplementary Fig. 4d; n = 34 animals). Likewise, PUF-3::GFP disappears normally from the embryos of emb-30(tn377ts) mutants shifted to 25°C at the L4 stage (Supplementary Fig. 4f; n > 35); these embryos arrest in metaphase during the first meiotic division (Furuta et al. 2000). PUF-11::GFP degradation was also unaffected by mat-1(RNAi) (Supplementary Fig. 4j; n = 9). We conclude that, as for LIN-41, the elimination of either PUF-3 or PUF-11 from early embryos does not require the activity of the APC. However, unlike LIN-41, the elimination of PUF-3::GFP and PUF-11::GFP from embryos is sel-10 independent.
ETC-1/Hul5/UBE3C promotes efficient protein clearance during the OET
Since PUF-3/11 are degraded in a SEL-10-independent manner, and because regulated protein degradation often requires ubiquitination (reviewed by Dikic 2017), we tested whether a different E3 ubiquitin ligase might promote the elimination of PUF-3::GFP from embryos. Many potential ubiquitin ligases have been identified in C. elegans, including (1) multisubunit E3s (SCF-type, APC), (2) HECT-domain E3s, (3) U-box E3s, and (4) more than 100 RING-finger proteins that might function as monomeric E3s (Kipreos 2005). We performed a small-scale RNAi screen that targeted the first 3 classes of ubiquitin ligases and identified the HECT-domain ubiquitin ligase ETC-1/Hul5/UBE3C as a promising candidate. When animals expressing both mSCARLET::LIN-41 and PUF-3::GFP [lin-41(tn1892); puf-3(tn1820) genotype] were exposed to etc-1(RNAi), there was no discernable change in mSCARLET::LIN-41 degradation, yet elevated levels of GFP were observed in multiple young embryos (n = 24/24 animals). This suggested that etc-1 knock down specifically interferes with the elimination of oocyte-expressed PUF-3::GFP but not mSCARLET::LIN-41. We confirmed these RNAi-based observations with etc-1(gk5182), a predicted loss-of-function allele, which introduces a premature stop codon, generated by the C. elegans Reverse Genetics Core Facility at the University of British Columbia (The C. elegans Deletion Mutant Consortium 2012). etc-1(gk5182); puf-3(tn1820) and etc-1(gk5182); puf-11(tn1824) animals exhibit GFP fluorescence in multiple embryos compared to etc-1(+) controls (Fig. 5a–d), while expanded mSCARLET is not observed in lin-41(tn1892); etc-1(gk5182) animals (Fig. 5e and f). However, detailed analysis presented in subsequent sections suggests that ETC-1 unlikely functions as an E3 ubiquitin ligase that targets specific proteins for degradation during the OET but instead functions as a factor that increases the processivity of the proteasome.

etc-1(gk5182) perturbs the degradation of GFP-fusion proteins during the OET. PUF-3::GFP (a and b), PUF-11::GFP (c and d), mSCARLET::LIN-41 (e and f), CYB-1::GFP (g and h), IFY-1::GFP (i and j), and GFPF::LIN-41 (k and l) fluorescence in young etc-1(+) (a, c, e, g, i, and k) and etc-1(gk5182) (b, d, f, h, j, and l) embryos. In each image, the position of the spermatheca (sp) is indicated for reference. Young embryos in the uterus are indicated with dashed outlines that highlight individual embryos (a–h, k, and l) or a bracket that indicates a region containing multiple postmeiotic embryos (i and j). GFP-positive embryos are indicated in green (a–d and g–l) while mSCARLET-positive embryos are indicated in magenta (e and f). For each fusion protein, these embryos contain detectably more fluorescence than the etc-1(+) embryos indicated in white. CYB-1::GFP reaccumulates to relatively high levels in older etc-1(+) embryos (g, arrowhead; this embryo is no longer in the uterus) and therefore has a distinctly different expression pattern than the other OET-degraded fusion proteins. Scale bars: 50 µm.
ETC-1 was proposed to function as an E3 ubiquitin ligase that regulates the level of cytoplasmic IFY-1/Securin and CYB-1/cyclin B1 in postmeiosis I embryos in C. elegans (Wang et al. 2013). In that study, RNAi was used to reduce etc-1 function [etc-1(RNAi)] and CYB-1 degradation was monitored using a transgene that uses heterologous regulatory elements to drive the expression of a GFP::CYB-1 fusion protein in the C. elegans germline (ekIs2[pie-1p::gfp::cyb-1]). We used a new viable and fertile allele of cyb-1 that expresses a GFP-tagged CYB-1 fusion protein (Supplementary Fig. 1c; CYB-1::GFPF) to examine CYB-1::GFP expression in wild-type and in etc-1(gk5182) embryos (Fig. 5g and h). Since CYB-1 is essential for viability (van der Voet et al. 2009), the CYB-1::GFP fusion protein made by cyb-1(tn1806[cyb-1::gfp::3xflag]) homozygotes must be substantially functional in vivo. In otherwise wild-type animals, CYB-1::GFP is reduced in young mitotic embryos relative to meiotic embryos and oocytes (Fig. 5g). However, in etc-1(gk5182); cyb-1(tn1806) embryos GFP levels continue to remain high after meiosis (Fig. 5h). We also confirmed that etc-1(gk5182) affects the degradation of IFY-1::GFP (IFY-1::GFPF) using the fosmid-based transgene ddIs128[ify-1::gfp::3xflag] (Sarov et al. 2012). etc-1(gk5182) appears to have a relatively weak effect on IFY-1::GFP (Fig. 5i and j) compared to PUF-3::GFP, PUF-11::GFP, and CYB-1::GFP (Fig. 5a–d and g–h). The amount of GFP fluorescence in postmeiotic etc-1(gk5182); ddIs128 embryos is only slightly elevated relative to etc-1(+) control embryos (Fig. 5i and j). We quantified this modest increase by comparing the IFY-1::GFP fluorescence levels in +2 embryos from etc-1(gk5182) animals and etc-1(+) controls and found it to be increased 1.4-times in etc-1 mutants (P < 1 × 10−4, t-test; n = 12 for each genotype).
ETC-1 is broadly expressed and present in oocytes and early embryos
ETC-1 and its orthologs, Hul5 (yeast) and UBE3C (humans) have 2 well-defined structural domains: an IQ domain close to the N-terminus and a HECT domain at the extreme C-terminus (Fig. 6a; Wang et al. 2013; Marín 2018). The HECT domain of UBE3C and other HECT-domain E3 ubiquitin ligases has a well-defined function; it is essential for mediating the acceptance and transfer of ubiquitin from an E2-conjugating enzyme to a degradation substrate via a cysteine residue at its catalytic site (You and Pickart 2001; Singh and Sivaraman 2020; reviewed in Lorenz 2018). The IQ domain-containing N-terminus of UBE3C mediates an interaction with the proteasome that is also important for function, but this portion of UBE3C is less well-conserved than the HECT domain and its function is less well-defined (You and Pickart 2001; You et al. 2003; Wang et al. 2013). Given this structural and functional information, we decided to tag the extreme N-terminus with GFP to examine where ETC-1 is expressed and localized in C. elegans.
![ETC-1 is expressed in the C. elegans germline. a) etc-1 alleles and the exon–intron structure of etc-1, the downstream gene in operon CEOP2380. Exons are shown as open boxes; the region of exon 1 between ATG #1 and ATG #2 would encode 20 additional amino acids if translation is initiated at ATG #1, the start codon immediately adjacent to the annotated SL1/SL2 trans-splice site (attttag), instead of ATG #2. Other regions indicated are the 3′-UTR of the upstream gene eIF-2B and the regions that encode the conserved IQ and HECT domains of ETC-1. b) The N-terminus of ETC-1 aligned with the N-terminus of the human ortholog UBE3C; the amino acids predicted to be added to ETC-1 are shown. This is the N-terminal portion of a full-length protein alignment generated by EMBOSS Needle with default alignment parameters. ETC-1 and UBE3C were 26% identical or 46% similar overall with 16% of the alignment gapped. c and d) GFP::ETC-1 fluorescence in C. elegans germlines (solid outlines) and early embryos (dashed outlines). GFP is N-terminal to either the first [c, etc-1(tn1919[gfp::3xflag::etc-1 #1])] or second [d, etc-1(tn1920[gfp::3xflag::etc-1#2])] methionine of ETC-1. Scale bars: 100 µm. e) A western blot of GFP::ETC-1 (∼150 kDa, see main text) and PUF-3::GFP-expressing strains with an anti-FLAG antibody that recognizes both fusion proteins. GFP::FLAG fragments derived from PUF-3::GFP strongly accumulate in etc-1(gk5182) and etc-1(tn1920[gfp::3xflag::etc-1#2]) hermaphrodites.](https://oup.silverchair-cdn.com/oup/backfile/Content_public/Journal/genetics/221/1/10.1093_genetics_iyac051/1/m_iyac051f6.jpeg?Expires=1750164566&Signature=qiCppvgSp8M3~K4w3YLOYBy1E9AJmMvxyavjjhFrLe1Z0rK~USFdMsmtLUz4LXA9PQlAycF9Ofw7CLCIAcYNyFL9~BmNTdI6O1388NmK-H7smCDj0~PW0VpNlYZAzeLF02NRqZnyx5fYWYUaxuP3MFx3DRh~rZQcXslZN2KM~shGeWmB041U0HjKHhkySUNxQQ~8DHGjqz0gpAVKgRwp6BE9nJnZs43rq0EcwS-ZdW0FFPEOiGONMLM~RbUZHZb61ig2VLRfQDGj1Lf~0KrIjznhNl0YkOqsCwxiaRor8ayRKEAWBRnXy6qXxpP-FSGSTOayWp4n~oWtAHd6BQL88A__&Key-Pair-Id=APKAIE5G5CRDK6RD3PGA)
ETC-1 is expressed in the C. elegans germline. a) etc-1 alleles and the exon–intron structure of etc-1, the downstream gene in operon CEOP2380. Exons are shown as open boxes; the region of exon 1 between ATG #1 and ATG #2 would encode 20 additional amino acids if translation is initiated at ATG #1, the start codon immediately adjacent to the annotated SL1/SL2 trans-splice site (attttag), instead of ATG #2. Other regions indicated are the 3′-UTR of the upstream gene eIF-2B and the regions that encode the conserved IQ and HECT domains of ETC-1. b) The N-terminus of ETC-1 aligned with the N-terminus of the human ortholog UBE3C; the amino acids predicted to be added to ETC-1 are shown. This is the N-terminal portion of a full-length protein alignment generated by EMBOSS Needle with default alignment parameters. ETC-1 and UBE3C were 26% identical or 46% similar overall with 16% of the alignment gapped. c and d) GFP::ETC-1 fluorescence in C. elegans germlines (solid outlines) and early embryos (dashed outlines). GFP is N-terminal to either the first [c, etc-1(tn1919[gfp::3xflag::etc-1 #1])] or second [d, etc-1(tn1920[gfp::3xflag::etc-1#2])] methionine of ETC-1. Scale bars: 100 µm. e) A western blot of GFP::ETC-1 (∼150 kDa, see main text) and PUF-3::GFP-expressing strains with an anti-FLAG antibody that recognizes both fusion proteins. GFP::FLAG fragments derived from PUF-3::GFP strongly accumulate in etc-1(gk5182) and etc-1(tn1920[gfp::3xflag::etc-1#2]) hermaphrodites.
When we examined the sequences at the 5′ end of the etc-1 gene (Fig. 6a), it became apparent that ETC-1 translation might begin 60 bp upstream of the annotated start codon (ATG #2 in Fig. 6a) (http://www.wormbase.org, release WS278). etc-1 is the downstream gene in a 2-gene operon and contains an annotated and sequence-verified trans-splice acceptor site immediately adjacent to a possible start codon (ATG #1 in Fig. 6a). In C. elegans, trans-splicing is used to physically separate the cotranscribed messenger RNAs of an operon (Spieth et al. 1993). We predicted that ETC-1 translation should be initiated at ATG #1 for the following reasons: (1) start codons are commonly found within 10 bp of a trans-splice acceptor site (Allen et al. 2011), and (2) after trans-splicing, ATG #1 would be the first start codon in the etc-1 mRNA. If correct, 20 additional amino acids would be appended to the N-terminus of the predicted ETC-1 protein. Seven of these amino acids are similar or identical to equivalent residues at the N-terminus of UBE3C (Fig. 6b). We created 2 different GFP-tagged alleles of etc-1: (1) etc-1(tn1919[gfp::3xflag::etc-1#1]), which places GFP (and another start codon) immediately upstream of the start codon adjacent to the trans-splice acceptor site (ATG #1), and (2) etc-1(tn1920[gfp::3xflag::etc-1#2]), which places an identical tag immediately upstream of the annotated start codon (ATG #2) (Supplementary Fig. 5a). Both etc-1(tn1919) and etc-1(tn1920) express GFP (Fig. 6c and d, and Supplementary Fig. 5b and c) and make large fusion proteins that approximate the predicted sizes of the GFP::ETC-1 fusion proteins (∼150 kDa, see below) (Fig. 6e, lanes 1 and 2). We conclude that a start codon immediately adjacent to the etc-1 5′ trans-splice site is capable of initiating translation in vivo.
etc-1(tn1919) and etc-1(tn1920) express GFP::ETC-1 (labeled GFPF::ETC-1) in the oogenic germline of hermaphrodites, where it is both cytoplasmic and nucleoplasmic (Fig. 6c and d). We observed no significant difference in the amount of cytoplasmic GFP::ETC-1 in the germlines of etc-1(tn1919) (n = 11) and etc-1(tn1920) (n = 8) adult hermaphrodites (P > 0.1 using a Student’s t-test). In addition, both alleles express GFP::ETC-1 in embryos (Fig. 6c and d) and in a variety of somatic tissues, including the intestine, the spermatheca, and unidentified cells near the pharynx (arrow in Supplementary Fig. 5b and c). Interestingly, etc-1(tn1920) adult hermaphrodites (n = 3) express 2.4-times more GFP::ETC-1 in the unidentified cells near the pharynx than etc-1(tn1919) adult hermaphrodites (n = 7) (P < 1 × 10−6 using a Student’s t-test). This result suggests there could be differential start codon usage in some cell types. In etc-1(tn1920) animals, the start codon at the beginning of the GFP coding sequence is 60 bp downstream of ATG #1 and in the same relative position as the annotated start codon (ATG #2) in the endogenous etc-1 gene (Supplementary Fig. 5a). We hypothesized that etc-1(tn1920) animals might be able to initiate GFP::ETC-1 translation both at the start codon used by etc-1(tn1919) animals (150.5 kDa GFP::ETC-1) and at the start codon located at the beginning of the GFP coding sequence (148 kDa GFP::ETC-1). Consistent with this idea, some of the GFP::ETC-1 made by etc-1(tn1920) animals appears to migrate slightly faster on western blots (Fig. 6e, lane 2). These observations suggest that ETC-1 translation can initiate at ATG #1 in most tissues, including the germline, while leaving the possibility that ETC-1 translation also initiates at ATG #2 in some somatic cells. However, it is also possible that the slightly different GFP expression patterns seen in etc-1(tn1919) and etc-1(tn1920) are caused by a difference in gene structure that we have not explicitly considered or the fact that these alleles are differently functional. As we will describe, etc-1(tn1919) (ATG #1) appears to be largely functional during the OET, while etc-1(tn1920) (ATG #2) resembles the strong loss-of-function etc-1(gk5182) allele.
etc-1 is nonessential
etc-1(RNAi) and etc-1(tm5615) deletion animals are viable and fertile; they do not have an overt phenotype in an otherwise wild-type background (Wang et al. 2013). Whereas etc-1(tm5615) is predicted to be an in-frame deletion (Fig. 6a), etc-1(gk5182) is a premature stop codon in the second exon of the etc-1 gene (Fig. 6a). This suggested to us that etc-1(gk5182) should be a strong loss-of-function allele. We therefore examined these animals for a variety of phenotypes, including fertility defects, but failed to find any obvious problems. For example, the brood size of etc-1(gk5182) hermaphrodites was no different from that of the wild type at 20°C [285.0 ± 25.2 (n = 23) for etc-1(gk5182) vs 292.8 ± 20.7 (n = 20) in the wild type; P = 0.2784, t test]. etc-1(gk5182) hermaphrodites also exhibited only low levels of embryonic lethality [0.3 ± 0.3% embryonic lethality (n = 6575) for etc-1(gk5182) vs 0.2 ± 0.3 (n = 5853) in the wild type]. We tested whether the strong loss-of-function mutation sel-10(ar41) (Supplementary Fig. 1a) would provide a sensitized background to reveal defects because SEL-10 and ETC-1 both help degrade proteins during the first meiotic division. However, etc-1(gk5182); lon-3(e2175) and etc-1(gk5182); lon-3(e2175) sel-10(ar41) hermaphrodites are essentially indistinguishable and produce large numbers of progeny [286 ± 26 larvae (n = 14) and 283 ± 29 larvae, (n = 15), respectively, at 20°C]. These brood sizes are similar to the brood sizes of lon-3(e2175) and lon-3(e2175) sel-10(ar41) hermaphrodites at 20°C (Spike et al. 2018). We examined whether etc-1(gk5182) animals are unusually sensitive to elevated temperatures because Hul5, the ETC-1 ortholog of budding yeast, facilitates the degradation of protein aggregates that form during heat stress (Fang et al. 2011). However, etc-1(gk5182) animals are homozygous viable and fertile at 25°C and can be maintained at this temperature for multiple generations. When wild-type animals are heat stressed for 3 h at 31°C, partially penetrant embryonic lethality occurs in the 24-h period immediately after the stress is applied (Huelgas-Morales et al. 2016). Using this heat stress protocol, similar percentages of embryos produced within the relevant 24-h period died when we compared animals with the etc-1(gk5182) genotype (21% lethality, n = 565 embryos, n = 12 animals) to the wild type (26% lethality, n = 463 embryos, n = 11 animals). Wild-type L4-stage larvae shifted from 20°C to 37°C for 1.5 h exhibit partially penetrant larval arrest and lethality (Zevian and Yanowitz 2014). Using this protocol, similar percentages of wild-type (54%, n = 268) and etc-1(gk5182) mutant animals (48%, n = 300) died after heat shock.
To unambiguously determine whether ETC-1 is important for fertility or viability, we used CRISPR-Cas9 genome editing to generate the etc-1(tn2077) null allele, which deletes the entire etc-1 open reading frame (Fig. 6a). etc-1(tn2077) mutants are viable and fertile at all temperatures tested (15°C, 20°C, and 25°C), confirming that etc-1 is a nonessential gene in C. elegans under standard laboratory conditions. Like etc-1(gk5182) hermaphrodites, etc-1(tn2077) hermaphrodites exhibit negligible embryonic lethality [0.3 ± 0.6% embryonic lethality (n = 4304)]. However, etc-1(tn2077) hermaphrodites produce significantly lower brood sizes than etc-1(gk5182) hermaphrodites [178.8 ± 55.6 (n = 24) for etc-1(tn2077) vs 285.0 ± 25.2 (n = 23) for etc-1(gk5182); P < 0.0001, t test]. We also observed that etc-1(tn2077) hermaphrodites were sluggish and appeared to exhibit a reduced life span (D.G., unpublished results). The reduced brood size of etc-1(tn2077) hermaphrodites might be due to the production of less sperm, which is ordinarily limiting for fertility. Whether this speculation proves correct and reflects the role of ubiquitin-mediated protein degradation in germline sex determination (Starostina et al. 2007), will require further study. Although the differences between etc-1(gk5182) and the large etc-1(tn2077) deletion could in principle be due to impacts on neighboring genes, these phenotypic differences are consistent with the idea that etc-1(gk5182) possibly retains residual ETC-1 function.
etc-1 alleles exhibit a genetic interaction with the anaphase-promoting complex
Wang et al. (2013) reported that etc-1(RNAi) enhances the phenotype of mat-1(ax161ts); him-8(e1489) animals at the permissive temperature, and causes them to produce one-cell arrested embryos. This enhancement supported the idea that ETC-1 and the APC share redundant functions during the OET, including the degradation of the key cell cycle regulators IFY-1/securin and CYB-1/Cyclin B. We initially revisited this genetic interaction using etc-1(gk5182) and 2 different temperature-sensitive alleles that attenuate APC function in C. elegans: emb-30(tn377ts) and mat-1(ax161ts) (Furuta et al. 2000; Shakes et al. 2003). Contrary to expectation, we found that etc-1(gk5182); emb-30(tn377ts) and mat-1(ax161ts); etc-1(gk5182) double mutants are each homozygous viable and fertile at 15°C. To extend this result, we examined the number of larvae produced by mat-1(ax161ts); etc-1(gk5182) and mat-1(ax161ts) parents. These animals were initially derived from mat-1-balanced strains (Supplementary Table 1). For each strain, we counted the number of larvae produced by parents that were third-generation mat-1(ax161ts) homozygotes raised at 15°C. mat-1(ax161ts); etc-1(gk5182) animals produced fewer progeny (100 ± 35 larvae, n = 15 animals) than mat-1(ax161ts) animals (165 ± 46 larvae, n = 17 animals) and this difference is statistically significant (P = 0.0001). However, we did not observe that the mat-1(ax161ts); etc-1(gk5182) double mutants produced one-cell arrested embryos. Thus, the double mutant phenotype is not comparable to the embryonic arrest phenotype of mat-1(ax161ts) (Supplementary Fig. 6b) or mat-1(ax161ts); him-8(e1489) animals at 25°C (Shakes et al. 2003).
Since our phenotypic analyses suggested that etc-1(gk5182) might retain some residual function, we analyzed whether etc-1(tn2077) exhibits a stronger genetic interaction with mat-1(ax161ts). We observed that mat-1(ax161ts); etc-1(tn2077) hermaphrodites are viable and fertile at 15°C and produce embryos that can undergo multiple rounds of cell division (Supplementary Fig. 6c). Some of these embryos hatch and grow into larvae. However, mat-1(ax161ts); etc-1(tn2077) hermaphrodites produce many fewer larvae than mat-1(ax161ts); etc-1(gk5182) hermaphrodites at 15°C. First-generation homozygotes derived from mat-1(ax161ts)/tmC18; etc-1(tn2077) parents produced, on average, 28 ± 19 larvae (n = 18). Second-generation homozygotes were also fertile and produced a similarly small number of larvae [24 ± 14 larvae (n = 36)]. However, dead embryos were also observed among the progeny of mat-1(ax161ts); etc-1(tn2077) hermaphrodites, and dead embryos were typically more common than hatched larvae when both of these were counted (n = 12 of 18 second-generation egg lays). Although we did not observe the one-cell arrest phenotype or the oocyte defects that were previously described (Supplementary Fig. 6), these observations nonetheless confirm that mat-1(ax161ts) embryonic lethality is enhanced by etc-1(tn2077), albeit at a later stage of embryogenesis that may result from a defect in mitotic cell division.
GFP-fusion proteins are partially proteolyzed in etc-1(gk5182) animals
The PUF-3::GFP, PUF-11::GFP, CYB-1::GFP, and IFY-1::GFP fusion proteins each contain 3 FLAG epitope tags in addition to the fluorescent protein tag (Supplementary Fig. 1c, Sarov et al. 2012). We used an antibody that recognizes the FLAG tag to examine each fusion protein by western blot in wild-type and etc-1(gk5182) hermaphrodites. Full-length PUF-3::GFP, PUF-11::GFP, and CYB-1::GFP fusion proteins migrate as expected in lysates made from both wild-type and etc-1(gk5182) hermaphrodites (Fig. 7a, lanes 1–6), while the IFY-1::GFP fusion protein migrates slightly slower than expected (Fig. 7c, lanes 1 and 2). However, in each etc-1(gk5182) lysate, a second band is also recognized by the anti-FLAG antibody (Fig. 7a, lanes 2, 4, and 6, and Fig. 7c, lane 2). This etc-1(gk5182)-specific band only accumulates when a GFP fusion protein is present (Supplementary Fig. 7a, compare lanes 2–7 with lanes 1 and 8) and can be recognized by a GFP-specific antibody (Supplementary Fig. 7b, lanes 2, 4, and 6). Depending on the GFP fusion protein, it migrates slightly faster (Fig. 7a, lanes 2, 4, and 6) or slower (Fig. 7c, lane 2) than a 40-kDa molecular weight marker. But in each case, the etc-1(gk5182)-specific band is predicted, based on size, to encompass the entire 31–32 kDa GFP::FLAG tag (Supplementary File 2). We estimate that these GFP::FLAG fragments account for 10–20% of the total signal in each etc-1(gk5182); puf-3(tn1820[puf-3::gfp::3xflag]) lane, 4–11% of the total signal in each etc-1(gk5182); puf-11(tn1824[puf-11::gfp::3xflag) lane, and 4–10% of the total signal in each etc-1(gk5182); cyb-1(tn1806[cyb-1::gfp::3xflag]) lane (Fig. 7a, lanes 2, 4, and 6). GFP::FLAG fragments appear to be less abundant in etc-1(gk5182); ddIs128[ify-1::gfp::3xflag] animals relative to the full-length IFY-1::GFP fusion protein (Fig. 7c), but we could not make an accurate assessment of this difference because the full-length fusion protein and fragment were not in the linear range of detection at the same time. These observations suggest that the GFP fluorescence observed in etc-1(gk5182) embryos (Fig. 5a–d and g–j) may derive from GFP::FLAG fragments rather than full-length PUF-3::GFP, PUF-11::GFP, CYB-1::GFP, or IFY-1::GFP fusion proteins.
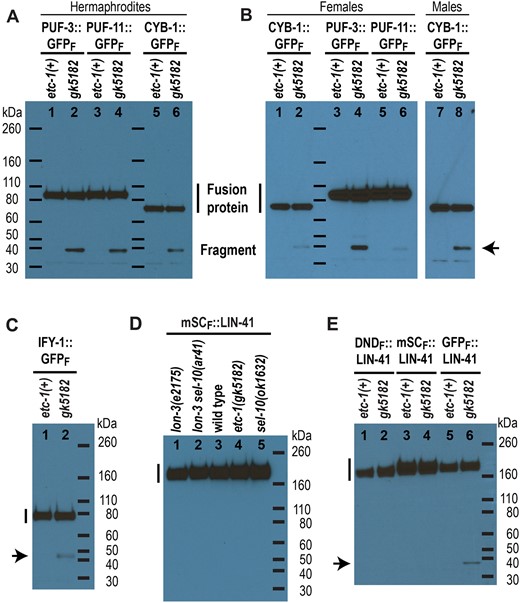
GFP-tagged fusion proteins are partially proteolyzed in etc-1(gk5182) animals. a–e) Western blots with a FLAG-specific primary antibody. Three FLAG tags are located at the extreme C-terminus of PUF-3::GFP, PUF-11::GFP, CYB-1::GFP, and IFY-1::GFP and in-between LIN-41 and each fluorescent protein (mSCARLET, DENDRA, or GFP). The predicted molecular mass of each full-length fusion protein (vertical line) is ∼89 kDa for each PUF::GFP fusion protein (a and b), 73 kDa for CYB-1::GFP (a and b), 61 kDa for IFY-1::GFP (c), and 154–156 kDa for each LIN-41 fusion protein (d and e), and the same set of molecular weight markers (horizontal lines) were used in (a) and (b). A partially proteolyzed GFP::FLAG-containing fragment >32 kDa (arrow) accumulates in the etc-1(gk5182) animals that express a GFP fusion protein (a–c, and e). The protein lysates were made from adult hermaphrodites (a, c–e), adult females (b), or adult males (b), as indicated.
Our observations of GFP fluorescence in living etc-1(lf) animals suggest that etc-1(gk5182) perturbs protein degradation during the OET (Fig. 5). However, if the GFP::FLAG fragments generated from each fusion protein accumulate prior to this time (e.g. during oogenesis), they might simply become easier to visualize when the full-length fusion protein is degraded during the OET. We examined whether GFP::FLAG fragments are generated from the PUF-3::GFP, PUF-11::GFP, and CYB-1::GFP fusion proteins in unmated etc-1(gk5182); fog-2(oz40) females to address this possibility. Development of the oogenic germline is normal in fog-2 mutant females, but due to the absence of the major sperm protein signal from sperm (McCarter et al. 1999; Miller et al. 2001), the rate of oocyte meiotic maturation is substantially decreased, and fertilization and embryo formation are prevented. We found that GFP::FLAG fragments are generated from each fusion protein in etc-1(gk5182); fog-2 females (Fig. 7b, lanes 2, 4, and 6; see Supplementary Fig. 7d for a longer exposure). Relative to the amount of each full-length fusion protein, however, GFP::FLAG fragments appear to be considerably less abundant in unmated etc-1(gk5182); fog-2 females than in etc-1(gk5182) hermaphrodites (compare Fig. 7a and b) or mated etc-1(gk5182); fog-2 females (compare Fig. 7b and Supplementary Fig. 7c and d). This was most noticeable for the GFP::FLAG fragments derived from CYB-1::GFP and PUF-11::GFP. These observations suggest that many of the GFP::FLAG fragments produced by etc-1(gk5182) mutant hermaphrodites and mated females are generated when each GFP fusion protein is degraded during the OET.
GFP::FLAG fragments also accumulate in etc-1(gk5182); cyb-1(tn1806); fog-2(oz40) adult males (Fig. 7b, lane 8). Endogenous CYB-1 is abundant in the male germline and eliminated toward the end of meiosis (Yoon et al. 2017). CYB-1::GFP exhibits a similar pattern of accumulation in fog-2 males (Supplementary Fig. 8a and b). In etc-1(gk5182); fog-2 males, however, a GFP signal perdures past the stage where CYB-1::GFP is normally eliminated (Supplementary Fig. 8c and d). Much of this signal localizes to residual bodies (Supplementary Fig. 8e and f). Residual bodies form at the end of the second meiotic division and receive cellular materials that are not required during subsequent stages of spermatogenesis (Ward et al. 1981; reviewed in Chu and Shakes 2013). It seems likely that, as in etc-1(gk5182) embryos, the GFP fluorescence seen in etc-1(gk5182) residual bodies derives from GFP::FLAG fragments that are formed when CYB-1::GFP is degraded during male meiosis.
etc-1 may promote proteasome processivity
Hul5 and UBE3C, the yeast and human orthologs of ETC-1, are proteasome-associated ubiquitin ligases that help the proteasome degrade difficult substrates (Crosas et al. 2006; Fang et al. 2011; Kuo and Goldberg 2017; Gottlieb et al. 2019). In this context, Hul5 and UBE3C are thought to be important for the processive degradation of proteins that are stalled on the proteasome. This hypothesis was based, in part, on experiments with GFP fusion proteins. In the absence of Hul5, or after UBE3C knockdown, the proteasomal degradation of substrates consisting of an unstable domain fused with the much more stable GFP domain is incomplete. This results in the accumulation of stable GFP-containing fragments of the original fusion protein (Aviram and Kornitzer 2010; Martínez-Noël et al. 2012; Chu et al. 2013). The experiments described in the previous section strongly suggest that ETC-1 plays a similar role in C. elegans. We added to these observations by examining additional GFP and non-GFP-tagged fusion proteins in etc-1(lf) hermaphrodites, as described below.
GFP::ETC-1 fusion proteins
Neither GFP::ETC-1 fusion protein is partially processed to a substantial extent (Fig. 6e, lanes 1 and 2). To determine whether either GFP-tagged allele is functional in vivo, we examined whether PUF-3::GFP is partially processed in etc-1(tn1919) and etc-1(tn1920) hermaphrodites. GFP::FLAG fragments accumulate in PUF-3::GFP-expressing etc-1(tn1920) animals (Fig. 6e, lane 6) and are similar in abundance to the GFP::FLAG fragments seen in etc-1(gk5182) animals (Fig. 6e, lane 4). etc-1(tn1920) therefore appears to be a strong loss-of-function mutation. Only a very small amount of the PUF-3::GFP-derived GFP::FLAG fragment accumulates in etc-1(tn1919) animals (Fig. 6e, see the longer exposure of lane 5), suggesting that etc-1(tn1919) retains a significant amount of function in the C. elegans germline. It is not clear why GFP::ETC-1-derived GFP::FLAG fragments do not substantially accumulate in etc-1(tn1920) animals. Possibly ETC-1 itself is not a substrate for proteasomal degradation. Alternatively, it is possible that these fragments accumulate to a level that is below our detection threshold. In this regard, it is worth noting that GFP::ETC-1 is expressed at much lower levels than PUF-3::GFP (Fig. 6e).
RNP-8::GFP fusion proteins
We created a GFP-tagged allele of rnp-8 (Supplementary Fig. 1c; RNP-8::GFPF in all figures) and found that RNP-8::GFP is abundantly expressed in oocytes and early embryos (Supplementary Fig. 9a). We do not know whether this GFP-tagged allele of rnp-8 (rnp-8(tn1860[rnp-8::gfp : 3xflag])) is functional in vivo. Although RNP-8 is thought to be degraded in response to oocyte maturation (Kim et al. 2010), RNP-8::GFP is not strongly downregulated during the OET (Supplementary Fig. 9a). GFP fluorescence is not obviously increased in rnp-8(tn1860); etc-1(gk5182) animals compared to etc-1(+) control animals (Supplementary Fig. 9a and b). Nevertheless, a small proportion of the RNP-8::GFP fusion protein made by rnp-8(tn1860) is partially processed into GFP::FLAG fragments in etc-1(gk5182) hermaphrodites (Supplementary Fig. 7c, lanes 7 and 8); however, this might represent a low level of turnover at other stages of germline development distinct from the OET.
LIN-41 fusion proteins
Only full-length mSCARLET::LIN-41 fusion proteins accumulate to a substantial level in wild-type, etc-1(gk5182) and sel-10(lf) hermaphrodites (Fig. 7d). We did not detect mSCARLET::FLAG or DENDRA::FLAG fragments at elevated levels in etc-1(gk5182), sel-10(ar41), or sel-10(ok1632) animals (Fig. 7d; Supplementary Fig. 7e). This is consistent with the observation that the levels of fluorescence appear to be the same in embryos made by wild-type and etc-1(gk5182) animals that express mSCARLET::LIN-41 (Fig. 5e and f) and the conclusion that the etc-1(gk5182) mutation does not perturb the SEL-10-mediated degradation of mSCARLET::LIN-41 or DENDRA::LIN-41 that we documented using fluorescence microscopy (Figs. 1a and b, and 2). We tested whether GFP::LIN-41 would behave in the same way by crossing our original GFP-tagged lin-41(tn1541) allele (Supplementary Fig. 1b; GFPS::LIN-41 below and in all figures) into an etc-1(gk5182) genetic background. However, we found that GFP is not appropriately downregulated in the embryos of lin-41(tn1541); etc-1(gk5182) hermaphrodites compared to lin-41(tn1541); etc-1(+) controls (Supplementary Fig. 9c and d). These observations suggest that GFPS::LIN-41 is partially processed in etc-1(gk5182) hermaphrodites and imply that GFP-tagged fusion proteins might be more recalcitrant than other fusion proteins to processivity-challenged proteasomes.
To rigorously investigate these possibilities, we created new lin-41 alleles that express a GFP::FLAG-tagged LIN-41 fusion protein (GFPF::LIN-41). We isolated 2 independent insertions that were given different allele names [lin-41(tn2054[gfp::3xflag::lin-41]) and lin-41(tn2055[gfp::3xflag::lin-41]), see Supplementary Fig. 1b]; these 2 alleles are molecularly identical and were used interchangeably in our experiments. Interestingly, GFP fluorescence in GFPF::LIN-41-expressing worms is much brighter than in GFPS::LIN-41-expressing worms (compare Supplementary Fig. 8e and g), possibly because the mRNA sequence that encodes the GFP::3xFLAG tag has a much higher codon adaptation index (CAI = 0.92) than the sequence that encodes the GFP::S tag (CAI = 0.28) (Redemann et al. 2011). More than half of the GFPF::LIN-41-expressing animals also exhibit a defect in egg laying (Egl phenotype) as adult hermaphrodites at 20°C (Supplementary Fig. 9h). A similar phenotype is observed when LIN-41 is over-expressed in somatic cells during the fourth larval (L4) stage by interfering with let-7 microRNA regulation [e.g. using the lin-41(xe11gf) allele described by Ecsedi et al. 2015]. With respect to our tagged alleles of lin-41, however, this defect uniquely characterizes the GFP::FLAG-tagged alleles, as most GFPS::LIN-41 (Supplementary Fig. 9f), mSCARLET::LIN-41, and DENDRA::LIN-41-expressing adults do not have an Egl phenotype. Despite these intriguing differences, the temporal expression pattern and regulation of GFPF::LIN-41 strongly resembles GFPS::LIN-41. Most importantly, GFPF::LIN-41-expressing animals exhibit higher levels of GFP fluorescence in the early embryos made by sel-10(ok1632) and etc-1(gk5182) mutants relative to otherwise wild-type hermaphrodites (Fig. 5k and l; Supplementary Fig. 9i–n). Interestingly, the subcellular localization pattern of GFPF::LIN-41-derived GFP fluorescence is distinctly different in the early embryos made by sel-10(ok1632) and etc-1(gk5182) mutant hermaphrodites (Supplementary Fig. 9k and m). In sel-10(ok1632) embryos, GFP fluorescence is primarily cytoplasmic and appears to be enriched in RNP particles called P granules (Supplementary Fig. 9m). Localization to embryonic P granules is also observed when LIN-41 degron sequences are disrupted in GFPS::LIN-41 (Spike et al. 2018). In etc-1(gk5182) embryos, however, GFP fluorescence does not localize to P granules and is slightly enriched in the nucleus (Supplementary Fig. 9k). These observations are consistent with the idea that GFPF::LIN-41 is partially processed into a GFP-containing protein fragment that is largely responsible for the excess fluorescence observed in early etc-1(gk5182) embryos.
The only difference between GFPF:::LIN-41, mSCARLET::LIN-41, and DENDRA::LIN-41 is the fluorescent protein fused to LIN-41 (Supplementary Fig. 1b). We examined all of these proteins in etc-1(gk5182) and etc-1(+) hermaphrodites by western blot, and confirmed that GFP::FLAG fragments accumulate in GFPF:::LIN-41-expressing etc-1(gk5182) adults at elevated levels relative to etc-1(+) animals (Fig. 7e). A longer exposure of the same western blot revealed low levels of GFP::FLAG fragments even in etc-1(+) hermaphrodites (Supplementary Fig. 7f, lane 5). However, etc-1-dependent fragments derived from the mSCARLET::LIN-41 and DENDRA::LIN-41 fusion proteins were not observed. We conclude that etc-1(gk5182) specifically disrupts the proteolysis of the GFP-containing LIN-41 fusion proteins (GFPF::LIN-41 and GFPS::LIN-41) and speculate that tagging LIN-41 with GFP creates a LIN-41 fusion protein that is more difficult to degrade. Consistent with this idea, GFP is known to be a particularly stable fluorescent protein that can be difficult for the proteasome to degrade (Khmelinskii et al. 2016; Reichard et al. 2016; Bragança and Kraut 2020). Based on these observations, we propose that fusion with GFP is an important cause of the proteolysis defects observed in etc-1(gk5182) animals (Figs. 5 and 7). In this context, we note the study that identified and described ETC-1 as a target-specific E3 ubiquitin ligase in C. elegans (Wang et al. 2013) relied on GFP fusion proteins and monitored protein degradation by looking exclusively at fluorescence. Our observations suggest, instead, that the C. elegans etc-1 gene has the same type of activity described for orthologous genes in yeast and humans (Aviram and Kornitzer 2010; Martínez-Noël et al. 2012; Chu et al. 2013) and that the ETC-1 protein promotes proteasome processivity, which may be especially important during the OET when many proteins are targeted for efficient proteasomal degradation.
Discussion
Proteolysis and translational repression limit the expression of RNA-binding proteins during the OET
Many of the oocyte-expressed proteins degraded during the OET in C. elegans are RNA-binding proteins that regulate translation (reviewed by Robertson and Lin 2013, 2015). These RNA-binding proteins include LIN-41 and the Pumilio/FBF-family RNA-binding proteins PUF-3 and PUF-11. PUF-3 and PUF-11 function redundantly, repress the translation of specific targets in oocytes, and play important roles in germline stem cells and embryonic development in C. elegans (Hubstenberger et al. 2012; Haupt et al. 2020). Since PUF-3 and PUF-11 appear to be degraded soon after meiotic maturation (Fig. 3), it seems likely that the maternal-effect embryonic lethality caused by an absence of both proteins is due to mRNA translation defects in developing oocytes. Interestingly, the timing and regulation of PUF-3 and PUF-11 degradation appear to be temporally and molecularly coordinated with meiotic maturation and strongly resemble what we have described for LIN-41 (Spike et al. 2018 and Fig. 3). The resemblances include a requirement for active CDK-1 (Fig. 4), which is essential for entry into meiotic M-phase (Boxem et al. 1999; Burrows et al. 2006), and independence from APC ubiquitin ligase subunits that promote the subsequent metaphase-to-anaphase transition (Furuta et al. 2000; Shakes et al. 2003). Additional connections between LIN-41, PUF-3, and PUF-11 include the identification of each protein and mRNA in purified LIN-41 RNPs, and the identification of LIN-41 and PUF-3/11 as factors important for the 3′-UTR-mediated translational repression of a common target, the Rbfox-related RNA-binding protein SPN-4 (Hubstenberger et al. 2012; Tsukamoto et al. 2017). These disparate observations suggest that LIN-41, PUF-3, and PUF-11 might collaborate and function, at least in part, as components of a regulatory RNP to inhibit the translation of SPN-4 in developing oocytes. Previously, we proposed that LIN-41 is inactivated as a translational repressor prior to its degradation (Spike et al. 2018). Since cdk-1(RNAi) does not reduce or eliminate SPN-4::GFP expression in early embryos (Tsukamoto et al. 2017), we speculate that PUF-3 and PUF-11 are likewise inactivated prior to their degradation. Thus, we suggest that the function of a LIN-41/PUF-3/PUF-11 RNP is downregulated through both CDK-1-dependent and -independent mechanisms near the end of oogenesis.
ETC-1, the APC, and proteasome processivity during the OET
Wang et al. (2013) identified and described ETC-1 (UBE Three C), the C. elegans UBE3C ortholog, as a HECT-type E3 ubiquitin ligase that promotes the degradation of the essential cell cycle proteins IFY-1/Securin and CYB-1/Cyclin B1 during the OET. Degradation was monitored using a GFP fusion protein (IFY-1::GFP or GFP::CYB-1), immunoprecipitation assays clearly documented a specific interaction between the GFP fusion protein and ETC-1, and in vitro assays confirmed that ETC-1 is able to interact with, and ubiquitylate, each target protein. These findings were consistent at the time with the interpretation that ETC-1 functions as an E3 ubiquitin ligase. However, they are also compatible with our results that suggest instead that ETC-1 functions as an E4 enzyme that mediates ubiquitin chain elongation for the processive degradation of proteins already targeted for ubiquitin conjugation by E3 enzymes—a function shown for Hul5 in budding yeast (Crosas et al. 2006). When combined with the observation that etc-1(RNAi) enhanced the one-cell arrest phenotype of mutants with a compromised APC [e.g. mat-1(ax161ts)], the biochemical and cell biological observations of Wang et al. (2013) appeared to support a simple and sensible model: that ETC-1 and the APC target the same C. elegans proteins (e.g. IFY-1 and CYB-1) for ubiquitination and degradation during the OET. We revisited the genetic interaction between etc-1 and the APC, at least in part, because our new results suggest that ETC-1 is not a substrate-specific E3 ligase and make it seem unlikely that ETC-1 plays a prominent role in the degradation of endogenous IFY-1 or CYB-1. Rather, our findings suggest that etc-1 mutants have difficulty degrading GFP-fusion proteins. The fact that etc-1(tn2077) null and etc-1(gk5182) loss-of-function mutants exhibit minimal embryonic lethality is consistent with this idea. Using etc-1(tn2077), a molecular null mutation that deletes the entire etc-1 open reading frame, we confirmed that the embryo lethal phenotype of mat-1(ax161ts) is substantially enhanced at 15°C in the absence of ETC-1. However, because the embryos produced by mat-1(ax161ts); etc-1(tn2077) hermaphrodites are primarily multicellular, this genetic interaction may reflect a defect in mitotic cell division rather than a defect in the OET.
The in vivo dynamics of GFP-fusion protein degradation in the absence of ETC-1
Our analyses of several different GFP fusion proteins, including GFP fusions with IFY-1 and CYB-1, provide a clear explanation for the apparent perdurance of each protein in the absence of ETC-1. It appears that the addition of a GFP moiety to several proteins that are rapidly degraded during the OET, such as IFY-1, CYB-1, PUF-3, PUF-11, or LIN-41, creates fusion proteins that are incompletely degraded in the absence of etc-1 function. Incomplete degradation results in the accumulation of a partial GFP-containing fragment in embryos that is easily confused for the full-length GFP fusion protein when only fluorescence is examined. Evidence that the GFP moiety is a determining factor is derived from the analysis of LIN-41 fusion proteins. LIN-41 fusion proteins containing one of 3 different fluorescent proteins, mSCARLET, DENDRA, or GFP, were examined and the only fusion protein that was incompletely degraded in an ETC-1-dependent manner was GFP::LIN-41 (Fig. 7e). Our experiments do not demonstrate, however, that the fused portion of the C. elegans protein is unimportant for determining whether substrate processivity is substantively reduced in the absence of ETC-1. For example, the GFP::ETC-1 fusion protein made by the loss-of-function etc-1(tn1920) allele may not be partially processed (Fig. 6e). Although it is tempting to speculate that this is because GFP::ETC-1 is not unstable during the OET (Fig. 6d), some of our other experiments indicate that fragments can be generated even when proteins appear to be relatively stable. For example, RNP-8::GFP is partially processed to a small extent in etc-1 mutants even though it is not rapidly degraded during the OET (Supplementary Figs. 6c and 8b). Likewise, the behaviors of PUF-3::GFP, PUF-11::GFP, and CYB-1::GFP in unmated females (Fig. 7b) argue for a more nuanced view. It seems likely that the proteasome helps degrade or “turn over” each GFP fusion protein to some extent in the adult germlines of unmated females and hermaphrodites. However, because each GFP-tagged protein is relatively stable prior to the OET, many fewer fragments are formed in females in the absence of etc-1 function. We suggest that the properties conferred by each fused C. elegans protein likely explain why some GFP fusion proteins appear to be less sensitive to etc-1 function than others, even when both proteins are rapidly degraded during the OET (e.g. IFY-1::GFP and PUF-3::GFP in Figs. 5 and 7). Consistent with this idea, sequences outside the GFP moiety have been shown to be important for the Hul5-dependent proteolytic processing of a GFP-Pcl5 fusion protein in S. cerevisiae (Aviram and Kornitzer 2010), and it is becoming increasingly clear that the method and type of substrate ubiquitination influences proteasome processivity (Reichard et al. 2016; Bragança and Kraut 2020).
Conclusion
Our analysis of ETC-1 updates the literature to show that it plays a conserved role to promote proteasome processivity. Proteasome processivity may be critically important during the OET when many oocyte regulatory proteins are rapidly turned over. Our experiments also provide a cautionary note on the need to analyze endogenous proteins where possible, utilize multiple protein tags and, ideally, analyze proteins on western blots when studying protein degradation in vivo. Fortunately, the ease and rapidity of genome editing continues to revolutionize the ability to conduct such experiments in the C. elegans system (reviewed by Nance and Frøkjær-Jensen 2019).
Data availability
All alleles and strains (Supplementary Table 1), plasmids (Supplementary File 1), and Sanger sequencing files are available upon request. Primer sequences are provided in Supplementary File 1. Supplementary File 2 contains the amino acid and nucleotide sequences of fluorophore tags, tagged alleles, and deletion alleles created for or used in this work.
Supplemental material is available at GENETICS online.
Acknowledgments
We thank the 2 anonymous reviewers for constructive suggestions, including the suggestion that we analyze an etc-1 molecular null mutation. We are grateful to Bob Goldstein, Judith Kimble, Geraldine Seydoux, and Marcus Vargas for providing strains or reagents. Some strains were provided by the Caenorhabditis Genetics Center, which was funded by grant P40OD010440 from the NIH Office of Research Infrastructure Programs. We also thank WormBase for sequences and annotations. Gabriela Huelgas-Morales and Todd Starich provided helpful suggestions during the course of this work.
Funding
This work was supported by National Institutes of Health grant R35GM144029 (to DG).
Conflicts of interest
None declared.
Literature cited
The C. elegans Deletion Mutant Consortium.