-
PDF
- Split View
-
Views
-
Cite
Cite
Mahekta R Gujar, Lakshmi Sundararajan, Aubrie Stricker, Erik A Lundquist, Control of Growth Cone Polarity, Microtubule Accumulation, and Protrusion by UNC-6/Netrin and Its Receptors in Caenorhabditis elegans, Genetics, Volume 210, Issue 1, 1 September 2018, Pages 235–255, https://doi.org/10.1534/genetics.118.301234
- Share Icon Share
Abstract
UNC-6/Netrin has a conserved role in dorsal-ventral axon guidance, but the cellular events in the growth cone regulated by UNC-6/Netrin signaling during outgrowth are incompletely understood. Previous studies showed that, in growth cones migrating away from UNC-6/Netrin, the receptor UNC-5 regulates growth cone polarity, as observed by polarized F-actin, and limits the extent of growth cone protrusion. It is unclear how UNC-5 inhibits protrusion, and how UNC-40 acts in concert with UNC-5 to regulate polarity and protrusion. New results reported here indicate that UNC-5 normally restricts microtubule (MT) + end accumulation in the growth cone. Tubulin mutant analysis and colchicine treatment suggest that stable MTs are necessary for robust growth cone protrusion. Thus, UNC-5 might inhibit protrusion in part by restricting growth cone MT accumulation. Previous studies showed that the UNC-73/Trio Rac GEF and UNC-33/CRMP act downstream of UNC-5 in protrusion. Here, we show that UNC-33/CRMP regulates both growth cone dorsal asymmetric F-actin accumulation and MT accumulation, whereas UNC-73/Trio Rac GEF activity only affects F-actin accumulation. This suggests an MT-independent mechanism used by UNC-5 to inhibit protrusion, possibly by regulating lamellipodial and filopodial actin. Furthermore, we show that UNC-6/Netrin and the receptor UNC-40/DCC are required for excess protrusion in unc-5 mutants, but not for loss of F-actin asymmetry or MT + end accumulation, indicating that UNC-6/Netrin and UNC-40/DCC are required for protrusion downstream of, or in parallel to, F-actin asymmetry and MT + end entry. F-actin accumulation might represent a polarity mark in the growth cone where protrusion will occur, and not protrusive lamellipodial and filopodial actin per se. Our data suggest a model in which UNC-6/Netrin first polarizes the growth cone via UNC-5, and then regulates protrusion based upon this polarity (the polarity/protrusion model). UNC-6/Netrin inhibits protrusion ventrally via UNC-5, and stimulates protrusion dorsally via UNC-40, resulting in dorsally-directed migration. The polarity/protrusion model represents a novel conceptual paradigm in which to understand axon guidance and growth cone migration away from UNC-6/Netrin.
NEURAL circuits and networks are formed by intricate interactions of axonal growth cones with the extracellular environment. The secreted UNC-6/Netrin guidance cue and its receptors UNC-5 and UNC-40/DCC guide cell and growth cone migrations in a manner conserved from invertebrates to mammals (Tessier-Lavigne and Goodman 1996; Mortimer et al. 2008). In Caenorhabditis elegans, UNC-6/Netrin is expressed in cells along the ventral midline (Wadsworth et al. 1996; Asakura et al. 2007). UNC-6 controls both ventral migrations (toward UNC-6) and dorsal migrations (away from UNC-6), and unc-6 mutants have defects in both ventral and dorsal guidance (Hedgecock et al. 1990; Norris and Lundquist 2011). Ventral vs. dorsal responses to UNC-6/Netrin are mediated by expression of UNC-40 and UNC-5 on growth cones. Classically, UNC-40 was thought to mediate ventral growth toward UNC-6 (Chan et al. 1996), and UNC-5 was thought to mediate dorsal growth away from UNC-6 (Leung-Hagesteijn et al. 1992), although UNC-40 also acts in dorsal growth along with UNC-5, likely as a heterodimer (Hong et al. 1999; MacNeil et al. 2009; Norris and Lundquist 2011; Norris et al. 2014). Recent studies indicate that UNC-5 can also act in ventral migrations (Levy-Strumpf and Culotti 2014; Yang et al. 2014; Limerick et al. 2018), and might serve to focus UNC-40 localization ventrally in the cell body toward the UNC-6/Netrin source (the statistically-oriented asymmetric localization model, SOAL). Thus, the roles of UNC-40 and UNC-5 in ventral and dorsal growth are more complex than initially appreciated.
While the roles of Netrin and its receptors in axon guidance have been well-studied, the cell biological effects of Netrin signaling on the growth cone during outgrowth in vivo remain unclear. Indeed, similar axon guidance defects can result from very different perturbations of the growth cone (i.e., excess growth cone protrusion and restricted growth cone protrusion can result in similar axon guidance defects) (Norris and Lundquist 2011; Norris et al. 2014), highlighting the importance of analyzing phenotypes at the growth cone level. Here, we use the growth cones of the VD motor axons to understand the effects of UNC-6/Netrin signaling on the growth cone during growth away from UNC-6/Netrin. In early L2 larvae, the VD growth cones extend anteriorly in the ventral nerve cord, and then turn dorsally to the dorsal nerve cord to make a commissural process (Figure 1A). In wild-type commissural VD growth cones, F-actin accumulation and filopodial protrusion occur predominantly on the dorsal leading edge of the growth cone (depicted in Figure 1A and Figure 2 and Norris and Lundquist 2011). UNC-5 is required for this growth cone polarity, as F-actin accumulation and protrusion are no longer biased dorsally in unc-5 mutants (depicted in Figure 1A) (Norris and Lundquist 2011). Furthermore, UNC-5 normally inhibits growth cone protrusion, as unc-5 mutants displayed VD growth cones with increased growth cone area and longer filopodial protrusions compared to wild type (depicted in Figure 1A) (Norris and Lundquist 2011). VD axon guidance defects in unc-5 mutants result from a failure of directed dorsal growth away from UNC-6/Netrin due to abolished growth cone polarity, with some migrating ventrally back toward the UNC-6/Netrin source (Norris and Lundquist 2011). The larger more protrusive unc-5 growth cones also advance more slowly (Norris and Lundquist 2011)—an effect observed in cultured neurons in which larger, more protrusive growth cones migrate more slowly than smaller growth cones (Ren and Suter 2016).
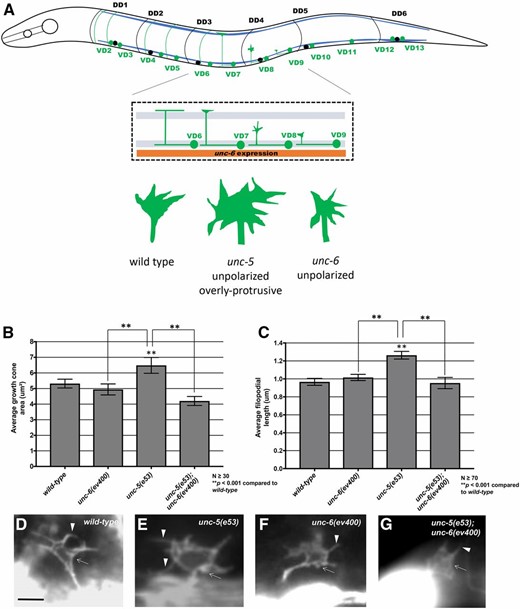
UNC-6 regulates VD growth cone protrusion. (A) A diagram showing an early L2 larval C. elegans hermaphrodite. Anterior is to the left, and dorsal is up. Blue lines represent the ventral and dorsal body wall muscle quadrants. In early L2, VD neurons (green) extend axons anteriorly in the ventral nerve cord, which then turn dorsally and migrate commissurally to the dorsal nerve cord. DD neuron cell bodies and processes are shown in black for comparison. The inset is a detail of the VD6 through VD9 region. During commissural growth, VD growth cones show a protrusive morphology with dynamic filopodial protrusions. In this diagram, VD6 has completed commissural migration, VD7 is nearly complete, VD8 is in the process of commissural growth when growth cone morphology is scored, and VD9 has just begun commissural growth. The orange box represents unc-6 expression in the VA and VB processes to the ventral of the VD and DD processes in the ventral nerve cord. Growth cone diagrams represent the structures of VD growth cones in wild-type, unc-5, and unc-6 mutants. (B and C) Graphs of the average growth cone area and filopodial length in wild-type and mutants, as described in Norris and Lundquist (2011) (see Materials and Methods). (D–G) Fluorescent micrographs of VD growth cones with Punc-25::gfp expression from the transgene juIs76 in early L2 larval hermaphrodites. Genotypes are indicated in each micrograph. Arrows indicate growth cone bodies, and arrowheads indicate filopodia. Bar in (D) represents 5 μm for all micrographs.
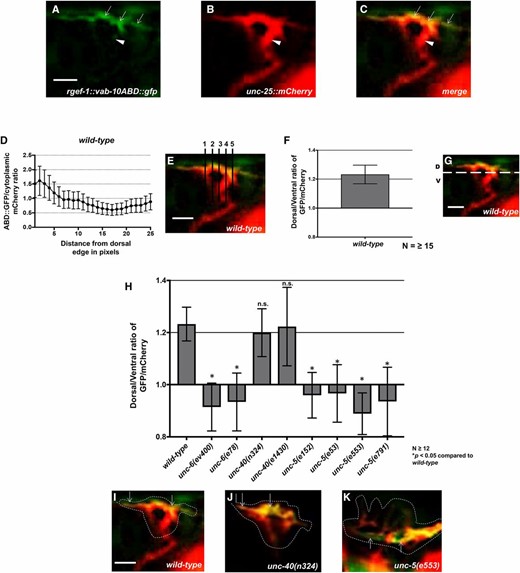
UNC-5 and UNC-6 but not UNC-40 are required for dorsal F-actin polarity. (A) VAB-10ABD::GFP accumulation at the dorsal edge of a wild-type VD growth cone (arrows). Ventral region of the growth cone with little VAB-10ABD::GFP accumulation (arrowheads). (B) mCherry growth cone volume marker. (C) Merge. Dorsal is up and anterior is left. (D–G) A representative line plot of a wild-type VD growth cone as previously described (Norris and Lundquist 2011). (D) A graph representing the pixel intensity ratio (arbitrary units) of GFP/mCherry (y-axis) against the distance from the dorsal growth cone edge. (E) For each growth cone, five lines were drawn as shown and the pixel intensity ratios were averaged (error bars represent SD). (F) The average dorsal-to-ventral ratio of GFP/mCherry in wild-type from multiple growth cones (≥15). Error bars represent the SEM of the ratios from different growth cones. (G) Growth cones were divided into dorsal and ventral halves, and the average intensity ratio of VAB-10ABD::GFP/mCherry was determined for each half and represented in (F). (H) The average dorsal-to-ventral ratio of GFP/mCherry from multiple growth cones (≥12) from different genotypes. Asterisks (*) indicate the significance of difference between wild-type and the mutant phenotype (* P < 0.05) (two-tailed t-test with unequal variance between the ratios of multiple growth cones of each genotype). Error bars represent the SEM (I–K) Representative merged images of VD growth cones with cytoplasmic mCherry in red (a volumetric marker) and the VAB-10ABD::GFP in green. Areas of overlap are yellow. Dashed lines indicate the perimeter of the growth cone. Bar, 5 μm.
unc-40 mutation had no significant effect on growth cone polarity, and protrusion was slightly but significantly reduced (Norris and Lundquist 2011). The weaker VD axon guidance defects of unc-40 reflect the relatively minor effects on the growth cone (Norris and Lundquist 2011). However, unc-5; unc-40 double mutants were found to have near wild-type levels of VD growth cone protrusion, suggesting that a functional UNC-40 was required for the overly protrusive growth cone phenotype observed in unc-5 loss of function mutants alone (Norris and Lundquist 2011). This suggests that UNC-40 has a proprotrusive role in the VD growth cone.
Constitutive activation of UNC-40 signaling (MYR::UNC-40) in VD growth cones led to small growth cones with little or no protrusion, similar to constitutive activation of UNC-5 (MYR::UNC-5) (Norris and Lundquist 2011; Norris et al. 2014). Functional UNC-5 was required for the inhibitory effects of MYR::UNC-40. This suggests that MYR::UNC-40 acts as a heterodimer with UNC-5 to inhibit protrusion, and in unc-40 mutants, UNC-5 alone was sufficient to inhibit protrusion. unc-6(ev400) null mutants had no effect on extent of VD growth cone protrusion, but did affect polarity of protrusion as well as F-actin polarity, both lost in unc-6 mutants (Norris and Lundquist 2011). Thus, UNC-6/Netrin affects VD growth cone polarity (F-actin and protrusion), but it is unclear if the effects on extent of protrusion by UNC-5 and UNC-40 involve UNC-6/Netrin.
That UNC-5 and UNC-40 cooperate to guide migrations of axons that grow toward UNC-6/Netrin indicates that the roles of these molecules are more complex than discrete “attractive” and “repulsive” functions. In this work we extend our previous findings of the effects of UNC-6/Netrin signaling on growth cone F-actin polarization and protrusion, and also show that UNC-6/Netrin regulates growth cone microtubule organization. Specifically, we demonstrate that UNC-6/Netrin drives growth cone protrusion, likely via UNC-40, that is normally restricted to the dorsal leading edge away from the UNC-6/Netrin source. In addition, we show that dorsal F-actin asymmetric accumulation also requires the Rac GTPases CED-10 and MIG-2 and the UNC-73/Trio Rac GEF. Furthermore, we find that UNC-6/Netrin and UNC-5 normally restrict growth cone microtubule + end accumulation, and, using tubulin mutants and colchicine treatment, demonstrate a requirement of MTs in growth cone protrusion. Together, these results imply that UNC-6/Netrin regulates growth away from it by regulating both the polarity of growth cone protrusion and the extent of growth cone protrusion. First, UNC-6/Netrin via UNC-5 polarizes the growth cone as reflected by F-actin accumulation and protrusion localized to the dorsal leading edge. UNC-6/Netrin and UNC-5 then restrict protrusion in ventral and lateral regions by restricting MT + end entry as well as by an MT-independent mechanism, possibly by regulating actin. Finally, UNC-6/Netrin also has proprotrusive role, via UNC-40, that normally drives protrusion at the dorsal leading edge. These results are consistent with a polarity/protrusion model of growth away from UNC-6/Netrin, in which UNC-6 polarizes the growth cone via UNC-5, and then regulates protrusion based upon this polarity.
Materials and Methods
Genetic methods
Experiments were performed at 20° using standard C. elegans techniques. Mutations used were LGI: unc-40(n324 and e1430), unc-73(rh40, e936, ev802, and ce362), tba-1(ju89); LGII: juIs76[Punc-25::gfp]. LGIV: unc-5(e53, e553, e791, and e152), unc-33(e204 and e1193), unc-44(e362, e1197, and e1260), ced-10(n1993); LGX: unc-6(ev400), unc-6(e78), mig-2(mu28), lqIs182 [Punc-25::mig-2(G16V)], lqIs170 [rgef-1::vab-10ABD::gfp]. Chromosomal locations not determined: lqIs279 and lqIs280 [Punc-25::ebp-2::gfp], lqIs296 [Punc-25::myr::unc-5], lhIs6 [Punc-25::mCherry]. lqIs279 and lqIs280 were integrants of lqEx809, which contained pCGY23-32[Punc-25::ebp-2::gfp] (a gift from Y. Jin) and Pgcy-32::cfp. The presence of mutations in single and double mutant strains was confirmed by phenotype, PCR genotyping, and sequencing. Extrachromosomal arrays were generated using standard gonadal injection (Mello and Fire 1995) and include: lqEx999 and lqEx1000 [Punc-25::myr::unc-40; Pgcy-32::yfp], lqEx1017 and lqEx1018 [Punc-25::ced-10(G12V); Pgcy-32::yfp], lqEx1192, lqEx1193 and lqEx1194[Punc-25::tba-1, Pgcy-32::yfp]; lqEx1195, lqEx1196, lqEx1197 and lqEx1198[Punc-25::tbb-1, Pgcy-32::yfp]; lqEx1199, lqEx1200, lqEx1201 and lqEx1202[Punc-25::tba-1(V323I), Pgcy-32::yfp]; lqEx1203, lqEx1204, lqEx1205 and lqEx1206[Punc-25::tba-1(E97K), Pgcy-32::yfp]; lqEx1207, lqEx1208 and lqEx1209[Punc-25::tbb-1(P220S), Pgcy-32::yfp]; lqEx1210, lqEx1211, and lqEx1212[Punc-25::tbb-1(P243L), Pgcy-32::yfp]. Multiple (≥3) extrachromosomal transgenic lines of transgenes described here were analyzed with similar effect, and data were pooled from multiple transgenes. The mig-2(mu28); ced-10(n1993M+) strain was balanced with the nT1 balancer.
Transgene construction
Punc-25::tba-1 and Punc-25::tbb-1 were made by amplifying the entire tba-1 and tbb-1 coding regions from the start to stop codons from genomic DNA. These were then placed behind the unc-25 promoter. Site-directed mutagenesis was used to generate single amino acid residue changes in the genomic regions of tba-1 and tbb-1. The tba-1 and tbb-1 variants were then placed under the control of the unc-25 promoter to analyze their effects on VD growth cone morphology and axon guidance. The coding regions of all plasmids generated by PCR were sequenced to ensure that no errors had been introduced by PCR. Sequences of all plasmids and oligonucleotide primers are available upon request.
Growth cone imaging
VD growth cones were imaged and quantified as previously described (Norris and Lundquist 2011). Unless noted, all growth cone imaging was done using the juIs76[Punc-25::gfp] integrated transgene (Jin et al. 1999). Briefly, animals at ∼16 hr posthatching at 20° were placed on a 2% agarose pad and paralyzed with 5 mM sodium azide in M9 buffer, which was allowed to evaporate for 4 min before placing a coverslip over the sample. Some genotypes were slower to develop than others, so the 16 hr time point was adjusted for each genotype. Growth cones were imaged with a Qimaging Rolera mGi camera on a Leica DM5500 microscope. Images were analyzed in ImageJ, and statistical analyses done with Graphpad Prism software. As described in Norris and Lundquist (2011), Norris et al. (2014), growth cone area was determined by tracing the perimeter of the growth cone body, not including filopodia. Average filopodial length was determined using a line tool to trace the length of the filopodium. Unless otherwise indicated, ≥25 growth cones were analyzed for each genotype. These data were gathered in ImageJ and entered into Graphpad Prism for analysis. A two-sided t-test with unequal variance was used to determine significance of difference between genotypes.
Analysis of axon guidance defects
VD neurons were visualized with a Punc-25::gfp transgene, juIs76 (Jin et al. 1999), which is expressed in GABAergic neurons including the six DDs and 13 VDs, 18 of which extend commissures on the right side of the animal. The commissure on the left side (VD1) was not scored. In wild type, an average of 16 of these 18 VD/DD commissures are apparent on the right side, due to fasciculation of some of the commissural processes. In some mutant backgrounds, <16 commissures were observed. In these cases, only observable axons emanating from the ventral nerve cord were scored for axon guidance defects. VD/DD axon defects scored include axon guidance defects (termination before reaching the dorsal nerve cord or wandering at an angle >45° before reaching the dorsal nerve cord) and ectopic branching defects (ectopic neurite branches present on the commissural processes). In the case of the effect of colchicine on axon guidance with unc-5(e53) only lateral midline crossing (axons that do not extend dorsally past the lateral midline) were considered. Fisher’s exact test was used to determine statistical significance between proportions of defective axons.
Colchicine treatment
The effects of colchicine on C. elegans VD growth cones and axons were analyzed in animals grown for three generations on standard NGM agar plates containing 1 mM colchicine.
Colchicine was added to a final concentration of 1 mM from a 100 mM stock by spread plating. These plates were then allowed to dry, after which OP50 was added.
VAB-10ABD::GFP imaging
The F-actin binding domain of VAB-10/spectraplakin fused to GFP has been used to monitor F-actin in C. elegans (Bosher et al. 2003; Patel et al. 2008). We used it to image F-actin in the VD growth cones as previously described (Norris and Lundquist 2011). To control for variability in growth cone size and shape, and as a reference for asymmetric localization of VAB-10ABD::GFP, a soluble mCherry volume marker was included in the strain. Growth cones images were captured as described above. ImageJ was used image analysis to determine asymmetric VAB-10ABD::GFP localization. For each growth cone, five line scans were made from dorsal to ventral (see Results). For each line, pixel intensity was plotted as a function of distance from the dorsal leading edge of the growth cone. The average intensity (arbitrary units) and SE for each growth cone was determined. For dorsal vs. ventral comparisons, the pixel intensities for VAB-10ABD::GFP were normalized to the volumetric mCherry fluorescence in line scans from the dorsal half and the ventral half of each growth cone. This normalized ratio was determined for multiple growth cones, and the average and SE for multiple growth cones was determined. Statistical comparisons between genotypes were done using a two-tailed t-test with unequal variance on these average normalized ratios of multiple growth cones of each genotype.
EBP-2::GFP imaging
EBP-2::GFP has previously been used to monitor microtubule plus ends in other C. elegans cells including neurons (Srayko et al. 2005; Kozlowski et al. 2007; Yan et al. 2013). We constructed a transgene consisting of the unc-25 promoter driving expression of ebp-2::gfp in the VD/DD neurons. In growth cones, a faint fluorescence was observed throughout the growth cone, resembling a soluble GFP, and allowing for the growth cone perimeter to be defined. In addition to this faint, uniform fluorescence, brighter puncta of EBP-2::GFP were observed that resembled the EBP-1::GFP puncta described in other cells and neurons. For each growth cone, the perimeter and filopodia were defined, and the EBP-2::GFP puncta in the growth cone were counted. For each genotype, the puncta number for many growth cones (≥25 unless otherwise noted) was determined. Puncta number displayed high variability within and between genotypes, so box-and-whiskers plots (Graphpad Prism) were used to accurately depict this variation. The gray boxes represent the upper and lower quartiles of the data set, and the “whiskers” represent the high and low values. Dots represent major outliers. Significance of difference was determined by a two-sided t-test with unequal variance.
Data availability
Strains and plasmids are available upon request. The authors affirm that all data necessary for confirming the conclusions of the article are present within the article, figures, and tables.
Results
Functional UNC-6 is required for excess growth cone protrusion of unc-5 mutants
While UNC-40 was required for excess protrusion in unc-5 mutants, it was unclear if UNC-6/Netrin was also required, if another UNC-40 ligand was involved, or if this was a ligand-independent role of UNC-40. As shown in Figure 1, B–G, unc-6 suppressed the excess growth cone protrusion (growth cone size and filopodial length) of unc-5 mutants, similar to unc-40. These data indicate that UNC-6 and UNC-40 are both required for the excess protrusion seen in unc-5 mutants. Thus, UNC-6/Netrin inhibits protrusion via UNC-5, and, in the absence of UNC-5, stimulates protrusion via UNC-40.
UNC-40 is not involved in growth cone F-actin polarity
The F-actin binding domain of the spectraplakin VAB-10 was previously used to monitor F-actin in the VD growth cone in C. elegans (Norris and Lundquist 2011). In wild-type VD growth cones, F-actin preferentially accumulated to the leading edge of the growth cone (Figure 2, A–G and Norris and Lundquist (2011)). Most growth cone filopodial protrusion was also biased to the dorsal leading edge of the VD growth cone, correlating with F-actin accumulation. As shown previously, VAB-10ABD::GFP dorsal asymmetry in the VD growth cone was abolished in unc-6 and unc-5 mutants (Figure 2, H and K and Norris and Lundquist (2011)). In these mutant growth cones, VAB-10ABD::GFP still accumulated at the growth cone periphery, but often in lateral and even ventral positions (Figure 2K). This loss of VAB-10ABD::GFP dorsal asymmetry was accompanied by a corresponding loss of dorsal asymmetry of filopodial protrusion, which occurred all around the growth cone in unc-5 and unc-6 mutants (Norris and Lundquist 2011). However, unc-40 mutation had no effect on VAB-10ABD::GFP distribution and dorsally-biased protrusion (Figure 2, H and J and Norris and Lundquist 2011). These results suggest that UNC-40 is not required for growth cone dorsal polarity of F-actin and protrusion as are UNC-6 and UNC-5. This also suggests that while an UNC-5:UNC-40 heterodimer can function in regulating protrusion, UNC-5 can polarize the growth cone and inhibit protrusion in the absence of UNC-40.
UNC-5 and UNC-6, but not UNC-40, regulate VD growth cone EBP-2::GFP accumulation
The MT+-end binding protein EBP-2 fused to GFP has been used previously to monitor MT+ ends in embryos and neuronal processes in C. elegans (Srayko et al. 2005; Kozlowski et al. 2007; Maniar et al. 2012; Yan et al. 2013; Kurup et al. 2015). We expressed ebp-2::gfp in the VD/DD neurons using the unc-25 promoter. Puncta of EBP-2::GFP fluorescence were distributed along the length of commissural axons (arrows in Figure 3A) and in growth cones (arrowheads in Figure 3A and arrows in Figure 3, C and D). In wild-type VD growth cones, an average of two EBP-2::GFP puncta were observed in the growth cone itself (Figure 2B). These were present at the growth cone base (arrowheads in Figure 3A) as well as in the growth cone periphery (Figure 3C). These data show that in wild-type, EBP-2::GFP puncta were abundant in the axon as previously observed, but relatively rare in the growth cone.
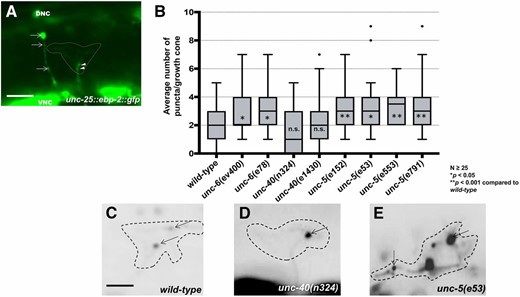
UNC-5 and UNC-6, but not UNC-40, are required to restrict growth cone EBP-2::GFP puncta. (A) A wild-type VD growth cone with Punc-25::ebp-2::gfp expression from the lqIs279 transgene. The extent of the growth cone body is highlighted by a dashed line. Arrows point to EBP-2::GFP puncta in the axons of a DD neuron. Arrowheads point to puncta in the VD growth cone. VNC is the ventral nerve cord, and DNC is the dorsal nerve cord. Bar, 5 μm. (B) Box-and-whiskers plot of the number of EBP-2::GFP puncta in the growth cones of different genotypes (≥25 growth cones for each genotype). The gray boxes represent the upper and lower quartiles, and error bars represent the upper and lower extreme values. Dots represent outliers. P values were assigned using the two-sided t-test with unequal variance. (C–E) Growth cones of different genotypes, with EBP-2::GFP puncta indicated with arrows. Dashed lines indicate the growth cone perimeter. Dorsal is up and anterior is left. Bar, 5 μm.
unc-5 and unc-6 mutants displayed significantly increased numbers of EBP-2::GFP puncta in VD growth cones and filopodial protrusions (Figure 3, B and E). In some mutant growth cones, more than eight puncta were observed, whereas wild-type never showed more than five. unc-40 mutants displayed no significant increase in EBP-2::GFP puncta accumulation (Figure 3, B and D). Sizes of EBP-2::GFP puncta in C. elegans neurons were previously found to be on the order of the smaller puncta we observe in wild type (∼100 nm) (Figure 3, A and C) (Maniar et al. 2012). In unc-5 and unc-6 mutants, we observed larger puncta (∼0.5–1 μm) (Figure 3E). We do not understand the nature of the distinct puncta sizes, but the same integrated transgene was used to analyze wild type and mutants. This suggests that puncta size and number are an effect of the mutant and not transgene variation. In sum, these studies suggest that UNC-5 and UNC-6 might be required to restrict MT + end entry into VD growth cones.
Microtubules are required for VD growth cone protrusion
Our results indicate that UNC-6 and UNC-5 restrict MT + end entry into the growth cone, which correlates with excess growth cone protrusion. This suggests that microtubules might have a proprotrusive role in the growth cone. To test this idea directly, we analyzed the effects of tubulin mutations and of the microtubule-destabilizing drug colchicine on VD growth cone morphology.
Missense mutations in the touch-receptor-specific mec-7/β tubulin and mec-12/α tubulin genes had effects on touch cell function and neurite outgrowth that did not resemble simple loss of function of the genes (Zheng et al. 2017). Phenotypic analysis and treatment with colchicine and paclitaxel led to the classification of these mutations as neomorphic, which resulted in microtubule stabilization, and antimorphic, which resulted in destabilization (Zheng et al. 2017). Neomorphic mutations resulted in ectopic neurite outgrowth from the touch receptor neurons.
tba-1 and tbb-1 encode α and β tubulins that are expressed broadly in neurons (Baran et al. 2010; Lockhead et al. 2016), and contain conserved residues altered in mec-12 and mec-7 neomorphic and antimorphic mutants. We constructed neomorphic mutations [tba-1(V323I) and tbb-1(P220S)] and antimorphic mutations [tba-1(E97K) and tbb-1(P243L)] and expressed these mutant forms in VD/DD neurons using the unc-25 promoter (Figure 4). The wild-type versions were also expressed as a control, which had no effect on VD morphology (Figure 4, A–C). Neomorphic mutations had no effect on growth cone area, but increased average filopodial length (Figure 4, A, B, and D). This is consistent with neomorphic mutations causing increased neurite outgrowth in the touch receptor neurons (Zheng et al. 2017). Antimorphic mutations resulted in reduced growth cone area and average filopodial length (Figure 4, A, B, and E), suggesting that MT destabilization reduces growth cone protrusion.
![Effects of tubulin mutations on VD growth cones. (A and B) Graphs representing VD growth cone area and average filopodial length in different backgrounds. tba-1 and tbb-1 represent transgenes expressing wild-type forms of the genes, and those expressing mutant forms are indicated. (C–F) Fluorescent micrographs of juIs76[Punc-25::gfp] expression in different backgrounds. tbb-1 represents transgenic expression of wild-type tbb-1, and transgenic expression of mutants are indicated. tba-1(ju89) is the homozygous mutant background. Bar in (C) represents 5 μm for (C–F). (G and H). Commissural VD and DD axons in wild-type and tba-1(ju89). Arrows in H indicate axon guidance defects. Bar in (G) represents 10 μm for (G and H).](https://oup.silverchair-cdn.com/oup/backfile/Content_public/Journal/genetics/210/1/10.1534_genetics.118.301234/3/m_235fig4.jpeg?Expires=1748370186&Signature=1oTSUzHwZV7gGDnzsS2gHDc2G~oyJZjcrkklZLSxsV-r84jAyr1L-p5dNOo279wr7Er8QPqtjF5Ubf2J6-QOv44cd8hkIm4HbEbN1zs~LF9QpV5sXZ7ZmuH8ijsr0Y16kE45Att8s~DwB6EXi2xAzQXtSP20HLdKEEu2GVYpixBeKA2H72MAu9IujRKhzgD04~5oYHsnwgyrKtPkAW1nk9mvrFdkAbUid4PNNVn9htZLqEjhhFGs96rzDoBa0rnaiuhavCCtA-KPrM4-QHTkQKskO4yEaKuOpw0EZ4BaovVBYiE~~pdp8z~AepLhqWd7AnohKszuvcJpVf2ElqMduw__&Key-Pair-Id=APKAIE5G5CRDK6RD3PGA)
Effects of tubulin mutations on VD growth cones. (A and B) Graphs representing VD growth cone area and average filopodial length in different backgrounds. tba-1 and tbb-1 represent transgenes expressing wild-type forms of the genes, and those expressing mutant forms are indicated. (C–F) Fluorescent micrographs of juIs76[Punc-25::gfp] expression in different backgrounds. tbb-1 represents transgenic expression of wild-type tbb-1, and transgenic expression of mutants are indicated. tba-1(ju89) is the homozygous mutant background. Bar in (C) represents 5 μm for (C–F). (G and H). Commissural VD and DD axons in wild-type and tba-1(ju89). Arrows in H indicate axon guidance defects. Bar in (G) represents 10 μm for (G and H).
The previously described tba-1(ju89) missense mutation is a gain-of-function mutation that has defects in axon guidance and synapse formation (Baran et al. 2010). tba-1(ju89) VD growth cones showed reduced growth cone area and filopodial length (Figure 4, A, B, and F), and also displayed significant VD/DD axon guidance defects (19% compared to 2% for wild type; P < 0.05) (Figure 4, G and H). The effects of tba-1(ju89) resembled those of antimorphic mutations, suggesting that tba-1(ju89) might be a dominant-negative mutation that destabilizes microtubules.
The microtubule-destabilizing compound colchicine was previously shown to suppress ectopic protrusions caused by stabilizing neomorphic tubulin mutations in the touch receptor neurons (Zheng et al. 2017). Wild-type VD growth cones displayed significantly reduced growth cone area and filopodial protrusion when animals were grown on 1 mM colchicine (Figure 5, A, B, and D), with accompanying VD/DD axon guidance defects (Figure 5, G and I). Furthermore, colchicine reduced excess growth cone protrusion in unc-5 mutants (Figure 5, A, B, and F), and partially suppressed axon guidance defects of unc-5(e53) mutants (Figure 5, J–L). In sum, these results indicate that MTs are required for protrusion in the growth cone, and suggest that axon guidance defects in unc-5 mutants are in part the result of excess protrusion due to excess MTs in the growth cones.
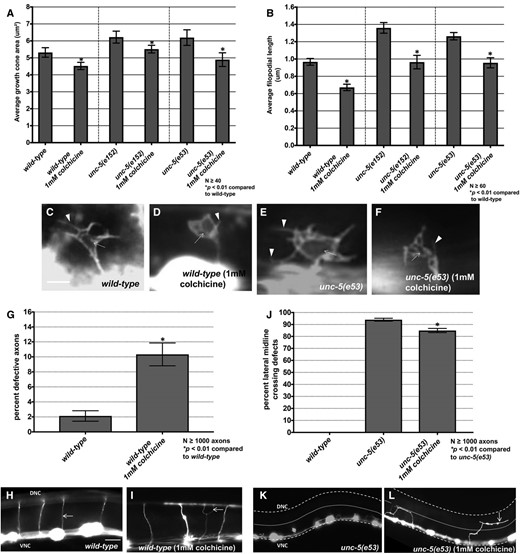
Effects of colchicine on VD growth cones. (A and B) Graphs showing growth cone area and average filopodial length in animals grown for three generations on 1 mM colchicine. (C–F) Fluorescent micrographs of VD growth cones. Arrows point to growth cone bodies, and arrowheads to filopodia. (G) A graph showing VD/DD axon guidance defects (failure to reach the dorsal cord, branching, or migration at a >45° angle to the dorsal nerve cord). (H and I) Fluorescent micrographs of VD/DD commissural axons. The arrow in (I) points to a guidance defect. (J) A graph showing the percentage of axons that fail to extend dorsally past the lateral midline (small dashed line). The ventral and dorsal nerve cords are indicated by heavier dashed lines. Bar in (H) represents 10 μm for (H, I, K, and L).
Growth cone F-actin polarity defects and excess EBP-2::GFP accumulation in unc-5 mutants is not dependent on functional UNC-6 or UNC-40
unc-6 and unc-40 suppressed the excess protrusion of unc-5 mutants (Figure 1). However, unc-5(e53); unc-40(n324) and unc-5(e53); unc-6(ev400) double mutants displayed unpolarized F-actin (Figure 6, A–D) and increased EBP-2::GFP accumulation similar to unc-5 alone (Figure 6, E–H). Thus, while UNC-6 and UNC-40 activities were required for the excess growth cone protrusion observed in unc-5 mutants, they were not required for unpolarized F-actin accumulation or for increased EBP-2::GFP accumulation. These results suggest that UNC-6 and UNC-40 have a role in protrusion that is independent of UNC-5-mediated F-actin dorsal accumulation and EBP-2::GFP accumulation. Consistent with this idea, unc-6(ev400) null mutants alone displayed loss of F-actin polarity and increased EBP-2::GFP puncta (Figure 2 and Figure 3), but not increased protrusion (Figure 1) (Norris and Lundquist 2011). This hybrid phenotype suggests that UNC-6 is required for both UNC-5-mediated inhibition of protrusion via F-actin polarity and MT restriction, and for UNC-40-mediated protrusion downstream, or in parallel to, of F-actin polarity and MT restriction. This also suggests that the F-actin accumulation we observe is not simply due to F-actin involved in lamellipodial and filopodial protrusions, but rather might represent a polarity mark that defines where protrusion can occur.
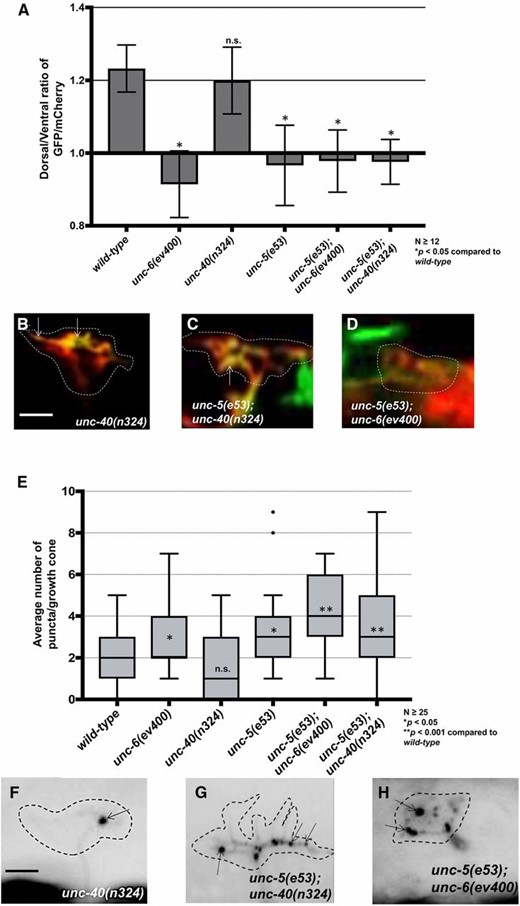
EBP-2::GFP puncta accumulation and loss of growth cone F-actin polarity in unc-5 mutants is not dependent on functional UNC-6 or UNC-40. (A) The average dorsal-to-ventral ratio of GFP/mCherry from multiple growth cones in wild-type and mutant animals as described in Figure 3. (B–D) Representative images of VD growth cones with cytoplasmic mCherry in red (a volumetric marker) and the VAB-10ABD::GFP in green as described in Figure 1. Bar, 5 μm. (E) Quantification of average number of EBP-2::GFP puncta in wild-type and mutant animals as described in Figure 2. (F–H) Fluorescence micrographs of EBP-2::GFP expression in VD growth cones. Arrows point to EBP-2::GFP puncta Bar, 5 μm
UNC-73 Rac GEF activity controls growth cone F-actin polarity
Previous studies showed that the Rac GTP exchange factor activity of UNC-73 was required to inhibit growth cone protrusion downstream of UNC-5 (Norris et al. 2014). unc-73(rh40), which specifically eliminates the Rac GEF activity of the molecule (Figure 7A) (Steven et al. 1998), resulted in excessive filopodial protrusion (Figure 7, B, C, and E, and (Norris et al. 2014)). unc-73(rh40) mutants displayed a loss of VAB-10ABD::GFP dorsal symmetry in the VD growth cone similar to unc-5 and unc-6 mutants (Figure 8, A and C). However, unc-73(rh40) mutants did not show significantly increased EBP-2::GFP puncta distribution compared to wild type (Figure 8, E and G). Thus, despite having excessively protrusive growth cones, unc-73(rh40) mutants did not display increased EBP-2::GFP puncta number. This indicates that the increased numbers of EBP-2::GFP puncta observed in unc-5 and unc-6 mutants was not simply due to larger growth cone size. This result also indicates that excess growth cone protrusion can occur in the absence of increased numbers of EBP-2::GFP puncta. unc-73(rh40) displayed strong VD/DD axon guidance defects, likely due to excess and unpolarized growth cone protrusion (Figure 7, H and J).
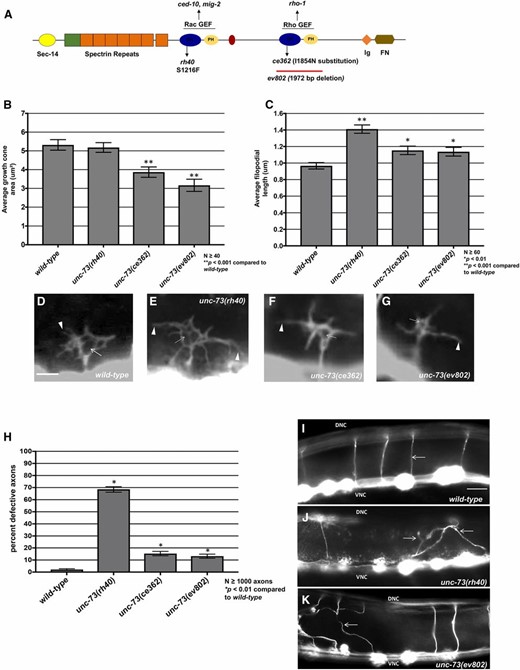
The Rac and Rho GEF activities of UNC-73/Trio affect growth cone protrusion and axon guidance. (A) A diagram of the full-length UNC-73/Trio molecule. The rh40, ce362, and ev802 mutations are indicated. (B and C) Graphs of the average growth cone area and filopodial length in wild-type and unc-73 mutants, as described in Norris and Lundquist (2011) (see Materials and Methods). Significance was determined by a two-sided t-test with unequal variance. (D–G) Fluorescence micrographs of VD growth cones with Punc-25::gfp expression from the transgene juIs76. Arrows point to the growth cone body, and arrowheads to filopodial protrusions. Bar, 5 μm for (D–G). (H) The graph quantifies VD/DD axon guidance defects in unc-73 mutants. Axons were scored as defective if they failed to reach the dorsal nerve cord or wandered >45° laterally during migration. (I–K) Micrographs of wild-type and unc-73 mutants showing axon guidance defects. Arrows point to commissural axons. Bar, 10 μm for (I–K).
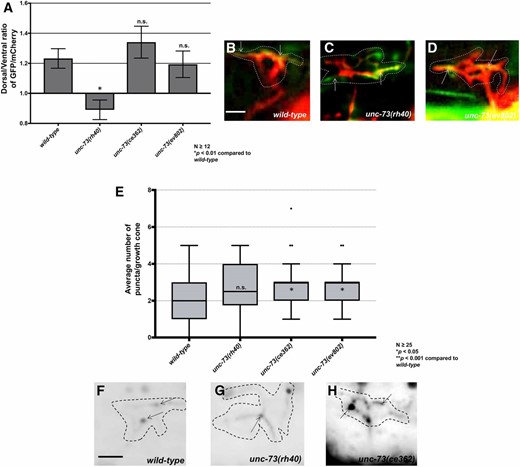
The Rac and Rho GEF activities of UNC-73/Trio affect F-actin polarity and EBP-2::GFP accumulation, respectively (A) The average dorsal-to-ventral ratio of GFP/mCherry from multiple growth cones in wild-type and mutant animals as described in Figure 1 and Figure 3. (B–D) Representative merged images of VD growth cones with cytoplasmic mCherry in red (a volumetric marker) and the VAB-10ABD::GFP in green, as in Figure 1. Areas of overlap are yellow (arrows). Bar, 5 μm. (E) Quantification of average number of EBP-2::GFP puncta in wild-type and mutant animals as described in Figure 2. (F–H) Fluorescence micrographs of VD growth cones showing EBP-2::GFP puncta (arrows). Bar in (D) represents 5 μm.
UNC-73 Rho GEF activity regulates growth cone EBP-2::GFP accumulation
The C- terminal GEF domain of UNC-73 controls the Rho GTPase (Figure 7A) (Spencer et al. 2001) and has been shown to affect motility and normal synaptic neurotransmission (Steven et al. 2005; Hu et al. 2011). unc-73(ce362) is a missense mutation in the Rho GEF domain (Figure 7A) (Williams et al. 2007; Hu et al. 2011; McMullan et al. 2012) and unc-73(ev802) is a 1972 bp deletion that completely deletes the Rho GEF domain (Figure 7A) (Williams et al. 2007). unc-73(ce362) and unc-73(ev802) displayed reduced growth cone body size, and increased filopodial length (Figure 7, B, C, F, and G) Neither unc-73(ce362) nor unc-73(ev802) had an effect on growth cone F-actin dorsal accumulation (Figure 8, A and D), but both showed a significant increase in growth cone EBP-2::GFP puncta (Figure 8, E and H). In sum, these data suggest that the Rac GEF domain of UNC-73 regulates F-actin polarity, and the Rho GEF might restrict microtubule entry. unc-73(ce362) and unc-73(ev802) displayed weak VD/DD axon guidance defects (Figure 7, H and K), which might represent the consequence of excess, but still polarized, growth cone protrusion.
The Rac GTPases CED-10 and MIG-2 affect F-actin polarity and EBP-2::GFP puncta accumulation in VD growth cones
The Rac GTPases CED-10/Rac and MIG-2/RhoG have been shown to redundantly control axon guidance (Lundquist et al. 2001; Struckhoff and Lundquist 2003). CED-10 and MIG-2 act with UNC-40 to stimulate protrusion in axons that grow toward UNC-6/Netrin (Demarco et al. 2012), and to inhibit growth cone protrusion with UNC-5-UNC-40 in the VD growth cones that grow away from UNC-6/Netrin (Norris et al. 2014). The Rac GEF TIAM-1 acts with CED-10 and MIG-2 to stimulate protrusion (Demarco et al. 2012), and the Rac GEF UNC-73/Trio acts in the antiprotrusive pathway (Norris et al. 2014).
The VD growth cones of mig-2; ced-10 double mutants resembled wild type, except that the filopodial protrusions had a longer maximal length and were longer lasting (Norris et al. 2014). This subtle phenotype might represent the fact that the molecules have roles in both proprotrusive and antiprotrusive pathways.
mig-2(mu28) and ced-10(n1993) single mutants and ced-10; mig-2 double mutants each showed significant F-actin polarity defects (Figure 9, A–D), consistent with the idea that the GEF domain of Trio affects F-actin polarity through Rac activation. Similar to unc-5; unc-40 and unc-5; unc-6 double mutants, loss of asymmetry of F-actin did not result in excess protrusion in mig-2 and ced-10 single mutants, possibly due to a proprotrusive role of the Racs independent of F-actin polarity. This again indicates the F-actin accumulation might mark the site of future protrusion rather than lamellipodial and filopodial actin itself involved in protrusion.
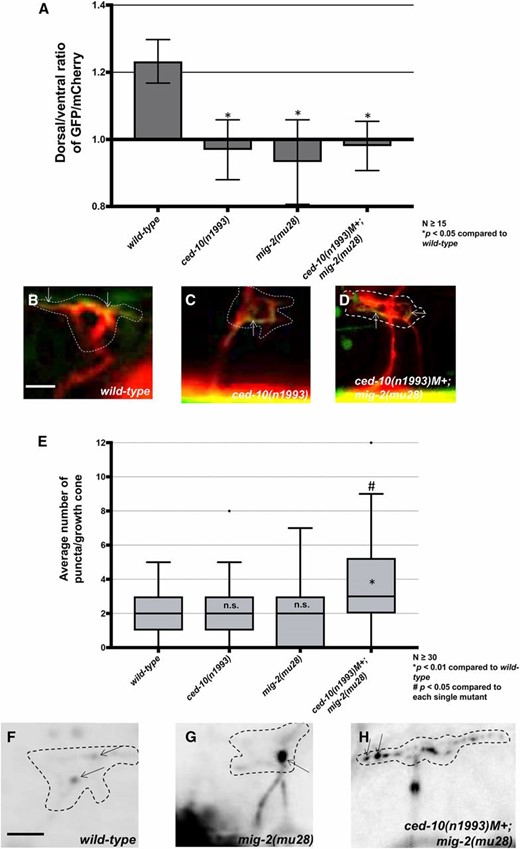
The Rac GTPases CED-10 and MIG-2 individually affect F-actin polarity and are redundant for EBP-2::GFP puncta accumulation. (A) The average dorsal-to-ventral ratio of GFP/mCherry from multiple growth cones in wild-type and mutant animals as described in Figure 1 and Figure 3. (B–D) Representative merged images of VD growth cones with cytoplasmic mCherry in red (a volumetric marker) and the VAB-10ABD::GFP in green as in Figure 1. Bar, 5 μm. (E) Quantification of average number of EBP-2::GFP puncta in wild-type and mutant animals as described in Figure 2. (F–H) Fluorescence micrographs of VD growth cones with EBP-2::GFP puncta indicated arrows. Bar, 5 μm.
ced-10 and mig-2 single mutants had no significant effect on EBP-2 distribution in the VD growth cone (Figure 9, E and H). However, ced-10; mig-2 double mutants showed a significant increase in EBP-2 puncta distribution in the VD growth cone and filopodial protrusions as compared to wild type and the single mutants alone (Figure 9, E and H). This result suggests that the Rac GTPases CED-10 and MIG-2 act redundantly in limiting EBP-2 puncta distribution in the VD growth cone. This also indicates that MIG-2 and CED-10 have a role in limiting EBP-2::GFP puncta that is independent of UNC-73/Trio Rac GEF activity.
Despite unpolarized growth cone F-actin, ced-10 and mig-2 single mutants have weak VD/DD axon guidance defects, whereas the ced-10; mig-2 double mutant has strong, synergistic guidance defects (Lundquist et al. 2001; Norris and Lundquist 2011). Possibly, perturbation of both F-actin polarization and MT + end accumulation are required for severe guidance defects in the double mutant. It is also possible that the moderate axon guidance defects in the single mutants reflects the additional, proprotrusive role of CED-10 and MIG-2 (i.e., despite unpolarized F-actin, protrusion is also inhibited, resulting in a moderate axon guidance phenotype). It is also possible that the F-actin polarity defects are less severe in single mutants and our assay lacks the resolution to detect the difference.
UNC-33/CRMP and UNC-44/ankyrin are required for F-actin polarity and restricting EBP-2::GFP from the VD growth cone
Previous studies showed that UNC-33/CRMP and UNC-44/ankyrin act downstream of UNC-5 and Rac GTPases to limit growth cone protrusion (Norris et al. 2014). unc-33 and unc-44 mutants randomized F-actin polarity similar to unc-5 and unc-73(rh40) (Figure 10, A–D). unc-33 and unc-44 also displayed a significant increase in EBP-2::GFP puncta in the growth cone and protrusions (Figure 10, E–H). Thus, UNC-33 and UNC-44 are both required for dorsal F-actin asymmetry as well as restriction of EBP-2::GFP growth cone puncta. That unc-33 and unc-44 phenotypes are similar to unc-5 is consistent with the previous genetic interactions placing UNC-33 and UNC-44 in the UNC-5 pathway.
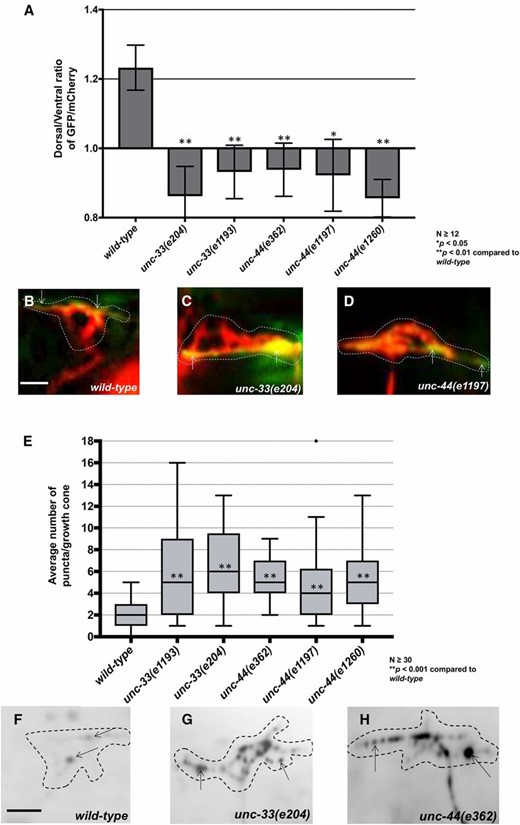
unc-33 and unc-44 mutants disrupt F-actin polarity and affect EBP-2::GFP puncta accumulation. (A) The average dorsal-to-ventral ratio of GFP/mCherry from multiple growth cones in wild-type and mutant animals as described in Figure 3. (B–D) Representative merged images of VD growth cones with cytoplasmic mCherry in red (a volumetric marker) and the VAB-10ABD::GFP in green, as in Figure 1. Bar, 5 μm. (E) Quantification of average number of EBP-2::GFP puncta in wild-type and mutant animals as in Figure 2. (F–H) Fluorescence micrographs of VD growth cones with EBP-2::GFP puncta indicate by arrows. Bar, 5 μm.
Constitutive activation of UNC-40, UNC-5, CED-10 and MIG-2 affects F-actin polarity and EBP-2 distribution
The heterodimeric receptor UNC-5-UNC-40 is required for inhibition of growth cone protrusion in growth away from UNC-6/netrin (Norris and Lundquist 2011; Norris et al. 2014). Constitutive activation of UNC-40 and UNC-5 by addition of an N-terminal myristoylation signal to their cytoplasmic domain (Gitai et al. 2003; Norris and Lundquist 2011) causes a significant decrease in VD growth cone protrusiveness, with a reduction in growth cone area and filopodial protrusions (Demarco et al. 2012; Norris et al. 2014). The Rac GTPases CED-10 and MIG-2 have been shown to act in both stimulation and inhibition of growth cone protrusion (Demarco et al. 2012; Norris et al. 2014). The constitutively activated Rac GTPases CED-10(G12V) and MIG-2(G16V) also cause an inhibited VD growth cone phenotype similar to myr::unc-40 and myr::unc-5 (Norris et al. 2014).
We assayed VAB-10ABD::GFP and EBP-2::GFP distribution in the VD growth cones of these various activated molecules. All four (MYR::UNC-5, MYR::UNC-40, CED-10(G12V), and MIG-2(G16V) showed peripheral F-actin accumulation around the entire growth cone, with the dorsal polarity lost (Figure 11, A–D). This was unexpected, as loss of unc-5, ced-10, and mig-2 each led to the same phenotype. Possibly, the gain-of-function constructs are unmasking an additional role of these molecules in stimulating F-actin accumulation not revealed by loss of function. We observed significantly fewer EBP-2::GFP puncta compared to wild type in each case (Figure 11, E–H), consistent with a normal role of the molecules in restricting EBP-2::GFP puncta.
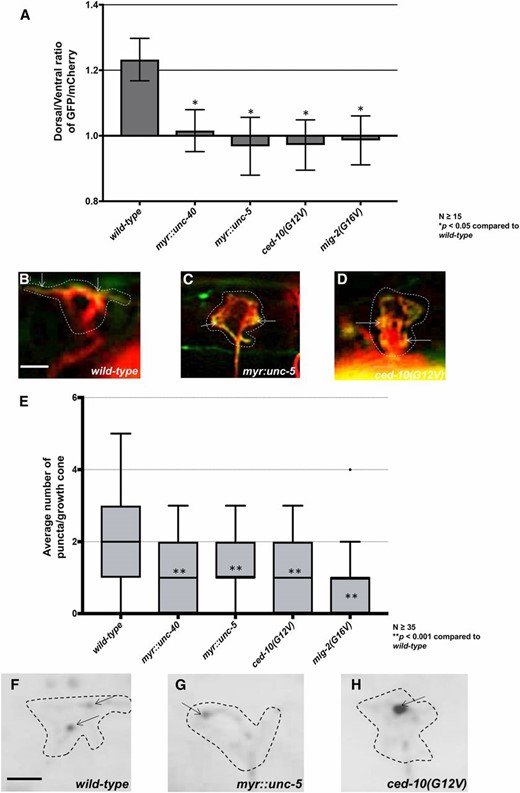
Constitutive activation of UNC-40, UNC-5, CED-10, and MIG-2 affects F-actin polarity and EBP-2 distribution. (A) The average dorsal-to-ventral ratio of GFP/mCherry from multiple growth cones in wild-type and mutant animals as described in Figure 3. (B–D) Representative merged images of VD growth cones with cytoplasmic mCherry in red (a volumetric marker) and the VAB-10ABD::GFP in green. Bar, 5 μm. (E) Quantification of average number of EBP-2::GFP puncta in wild-type and mutant animals as described in Figure 2. (F–H) Fluorescence micrographs of VD growth cones with EBP-2::GFP puncta indicated by arrows. Bar, 5 μm.
Discussion
Results presented here begin to unravel the complex cell biological processes of the growth cone that are regulated by UNC-6/Netrin during growth cone migration away from UNC-6/Netrin. These results, combined with previous studies (Norris and Lundquist 2011; Norris et al. 2014), indicate that UNC-6/Netrin regulates growth cone polarity as well as growth cone protrusion that occurs in response to this polarity (Figure 12). By monitoring F-actin accumulation in growth cones, we show that UNC-6 regulates F-actin polarity, resulting in F-actin accumulation on the dorsal leading edge of the growth cone. This site of F-actin accumulation corresponds to where filopodial protrusion occurs in the growth cone (Figure 12A). By restricting F-actin accumulation to the dorsal leading edge, UNC-6/Netrin ensures that growth cone protrusion occurs only at the dorsal leading edge and not in ventral and lateral regions. By monitoring MT + ends, we show that UNC-6/Netrin restricts MT + end accumulation in growth cones, and that MTs are required for protrusion in the growth cone (Figure 12B). By restricting MT+ ends, UNC-6/Netrin ensures that growth cone protrusion is limited and not excessive. Both of these roles of UNC-6/Netrin require the UNC-5 receptor. We also show that UNC-6/Netrin has a proprotrusive role downstream of, or in parallel to, F-actin dorsal accumulation and MT + ends that is dependent upon the receptor UNC-40 (Figure 12C). Together, these data suggest the polarity/protrusion model of directed growth cone migration away from UNC-6/Netrin. This model is exemplified by the unc-5 mutant, in which F-actin accumulates all around the growth cone with corresponding unpolarized protrusion, and more MT + ends enter the growth cone, resulting in excess protrusion (Figure 12D). These unpolarized, excessively protrusive growth cones fail to achieve directed dorsal migration away from UNC-6/Netrin. Our results are consistent with a recently described model of growth cone migration toward UNC-6/Netrin, the SOAL model (Kulkarni et al. 2013; Yang et al. 2014; Limerick et al. 2018). In both models, UNC-40-mediated protrusion in response to UNC-6/Netrin is polarized and focused by UNC-5: in growth toward UNC-6/Netrin, UNC-40 protrusive activity is focused toward the UNC-6/Netrin source; and in growth away from UNC-6/Netrin, UNC-40 protrusive activity is focused away from the UNC-6/Netrin source.
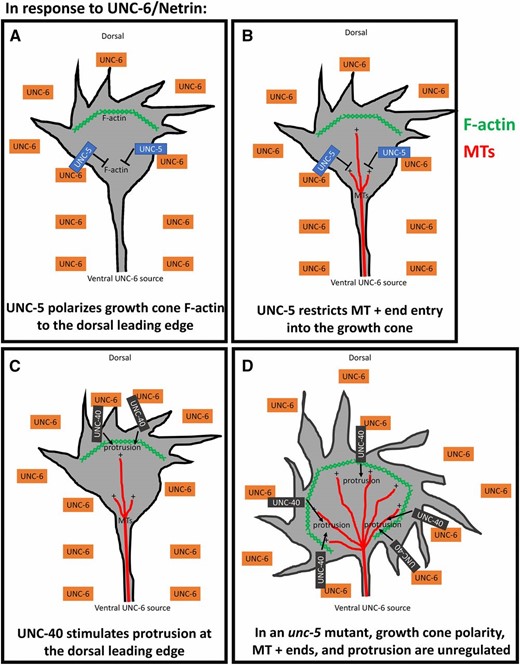
The polarity/protrusion model of growth cone migration away from UNC-6/Netrin. Our results indicate that UNC-6/Netrin controls multiple, complex aspects of growth cone behavior and morphology during growth cone migration away from UNC-6/Netrin. (A) First, UNC-6/Netrin, via UNC-5, polarizes the growth cone, resulting in F-actin accumulation (green hatches) to the dorsal leading edge and restricting it laterally and ventrally. (B) UNC-6/Netrin and UNC-5 also restrict MT + end entry (red lines) into the growth cone, which inhibits protrusion laterally and ventrally, as MTs have a proprotrusive role in the growth cone. (C) UNC-6/Netrin, via UNC-40, stimulates protrusion to the dorsal leading edge corresponding to the site of F-actin polarization. The combination of dorsal stimulation of protrusion and lateral and ventral restriction of protrusion results in directed growth away from UNC-6/Netrin. (D) In an unc-5 mutant, growth cone F-actin polarity is lost, resulting in protrusion around the perimeter of the growth cone. Also, MT + ends enter the growth cone in greater numbers, increasing the extent of growth cone protrusion. VD/DD axon guidance defects result in unc-5 mutants by a combination of unpolarized and unrestricted growth cone protrusion.
UNC-6/Netrin, via UNC-40, drives growth cone protrusion downstream of, or in parallel to, growth cone polarity and MT + end entry
We show that UNC-6/Netrin and the receptor UNC-5 regulate growth cone polarity as reflected by dorsal F-actin accumulation, and restrict MT + end entry into the growth cone. Both of these serve to limit growth cone protrusion, as protrusion occurs only dorsally at the site of F-actin accumulation, and MT + ends have a proprotrusive role. Thus, directed migration resulted from restricting protrusion to the dorsal leading edge and inhibiting it ventrally and laterally.
Both unc-6 and unc-40 mutation reduced the excess growth cone protrusion in unc-5 mutants, suggesting that UNC-6 can also stimulate protrusion via the UNC-40 receptor. However, unc-6 and unc-40 mutation did not affect F-actin accumulation or MT + end entry, as unc-5; unc-6 and unc-5; unc-40 double mutants still displayed unpolarized F-actin and excess EBP-2::GFP puncta. This indicates that UNC-6 and UNC-40 are required to stimulate growth cone protrusion downstream of, or in parallel to, F-actin and MT + ends. In other words, F-actin accumulation and MT + end entry themselves are not sufficient to drive protrusion, but rather require UNC-6 and UNC-40 to do so. This also explains why the unc-6 mutant displayed unpolarized growth cones with excess EBP-2::GFP puncta, but not excess protrusion, as UNC-6 also has proprotrusive roles downstream of, or in parallel to, F-actin accumulation and MT + end entry.
In cultured growth cones, MTs are involved in both DCC and UNC5C-mediated axon outgrowth, and DCC and UNC5C physically associate with MTs in a Netrin-dependent manner in cultured cells (Qu et al. 2013; Shao et al. 2017; Huang et al. 2018). Our results suggest a link between UNC-6/Netrin signaling and VD growth cone MTs in vivo.
F-actin accumulation might define the site of future growth cone protrusion
The unc-6; unc-5 and unc-40; unc-5 double mutants suggest that F-actin accumulation and MT + end entry can occur without excess protrusion. Thus, the F-actin accumulation we observe is not likely the result of increased F-actin due to lamellipodial and filopodial protrusion. Rather, our results suggest that F-actin accumulation in the growth cone represents a polarity mark that might define where future lamellipodial and filopodial protrusion will occur. UNC-6/Netrin and the UNC-5 receptor ensure that this future site of protrusion is located at the dorsal leading edge, resulting in directed protrusion away from UNC-6/Netrin.
VD growth cone protrusion requires microtubules
In wild-type VD growth cones, the dorsally directed filopodial protrusions are dynamically extended and retracted during outgrowth (Knobel et al. 1999; Norris and Lundquist 2011). In unc-5 mutants, filopodial protrusions are longer and longer-lasting (i.e., less dynamic than wild-type) (Norris and Lundquist 2011). Indeed, some filopodia do not retract and instead form neurites and axon branches. As a result, unc-5 growth cones fail to advance, consistent with in vitro results indicating that larger growth cones move at a slower rate (Ren and Suter 2016).
We find that unc-5 mutants display increased numbers of EBP-2::GFP puncta, representing MT + ends, that correlate with increased protrusion. We find that expression of mutant forms of tba-1 and tbb-1 predicted to destabilize microtubules (Zheng et al. 2017) caused reduced protrusion, as did treatment with the MT-destabilizing compound colchicine. Furthermore, colchicine treatment reduced protrusion in unc-5 mutants. Together, these data indicate that MT destabilization reduces growth cone protrusion, and that MTs might have a proprotrusive role in the growth cone. Expression of one tbb-1 mutant predicted to stabilize MTs resulted in significantly increased filopodial protrusion, consistent with a proprotrusive role. The previously described gain-of-function tba-1(ju89) mutation caused reduced growth cone protrusion, suggesting that it might destabilize MTs and have a dominant-negative effect.
Our results are consistent with in vitro studies of growth cones in which MT + end entry into the growth cone is tightly regulated, is intimately associated with F-actin, and is essential for protrusion and outgrowth (Lowery and Van Vactor 2009; Dent et al. 2011; Vitriol and Zheng 2012; Coles and Bradke 2015). Possibly, MT entry into growth cones serves as a conduit for transport of vesicles, organelles, and proprotrusive factors involved in actin polymerization that drive filopodial protrusion in C. elegans, such as Arp2/3, UNC-115/abLIM, and UNC-34/Enabled (Shakir et al. 2006, 2008; Norris et al. 2009). This is consistent with results from cultured growth cones showing that MT stabilization results in growth cone turning in the direction of stabilization, and MT destabilization results in growth cone turning away from MT destabilization (Buck and Zheng 2002). We do not know how MT entry relates to F-actin accumulation, but we speculate that the site of F-actin accumulation might represent where in the growth cone these proprotrusive factors are delivered and/or activated (via UNC-6/Netrin signaling through UNC-40).
The UNC-73/Trio GEF and Rac GTPases regulate F-actin and MT+ end accumulation
Rac GTPases CED-10 and MIG-2 and the UNC-73/Trio Rac GEF have been shown to play central roles in axon guidance (Steven et al. 1998; Lundquist et al. 2001; Lundquist 2003; Struckhoff and Lundquist 2003). Rac GTPases CED-10 and MIG-2 are required to both stimulate and inhibit protrusion, with distinct GEFs regulate each of these activities. TIAM-1 stimulates protrusion (Demarco et al. 2012), and UNC-73 limiting protrusion (Norris et al. 2014).
Previous results indicated the UNC-73, CED-10, and MIG-2 were required for the effects of activated MYR::UNC-5 and MYR::UNC-40 in growth cone inhibition of protrusion (Norris et al. 2014). Our results here show that ced-10 and mig-2 each affect F-actin polarity, but did not increase protrusion, consistent with the additional proprotrusive roles of these Rac GTPases. ced-10 and mig-2 were redundantly required for EBP-2::GFP restriction, suggesting that they control both F-actin accumulation and MT + end accumulation.
The Rac GEF activity of unc-73 was required for F-actin polarity, consistent with the idea that UNC-73 regulates actin during cell growth and growth cone migrations (Steven et al. 1998; Bateman et al. 2000; Lundquist et al. 2001; Wu et al. 2002). unc-73(rh40) had no effect on EBP-2::GFP accumulation in VD growth cones despite having larger, more protrusive growth cones. This suggests that the UNC-73 Rac GEF activity might inhibit protrusion by a mechanism distinct from restricting MT + end entry, possibly by affecting actin polymerization directly. Such a mechanism could involve the flavin monooxygenase (FMOs) FMO-1 and FMO-5, which were recently shown to act downstream of UNC-5 and activated Rac GTPases to inhibit VD growth cone protrusion (Gujar et al. 2017). In Drosophila, the FMO-containing MICAL molecule causes actin depolymerization by directly oxidizing actin (Hung et al. 2010, 2011). The C. elegans genome does not encode a single MICAL-like molecule containing an FMO plus additional functional domains. In C. elegans FMOs might play an analogous role to MICAL in actin regulation and growth cone inhibition.
Our results suggest that the Rho GEF activity of UNC-73 is required to restrict MT + end accumulation, but not F-actin polarity. However, the growth cones were slightly smaller with slightly increased filopodial length. This complex phenotype could reflect the role of RHO-1 in the growth cone, or could reflect that these mutations are not specific to the Rho GEF domain and might affect overall function of the molecule.
These results indicate that Rac GTPases CED-10 and MIG-2 affect both F-actin polarity and MT + end accumulation in the growth cone. The Rac GEF domain of UNC-73 might regulate MIG-2 and CED-10 in F-actin polarity, but a distinct GEF activity likely regulates MIG-2 and CED-10 in MT + end accumulation. Furthermore, the Rho GEF domain of UNC-73 might also play a role in MT + end restriction.
UNC-33/CRMP regulates F-actin polarity and MT + end accumulation
Our previous work showed that the collapsin-response-mediating protein UNC-33/CRMP and UNC-44/ankyrin are required for inhibition of protrusion by UNC-5-UNC-40 and Rac GTPases. Here, we show that UNC-33 and UNC-44, similar to UNC-5, are required for F-actin polarity and to restrict MT + end accumulation in the growth cone. CRMPs were first identified as molecules required for growth cone collapse induced by semaphorin-3A through Plexin-A and Neuropilin-1 receptors (Goshima et al. 1995; Takahashi et al. 1999). CRMP4 knockdown in cultured mammalian neurons led to increased filopodial protrusion and axon branching (Alabed et al. 2007), consistent with our findings of UNC-33/CRMP as an inhibitor of protrusion. However, hippocampal neurons from a CRMP4 knock-out mouse exhibited decreased axon extension and growth cone size (Khazaei et al. 2014).
CRMPs have various roles in actin and MT organization and function (Khazaei et al. 2014). CRMP2 promotes microtubule assembly in vitro by interacting with tubulin heterodimers and microtubules to regulate axonal growth and branching (Fukata et al. 2002). CRMP2 also binds to the kinesin-1 light chain subunit and acts as an adaptor for the transport of tubulin heterodimers as well as the actin regulators Sra-1 and WAVE into axonal growth cones (Kawano et al. 2005; Kimura et al. 2005). Furthermore, CRMP4 physically associates with in vitro F-actin (Rosslenbroich et al. 2005). In cultured DRG neurons, CRMP1 colocalizes to the actin cytoskeleton (Higurashi et al. 2012), and drives actin elongation in lamellipodia formation in cultured epithelial cells (Yu-Kemp et al. 2017). These studies indicate that CRMPs can have both positive and negative effects on neuronal protrusion, and most of the biochemical evidence indicates that CRMPs promote actin assembly and MT function.
Our results suggest that UNC-33/CRMP has a negative effect on growth cone protrusion and MT entry into growth cones, consistent with the original finding of CRMPs as antiprotrusive factors (Goshima et al. 1995; Takahashi et al. 1999). The role of UNC-44/ankyrin might be to properly localize UNC-33/CRMP as previously described (Maniar et al. 2012). As CRMPs have known roles in actin and MT organization, UNC-33 is a good candidate for a molecule that coordinates actin and MT function in the growth cone to ensure dorsal protrusion and ventral-lateral inhibition of protrusion in directed growth away from UNC-6/Netrin.
The complex role of UNC-40 in growth cone protrusion
UNC-40 was dispensable for F-actin polarity and MT + end restriction, suggesting that UNC-5 alone can mediate these responses to UNC-6/Netrin. However, UNC-40 can act as a heterodimer with UNC-5 to mediate growth away from Netrin (Hong et al. 1999; MacNeil et al. 2009), demonstrated in the VD growth cones by the inhibited growth cone phenotype of activated MYR::UNC-40, which requires functional UNC-5 (Norris and Lundquist 2011). It is unclear how these distinct activities contribute to VD growth cone outgrowth, although genetic evidence suggests that the UNC-40-UNC-5 heterodimer might be more important as the growth cone becomes more distant from the ventral UNC-6/Netrin source (MacNeil et al. 2009), consistent with the weaker VD/DD axon guidance phenotype of unc-40 mutants.
Our results demonstrate a clear role of UNC-40 in stimulating protrusion downstream of, or in parallel to, F-actin polarity and MT + end entry, normally at the dorsal leading edge, as evidenced most strongly by suppression of unc-5 excess protrusion by unc-40. The growth cones of unc-40 mutants showed reduced filopodial protrusion (Norris and Lundquist 2011), but protrusion was not entirely abolished. Thus, other molecules might act redundantly with UNC-40 to drive protrusion, and the excess protrusion of unc-5 mutants might represent a sensitized background upon which the proprotrusive effects of unc-40 are evident. Thus, UNC-40 has both proprotrusive and antiprotrusive roles in the growth cone. The activated MYR::UNC-40 causes inhibition of growth cone protrusion and fewer MT + ends, suggesting that the antiprotrusive roles of UNC-40 predominate in the activated MYR::UNC-40 in VD growth cones, as do the antiprotrusive roles of activated Rac GTPases MIG-2 and CED-10. In the HSN neuron, whose growth cone grows toward UNC-6/Netrin, activated MYR::UNC-40 causes excess protrusion (Norris and Lundquist 2011), indicating differences in MYR::UNC-40 activity in different growth cones.
The polarity/protrusion model of directed growth away from UNC-6/Netrin
Our results suggest that UNC-6/Netrin polarizes the growth cone via the UNC-5 receptor, resulting in asymmetric dorsal F-actin accumulation and protrusion (Figure 12). UNC-6/Netrin then regulates protrusion in response to this polarity. Through the UNC-5 receptor, UNC-6/Netrin inhibits ventral and lateral protrusion by restricting MT + end entry and via an MT-independent mechanism possibly involving actin. Through the UNC-40 receptor, UNC-6/Netrin stimulates protrusion at the dorsal leading edge downstream of the F-actin polarity established previously. By maintaining this polarity of protrusion, directed growth away from UNC-6/Netrin results. F-actin polarity might define where UNC-40-mediated protrusion can occur, and MTs might deliver proprotrusive factors into the growth cone that are activated by UNC-40 signaling (e.g., proprotrusive actin regulators and/or vesicle fusion).
Classically, the conserved ventral expression of Netrin was thought to establish a chemotactic gradient of Netrin to which growth cones were either repelled or attracted, depending upon whether or not they expressed UNC-5 (Tessier-Lavigne and Goodman 1996). Our results, and those of others (Kulkarni et al. 2013; Yang et al. 2014; Limerick et al. 2018), indicate that both UNC-40 and UNC-5 act in growth cones that both migrate toward and away from UNC-6/Netrin. In both the SOAL model of growth toward UNC-6/Netrin (Kulkarni et al. 2013; Yang et al. 2014; Limerick et al. 2018) and in our polarity/protrusion model of growth away from UNC-6/Netrin (Figure 12), UNC-5 serves to polarize and focus the site of UNC-40-mediated protrusion and to prevent protrusion from other regions of the growth cone. In other words, UNC-6/Netrin polarizes the growth cone and then regulates protrusion based upon this polarity. Thus, the difference between growth toward vs. growth away from UNC-6/Netrin depends upon where in the growth cone UNC-40-mediated protrusion is localized. UNC-40 activity is polarized toward the UNC-6/Netrin source in growth toward UNC-6/Netrin (the SOAL model), and is polarized away from the UNC-6/Netrin source in growth away from UNC-6/Netrin (the polarity/protrusion model).
Recent studies in the vertebrate spinal cord have shown that expression of Netrin-1 in the floorplate is not required for ventral commissural axon ventral guidance, (Dominici et al. 2017; Varadarajan and Butler 2017; Varadarajan et al. 2017; Yamauchi et al. 2017). Rather, expression in ventricular cells is important, possibly in a contact-mediated haptotactic event. It will be important to determine if similar polarity/protrusion events described here are occurring in the growth cones commissural axons responding to Netrin-1 from ventricular cells in the spinal cord.
Outstanding questions about the polarization/protrusion model presented here remain. For example, how does UNC-6/Netrin result in polarized protrusive activities in the growth cone? Asymmetric localization of UNC-40 and/or UNC-5 is an attractive idea, but UNC-40::GFP shows uniform association of the growth cone margin in VD growth cones and no asymmetric distribution (Norris et al. 2014). Also, once established, how is polarized protrusive activity maintained as the growth cone extends dorsally away from the UNC-6/Netrin source? Answers to these questions will be the subject of future study.
Acknowledgments
The authors thank the members of the Lundquist and Ackley laboratories for discussion, E. Struckhoff for technical assistance, and Y. Jin for the pCGY23-32 Punc-25::ebp-2::gfp plasmid. This work was supported by National Institutes of Health (NIH) grants R01NS040945, R56NS095682, and P20GM103638. A.S. was a Kansas Infrastructure Network of Biomedical Excellence Undergraduate Scholar and Star Trainee (NIH P20GM103418), and was supported by the University of Kansas Center for Undergraduate Research.
Footnotes
Communicating editor: M. Sundaram