-
PDF
- Split View
-
Views
-
Cite
Cite
Jacob S Jaszczak, Jacob B Wolpe, Anh Q Dao, Adrian Halme, Nitric Oxide Synthase Regulates Growth Coordination During Drosophila melanogaster Imaginal Disc Regeneration, Genetics, Volume 200, Issue 4, 1 August 2015, Pages 1219–1228, https://doi.org/10.1534/genetics.115.178053
- Share Icon Share
Abstract
Mechanisms that coordinate growth during development are essential for producing animals with proper organ proportion. Here we describe a pathway through which tissues communicate to coordinate growth. During Drosophila melanogaster larval development, damage to imaginal discs activates a regeneration checkpoint through expression of Dilp8. This both produces a delay in developmental timing and slows the growth of undamaged tissues, coordinating regeneration of the damaged tissue with developmental progression and overall growth. Here we demonstrate that Dilp8-dependent growth coordination between regenerating and undamaged tissues, but not developmental delay, requires the activity of nitric oxide synthase (NOS) in the prothoracic gland. NOS limits the growth of undamaged tissues by reducing ecdysone biosynthesis, a requirement for imaginal disc growth during both the regenerative checkpoint and normal development. Therefore, NOS activity in the prothoracic gland coordinates tissue growth through regulation of endocrine signals.
ALLOMETRY, broadly defined as the scaling of organ growth, can have a profound impact on the biological function in animals. For example, in the male dung beetle, Onthophagus netriventris, an inverse allometry is observed between horn and testes size, producing distinct reproductive strategies (Simmons and Emlen 2006; Emlen et al. 2012). Allometric growth regulation can also impact human health where variation from optimal relative heart size can increase susceptibility to cardiovascular disease (Hill and Olson 2008). Despite the fundamental role of growth scaling in biology, no described pathways explain how tissues coordinate growth. Our understanding of growth regulation has been focused on either tissue-autonomous mechanisms—such as how morphogens regulate the activity of cellular growth pathways—or systemic mechanisms such as how endocrine factors control growth in response to environmental change.
These tissue-autonomous and systemic pathways of growth regulation do not explain allometric growth observed during development. Transplantation experiments (Madhavan and Schneiderman 1969) and growth perturbation experiments in Drosophila and other insects (Nijhout and Emlen 1998; Simmons and Emlen 2006; Parker and Shingleton 2011) suggest that interorgan communication may be necessary for allometric growth. Based on these observations, Stern and Emlen (1999) proposed a model for growth coordination that requires communication between growing organs, either directly or indirectly through an endocrine organ. However, the mechanism of this communication pathway has been unclear.
In Drosophila larvae, the growth of the imaginal discs is tightly regulated to produce adult structures with specific size and proportion (Mirth and Shingleton 2012; Callier and Nijhout 2013). Allometry between these tissues is preserved even when developmental growth programs are altered. For example, Drosophila imaginal discs have a remarkable capacity to regenerate and restore proper size and allometry following damage (Bryant 1971; Schubiger 1971). Damage to an imaginal disc activates a regeneration checkpoint (Halme et al. 2010) that extends the larval period of development (Rahn 1972; Dewes 1975; Simpson et al. 1980; Poodry and Woods 1990; Halme et al. 2010), allowing time for regenerative tissue repair. Regeneration checkpoint activation also slows the growth rate of undamaged tissues (Madhavan and Schneiderman 1969; Stern and Emlen 1999; Martín and Morata 2006; Parker and Shingleton 2011), coordinating regeneration with the growth of undamaged imaginal discs. Both developmental delay and growth coordination depend on the expression of Drosophila insulin-like peptide 8 (Dilp8) in damaged tissues (Colombani et al. 2012; Garelli et al. 2012). Dilp8 is a secreted protein that shares structural features with the insulin/relaxin protein family. Several questions remain about how Dilp8 produces both growth regulation and developmental delay. It is possible that these two responses might be mechanistically linked; for example, growth restriction may lead to developmental delay (Poodry and Woods 1990; Stieper et al. 2008). Alternatively, these two systemic responses may reflect distinct Dilp8-dependent mechanisms. Additionally, it remains unclear whether Dilp8 functions to directly coordinate growth between tissues or whether Dilp8 mediates growth coordination indirectly through other systemic growth signals.
Nitric oxide synthase (NOS) produces nitric oxide (NO), a potent free radical that regulates many biological processes, including neuronal activity, immunity, and vascular regulation. Altering the activity of the sole NOS protein found in Drosophila produces changes in imaginal disc growth (Kuzin et al. 1996) and larval tissue growth (Cáceres et al. 2011). However, the mechanism of this regulation has remained unclear. In the experiments presented here, we outline a pathway through which tissues communicate with each other to produce allometric growth. We demonstrate that NOS activity is required for the Dilp8-dependent coordination of growth between regenerating and undamaged tissues following tissue damage and that NOS regulates growth in undamaged tissues by reducing ecdysone biosynthesis in the prothoracic gland.
Materials and Methods
Drosophila stocks
w*; P{UAS-Nos.L}2; P{UAS-Nos.L}3 was provided by Pat O’Farrell (Yakubovich et al. 2010); y,w; phm-GAL4{51A2} by Alexander Shingleton (Mirth et al. 2005); UAS-NOSmac and UAS-NOSIR-X by Henry Krause (Cáceres et al. 2011); NOS1 by James Skeath (Lacin et al. 2014); UAS-eiger and UAS-reaper and rn-Gal4, UAS-YFP by Iswar Hariharan (Smith-Bolton et al. 2009); UAS-dilp8::3xFLAG by Maria Dominguez (Garelli et al. 2012); and UAS-Avl RNAi by David Bilder (Lu and Bilder 2005). All other stocks were obtained from the Bloomington Drosophila Stock Center or the Vienna Drosophila RNAi Center. Identifying stock numbers are referenced in the text.
Drosophila culture and media
Unless otherwise specified, larvae were reared at 25° on standard cornmeal–yeast–molasses media (Bloomington Drosophila Stock Center) supplemented with live baker’s yeast granules. Developmental timing was synchronized through the collection of a 4-hr egg-laying interval on grape agar plates. Twenty hatched first instar larvae were transferred to vials containing media 24 hr after egg deposition (AED) (48 hr AED when raised at 21°). Heat shock-mediated expression was induced by 29° pretreatment and heat shock for 30 min at 37°. Nutrient restriction was initiated at 92 hr AED by transferring larvae to media containing only 1% agarose (Apex) in 1× PBS (pH 7.4, Sigma P4417) for the remainder of larval development.
Ionizing irradiation damage
Irradiation was performed as previously described (Halme et al. 2010). Briefly, staged larvae were raised in petri dishes on standard media and exposed to 25 Gy X-irradiation generated from a Faxitron RX-650 operating at 130 kV and 5.0 mA. For targeted irradiation experiments, shielded and control larvae were immobilized by being chilled in an ice bath, mounted on chilled glass coverslips, and kept on ice during the duration of the irradiation. Larvae were partially shielded from ionizing irradiation by placing a 0.5-cm2 strip of lead tape (Gamma) over the estimated anterior third of their body, covering segments T1–T3. Larvae and control larvae were returned to cornmeal–molasses food at 25° following irradiation.
DAF2-DA assay
NO production was detected by 4,5-diaminofluorescein diacetate (DAF2-DA, Sigma). Brain complexes were dissected in PBS and incubated in 10 μM DAF2-DA for 1 hr at 28°, rinsed in PBS, stained with DAPI 1:1000, rinsed in PBS, and imaged by confocal microscopy. DAF2-DA fluorescence was quantified in ImageJ by measuring the mean gray value of each prothoracic gland (PG) lobe normalized to the background fluorescence of the adjacent brain hemisphere.
Additional methods and reagents are described in Supporting Information, File S1.
Results
NOS is necessary for growth regulation during the regeneration checkpoint
During larval development, imaginal disc damage activates a regeneration checkpoint that coordinates the regeneration of damaged imaginal tissues with developmental progression. Activation of the regeneration checkpoint produces both (1) delayed larval–pupal transition (Rahn 1972; Dewes 1975; Simpson et al. 1980; Poodry and Woods 1990; Halme et al. 2010) and (2) a reduced growth rate of undamaged imaginal tissues (Stern and Emlen 1999; Martín and Morata 2006; Parker and Shingleton 2011). Developmental delay and growth regulation have been shown to be dependent on Dilp8, but it has not been determined how Dilp8 reduces growth of undamaged imaginal tissues (Colombani et al. 2012; Garelli et al. 2012). We observe that growth coordination between damaged and undamaged imaginal discs occurs when damage is induced by genetically targeted ablation of wing imaginal discs (Bx > eiger) or by exposing the posterior of the larva to X-irradiation (Figure 1, A–C, and Figure S1B for a description of the targeted irradiation technique). Consistent with earlier work (Colombani et al. 2012; Garelli et al. 2012), we find that both of these targeted damage models depend on the expression of dilp8 from damaged tissues for growth coordination and developmental delay (Figure 1, B and C; Figure S1, C and D).
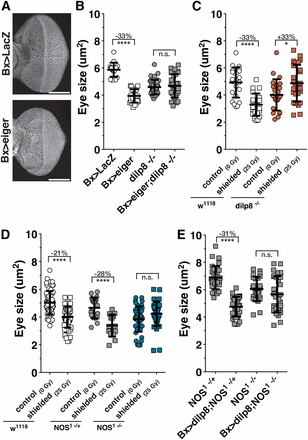
NOS is required for imaginal disc growth coordination during the regeneration checkpoint. (A) Growth reduction of undamaged eye imaginal discs in larvae with targeted tissue damage in the wings (Bx > eiger) and control larvae (Bx > GFP). Eyes were isolated at 104 hr AED and stained with rhodamine-labeled phalloidin. Bar, 100 μm. (B) Dilp8 is required for coordinating imaginal tissue growth during targeted wing damage. Eye imaginal disc size measured at 104 hr AED following targeted wing expression of eiger (Bx > eiger) or control (Bx > LacZ) in larvae wild type for dilp8 or homozygous dilp8−/−. (C) Dilp8 is required for coordinating imaginal tissue growth during irradiation damage. Measurement of undamaged eye imaginal disc size following targeted irradiation (shielded, 25 Gy) compared to unirradiated control (0 Gy) in wild type (w1118) and homozygous dilp8−/− larvae. Posterior tissues were exposed to 25 Gy ionizing irradiation at 80 hr AED while anterior tissues were shielded using lead tape (see Materials and Methods and Figure S1B for more detail). Eye imaginal disc size measured at 104 hr AED. (D) NOS is required for coordinating imaginal tissue growth during the regeneration checkpoint. Coordination of growth during targeted irradiation is lost in larvae mutant for NOS. Measurement of undamaged eye imaginal disc size following targeted irradiation compared to unirradiated control in wild type (w1118) and larva heterozygous or homozygous for NOS mutant (NOS1). Posterior tissues were exposed to irradiation at 80 hr AED, and eye imaginal disc size was measured at 104 hr AED. (E) Dilp8 growth restriction requires NOS. Eye imaginal disc growth restriction during dilp8 overexpression in the wing (Bx > dilp8) is lost in larvae mutant for NOS (NOS1 −/−). Larvae were raised at 29°, and eye imaginal disc size was measured at 100 hr AED. Statistical analysis: mean ± SD. *P < 0.05, ****P < 0.001 calculated by two-tailed Student’s t-test.
NOS regulates imaginal disc growth during Drosophila development (Kuzin et al. 1996), but the mechanism of this regulation is unknown. Therefore, we asked whether NOS is involved in Dilp8-dependent growth coordination. Using targeted irradiation, we observed that the reduced growth of shielded eye discs is rescued when larvae are homozygous for an amorphic allele of NOS [NOS1 (Lacin et al. 2014)] (Figure 1D). Overexpression of Dilp8 is sufficient to reduce imaginal disc growth and produce developmental delay (Figure S1E) (Colombani et al. 2012; Garelli et al. 2012). To determine whether Dilp8-induced growth restriction is dependent on NOS, we measured growth of eye imaginal discs in NOS mutant larvae overexpressing Dilp8 in the wing imaginal discs (Bx > dilp8;NOS1−/−). We observe that Dilp8-induced growth restriction is rescued in NOS1−/− mutant larvae (Figure 1E). Therefore NOS is required for growth coordination during the regeneration checkpoint, and Dilp8 is dependent on NOS for imaginal disc growth restriction.
Nitric oxide synthase activity in the prothoracic gland regulates imaginal disc growth
Consistent with previous observations (Kuzin et al. 1996), we find that a transient pulse of NOS expression (hs > NOS) early in the third larval instar (76 hr after egg deposition, AED) reduces imaginal disc growth. (Figure 2A and Figure S2A). However, overexpression of NOS within imaginal discs produces no observable effect on imaginal disc growth (Figure S2B), suggesting that NOS regulates growth via a nonautonomous pathway. Additionally, we observed that NOS induction following heat shock produces a developmental delay (Figure 2A and Figure S2A) without producing damage or apoptosis within the imaginal discs (Figure S2C).
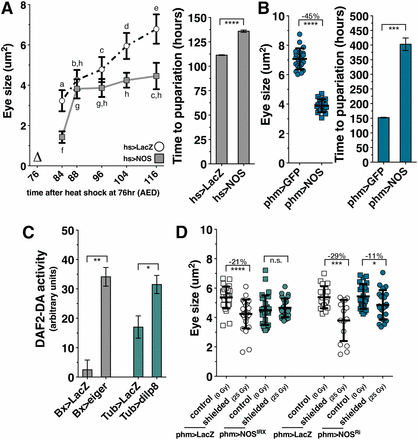
NOS is required in the PG to coordinate imaginal tissue growth during the regeneration checkpoint. (A) A systemic pulse of NOS expression early during the larval feeding period restricts imaginal disc growth throughout the rest of larval development. NOS was systemically expressed by heat shock (∆) at 76 hr AED, and eye imaginal disc size was measured in populations of larva at subsequent time points. NOS overexpression at 76 hr AED extends larval development. Measurement of pupariation timing (marked by eversion of anterior spiracles) following systemic expression of NOS (hs > NOS) is depicted on the right. (B) NOS overexpression in the PG (phm > NOS) restricts imaginal disc growth and extends larval development. phm > GFP- and phm > NOS-expressing larvae were raised at 21° (see Figure S2D). (C) Both targeted tissue damage (Bx > eiger) and systemic dilp8 expression (Tub > dilp8) increase NO production in the PG. Measurement of NO production by the fluorescent reporter DAF2-DA. Brain complexes with the PG attached were isolated and stained with DAPI and DAF2-DA at 93 hr AED. n for Bx > LacZ = 36, Bx > eiger = 23, Tub > LacZ = 20, and Tub > dilp8 = 23. (D) NOS is required in the PG for regeneration checkpoint growth coordination. Measurement of undamaged eye imaginal disc size following shielded irradiation (25 Gy) compared to unirradiated control (0 Gy) in control (phm > LacZ) or NOS-targeted RNAi expressed in the PG (phm > NOSIR-X or phm > NOSRi BL28792). Posterior tissues were exposed to 25 Gy ionizing irradiation at 80 hr AED and anterior tissues, including the eye discs, were shielded using lead tape. Eye imaginal disc size was measured at 104 hr AED. Statistical analysis: (A) Differing letters denote statistical significance calculated by one-way ANOVA with Tukey’s post-test. (B) Mean ± SD. Time in A and B is the mean of triplicate experiments ± SEM. (C) Mean of triplicate experiments. (D) Mean ± SD. *P < 0.05, **P < 0.01, ***P < 0.005, and ****P < 0.001 calculated by two-tailed Student’s t-test.
The timing of developmental transitions in Drosophila larvae is regulated by the PG through pulsed production of the steroid hormone ecdysone (Warren et al. 2006). NOS expression in the PG has been demonstrated to regulate the larval-to-pupal transition by promoting ecdysone production in postfeeding larvae (Cáceres et al. 2011). However, when Cáceres et al. constitutively overexpressed NOS in the PG at 25° throughout larval development, they observed a delayed developmental progression and decreased larval size. Therefore, we examined whether NOS regulates growth through activity in the PG during the larval growth prior to the postfeeding phase of larval development. Using the phantom-Gal4 driver, which specifically targets Gal4-mediated expression to the PG throughout larval development (Mirth et al. 2005), we observed that most phm > NOS larvae raised at 25° died prior to the third larval instar. To determine if larval lethality could be rescued by reducing the expression of NOS in the PG, we raised phm > NOS and control larvae at 21°, which reduces GAL4 activity and slows developmental time (Figure S2D). The majority of phm > NOS larvae raised at 21° progressed through the third instar to pupation (Figure S2E). We observed that in these phm > NOS larvae the growth rate of the eye imaginal tissues is reduced relative to control larvae raised at 21° (Figure 2B). The pupation of these larvae is also delayed in comparison to control larvae (Figure 2C). Therefore, NOS overexpression in the PG is sufficient to reduce the growth of imaginal discs during the third larval instar and can delay the exit from larval development.
NOS catalyzes the production of the free radical nitric oxide (NO), an important cellular signaling molecule, from l-arginine. To determine whether NOS activity is increased in the PG during the regeneration checkpoint, we used the fluorescent reporter molecule DAF2-DA to measure NO production, as can be observed in the PG of phm > NOS larvae (Figure S3). Larva with genetically targeted wing ablation (Bx > eiger) or systemic Dilp8 misexpression produce increased levels of NO signaling in the PG compared to control larvae (Figure 2C), demonstrating that the regeneration checkpoint acts through Dilp8 to increase NOS activity in the PG. To examine whether this activation of NOS in the PG is required for the regeneration checkpoint growth coordination, we expressed a NOS-targeted RNAi to disrupt NOS function in the PG using the phm-GAL4 driver [phm > NOSIR-X (Cáceres et al. 2011) or phm > NOSRi (Bloomington #28792)]. Using the targeted irradiation technique, we observed that depletion of NOS in the PG by RNAi restored eye imaginal disc growth in shielded larvae to the rate observed in unirradiated larvae (Figure 2D). Therefore, nitric oxide production is increased in the PG during the regeneration checkpoint, and NOS activation in the PG is necessary to regulate imaginal tissue growth during the regeneration checkpoint.
Growth regulation during the regeneration checkpoint is dependent on NOS. However, we observed no effect on the delay of development induced by irradiation in NOS RNAi knockdown or in the NOS mutant larvae (Figure 3, A and B). These data suggest that overexpression of NOS (Figure 2, A and B) delays development through a mechanism distinct from the regeneration checkpoint. Together, these data demonstrate that localized imaginal disc damage produces two effects: (1) growth inhibition in undamaged imaginal tissues, which is dependent on NOS function in the PG, and (2) a delay in developmental timing, which occurs through a NOS-independent pathway.
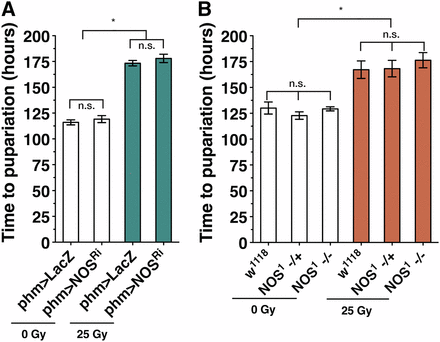
NOS is not required for regulation of developmental time during the regeneration checkpoint. Loss of NOS function either by (A) knockdown of NOS in the PG (phm > NOSRi) or (B) NOS mutant (NOS1) does not alter developmental delay. Measurement of pupariation timing for larvae with irradiation damage (25 Gy) and control larvae (0 Gy). Mean of triplicates ± SEM. *P < 0.05 calculated by one-way ANOVA with Tukey’s post-test.
Growth of imaginal tissues is selectively regulated during the regeneration checkpoint
Larval size is determined by the growth of polyploid larval tissues such as the larval epidermis, fat body, and salivary glands (Oldham et al. 2000). Unlike the diploid imaginal tissues, which will become much of the adult fly following metamorphosis, most larval tissues are histolysed during metamorphosis and do not contribute significantly to the adult. To determine whether imaginal tissues are selectively targeted for growth regulation during the regeneration checkpoint, we compared the effects of regeneration checkpoint activation on the growth of both imaginal tissues and total larval size, which correlates with the growth of the polyploid larval epidermis (Cheng et al. 2011). Consistent with our earlier observations, targeted irradiation or genetically targeted ablation of wing imaginal discs (Bx > eiger) is sufficient to activate the regeneration checkpoint and produce growth inhibition of undamaged eye imaginal discs (Figure S4, A and B). Both damage models depend on Dilp8 for growth inhibition and developmental delay (Figure 1 and Figure S1). Consistent with a role for NOS and Dilp8 in growth regulation during the regeneration checkpoint, we observe growth inhibition of undamaged eye imaginal tissues by expression of NOS in the PG (phm > NOS, Figure S4B) and dilp8 expression in the wing pouch, the region of the imaginal disc that will become the adult wing blade (rn > dilp8, Figure S4B).
In stark contrast, we found that checkpoint activation does not reduce overall larval growth (Figure S4, C and D). In our two damage models, larval growth continued at the same rate or even slightly faster than the growth observed in control larvae. Similarly, we observed a slight but not statistically significant increase in the rate of larval tissue growth in larvae with phm > NOS and wing-targeted expression of Dilp8 (rn > dilp8), as compared with control larvae (Figure S4D). Additionally, we examined other disruptions of wing imaginal disc growth and found that induction of neoplastic tumors in the wing imaginal tissues using knockdown of the Drosophila syntaxin protein Avalanche [Bx > avlRNAi (Lu and Bilder 2005)] also produces slower growth in the eye imaginal discs without altering larval tissue growth (Figure S4, B and D). This is consistent with the observed activation of Dilp8 during tumorigenesis (Colombani et al. 2012; Garelli et al. 2012). This pattern of growth regulation observed during the regeneration checkpoint—i.e., reduced growth of imaginal discs and sustained or even increased growth of larval tissues —contrasts with the growth pattern observed in larvae with reduced insulin signaling in response to nutrient restriction, where growth of both imaginal and larval tissues is reduced (Figure S4, B and D). Therefore, we sought to test a growth regulatory pathway other than insulin signaling that would explain how regenerative checkpoint activation and NOS activity could specifically reduce imaginal disc growth.
NOS in the PG inhibits ecdysone biosynthesis during the larval growth phase
The PG produces pulses of ecdysone synthesis during the larval growth phase that determine the timing of developmental transitions such as larval molts, the mid-third instar transition (Andres and Cherbas 1992), critical weight (Koyama et al. 2014), and the exit from larval development. Experiments support roles for ecdysone in both promoting (Nijhout et al. 2007; Delanoue et al. 2010; Nijhout and Grunert 2010; Parker and Shingleton 2011) and restricting (Colombani et al. 2005; Mirth et al. 2005; Delanoue et al. 2010; Nijhout and Grunert 2010; Boulan et al. 2013) growth of imaginal discs. Activation of the regeneration checkpoint slows the progression of the morphogenetic furrow in undamaged eye discs (see Figure S4A). Furrow progression is dependent on ecdysone (Brennan et al. 1998), and therefore its slowed progression is consistent with the regeneration checkpoint reducing ecdysone signaling during larval development. Since the overexpression of NOS in the PG influences both developmental timing and imaginal disc growth, we examined whether NOS activity in the PG alters ecdysone signaling during the regeneration checkpoint.
Regeneration checkpoint activation has been shown to reduce ecdysone biosynthesis (Hackney et al. 2012). To examine whether NOS activity in the PG reduces ecdysone production, we measured ecdysteroid levels using a competitive enzyme immunoassay (Porcheron et al. 1989). In larvae overexpressing NOS in the PG (phm > NOS), we observed a strong reduction in ecdysteroid levels during the mid-third instar when imaginal disc growth is rate reduced (Figure 4A). To determine whether ecdysone signaling is reduced during this period, we measured transcription of the ecdysone target gene E74B (Colombani et al. 2005; Parker and Shingleton 2011; Hackney et al. 2012). In phm > NOS-expressing larvae we observed that the expression of E74B is lower than in control larvae during the mid- and late third instar (Figure 4B), suggesting that ecdysone titers are reduced during this period. Consistent with previous studies (Hackney et al. 2012), we observed that transcription of E74B is reduced following activation of the regeneration checkpoint in Bx > eiger larvae (Figure S5A). Together, these results demonstrate that ecdysone production is reduced when NOS is active in the PG during the third instar larval growth period.
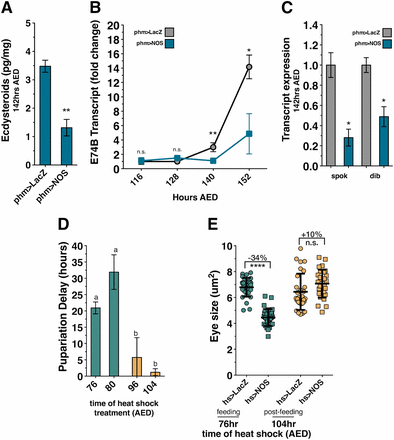
NOS overexpression during larval feeding inhibits ecdysone biosynthesis. (A) NOS activity in the PG reduces ecdysteroid production. The presence of ecdysteroids is reduced in larvae with NOS overexpression in the PG (phm > NOS) compared to control (phm > LacZ) larvae. Ecdysone levels were measured by ELISA assay for independent isolation triplicates. (B) NOS expression in the PG reduces ecdysone signaling. Transcription of E74B is reduced in larvae with NOS overexpression in the PG (phm > NOS) compared to control (phm > LacZ) larvae. Transcription levels measured by qRT-PCR in triplicate, normalized to control expression levels at 116 hr AED. (C) NOS activity in the PG reduces Halloween gene transcription. Relative expression of spok and dib in control (phm > LacZ) larvae and larvae with NOS overexpression in the PG (phm > NOS) are depicted. Transcription levels were measured by qRT-PCR in triplicate, normalized to control transcription levels. (D) Expression of NOS early during the larval feeding period (76 and 80 hr AED) substantially delays larval development, while NOS expression late during the wandering period (96 and 104 hr AED) does not delay development. NOS was systemically expressed by heat shock (hs > NOS) once at 76, 80, 96, or 104 hr AED, and time to pupariation was measured. (E) Expression of NOS early during the larval feeding period restricts imaginal disc growth, while NOS expression late during the wandering period does not inhibit growth. NOS was systemically expressed by heat shock (hs > NOS) at either 76 or 104 hr AED, and eye imaginal disc size was measured at 116 hr AED. All phm > LacZ- and phm > NOS-expressing larvae were raised at 21°. Statistical analysis: A and E, mean of triplicates ± SD calculated by two-tailed Student’s t-test. (B and C) Mean of triplicates ± SEM, calculated by paired one-tailed t-test. (D) Mean of triplicates ± SEM. *P < 0.05, **P < 0.01, ****P < 0.001.
To better understand how NOS reduces ecdysone production in larvae, we examined whether NOS regulates the expression of ecdysone biosynthetic genes. Ecdysone is synthesized in the PG from sterol precursors by the consecutive actions of the P450 enzymes collectively referred to as “the Halloween enzymes” (Gilbert and Rewitz 2009). Previous work has demonstrated that the expression of Halloween genes is reduced during activation of the regeneration checkpoint (Hackney et al. 2012). To determine whether NOS regulates ecdysone synthesis by limiting Halloween gene expression, we examined the transcription of the Halloween genes spookier (spok) (Ono et al. 2006) and disembodied (dib) during either targeted tissue damage or NOS overexpression in the PG (Chávez et al. 2000). Transcription of both spok and dib is reduced in phm > NOS larvae in comparison to control larvae (Figure 4C), consistent with the reductions observed during regeneration checkpoint activation (Figure S5A). Therefore, upon activation of the regeneration checkpoint, NOS functions in the PG to reduce ecdysone signaling through the transcriptional repression of ecdysone biosynthesis genes.
This model contrasts with a model arising from previous work demonstrating that NOS activity in the PG of postfeeding larvae inhibits the nuclear hormone receptor E75, an antagonist of ecdysone biosynthesis (Cáceres et al. 2011). To reconcile these two distinct descriptions of NOS activity in the PG, we first sought to determine whether this E75-dependent pathway of ecdysone regulation is active during the earlier growth phase of larval development. We observed that the E75B expression, which is normally upregulated during the postfeeding period of larval development, is completely suppressed in larvae with targeted wing damage (Figure S5B). Therefore, we conclude the E75-dependent pathway is not likely to be active during the growth phase of larval development and is delayed following activation of the regeneration checkpoint. Consistent with this, the ability of transient NOS misexpression (hs > NOS) to delay pupation is most robust when expressed during larval feeding (76 or 80 hr). This delay is significantly decreased when NOS was misexpressed later in the third instar as the larvae entered the postfeeding phase (96 or 104 hr) (Figure 4D). These results suggest that the ecdysone-inhibiting and ecdysone-promoting mechanisms of NOS are temporally separated during the larval growth and postfeeding phases of development.
To further test whether NOS activity is dependent on developmental stage of the larvae, we examined whether the reduction of imaginal disc growth induced by transient misexpression of NOS (hs > NOS) is dependent on the stage of development. We observed that misexpressing NOS early in the third instar during the larval feeding period (76 hr AED) produces a robust restriction of imaginal disc growth (Figure 4E, 76 hr). However, we found that misexpression of NOS late in the third instar, at the time that larvae stop feeding (104 hr AED), produces minimal effect on developmental time and imaginal disc growth (Figure 4, D and E, 104 hr). In fact, a slight increase in growth was measurable. Consistent with this, we also observed decreases in E74B and dib transcription after early NOS misexpression in contrast to an increase in spok transcription resulting from late NOS misexpression (Figure S5, C and D). These results suggest that as development progresses the regulatory effect of NOS in the PG has two distinct states. During the feeding phase of larval development, NOS inhibits ecdysone production as we describe here. Later in postfeeding larvae, as described in Cáceres et al. (2011), NOS functions to promote ecdysone signaling by inhibiting E75 activity.
Regeneration checkpoint reduces growth of undamaged tissues by limiting ecdysone signaling
We then determined whether the reduced growth of undamaged discs during regeneration checkpoint activation is the result of reduced ecdysone production. Feeding larvae food supplemented with ecdysone (0.6 mg/ml) increases ecdysone titer in larvae (Colombani et al. 2005). Using this approach, we tested whether we could bypass NOS-dependent growth inhibition by ecdysone feeding. We observed that ecdysone feeding can bypass the imaginal disc growth restriction produced by (1) imaginal tissue damage (Bx > eiger, Figure 5A), (2) regeneration checkpoint signaling (Tub > dilp8, Figure 5B), or (3) transient misexpression of NOS (hs > NOS, Figure 5C and Figure S6A) and NOS activity in the PG (phm > NOS, Figure 5D). Ecdysone feeding did not significantly alter the growth of larval tissues during damage or NOS overexpression, but strongly reduced larval tissue growth in dilp8-misexpressing larvae, as reflected in the overall larval size (Figure S6C).
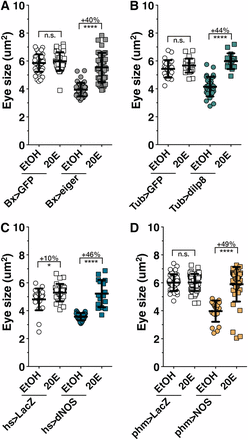
Imaginal disc growth restriction during the regeneration checkpoint is the result of reduced ecdysone signaling. Ecdysone levels are rate-limiting for imaginal disc growth during the regeneration checkpoint. 20-Hydroxyecdysone (20E) rescues growth restriction induced by (A) targeted wing damage (Bx > eiger), (B) systemic dilp8 misexpression (Tub > dilp8), (C) systemic NOS misexpression (hs > NOS), and (D) PG NOS overexpression (phm > NOS) compared to control ethanol fed only larvae (EtOH). Statistical analysis: mean ± SD. *P < 0.05 and ****P < 0.001 calculated by two-tailed Student’s t-test.
To determine whether ecdysone promotes imaginal disc growth during normal development, we reduced ecdysone levels in third instar larvae by transferring larvae to yeast–sucrose food prepared using erg6−/− mutant yeast, which lacks the necessary steroid precursors for ecdysone synthesis (Bos et al. 1976; Parkin and Burnet 1986) (see Materials and Methods). This resulted in a marked decrease in imaginal tissue growth (Figure S6D); an extended developmental time to pupation (Figure S6E); and a slight, but significant, increase in larval tissue growth (Figure S6F) as compared with control larvae. Therefore, ecdysone is required for a normal rate of imaginal disc growth during the third instar even in the absence of imaginal disc damage. Furthermore, we observed that ecdysone limitation by growth on erg6−/− food produces only a minor effect on the growth of imaginal tissues in Bx > eiger larvae (Figure S6D). This epistatic interaction supports a model in which the regeneration checkpoint and ecdysone regulate imaginal tissue growth via convergent mechanisms. Together, these results demonstrate that the regeneration checkpoint limits undamaged imaginal disc growth through NOS-dependent reduction of ecdysone synthesis.
Discussion
During Drosophila development, damage to larval imaginal discs elicits a regeneration checkpoint that has two effects: (1) it delays the exit from the larval phase in development to extend the regenerative period and (2) it coordinates regenerative growth with the growth of undamaged tissues by slowing the growth rate of distal, undamaged tissues. How regenerating tissues communicate with undamaged tissues to coordinate growth has been an open question. Damaged tissues may produce signals that directly influence the growth of undamaged tissues or may indirectly influence the growth of undamaged tissues by producing signals that alter the levels of limiting growth factors. Consistent with the latter model, we describe an indirect-communication pathway for growth coordination during the regeneration checkpoint (Figure 6).
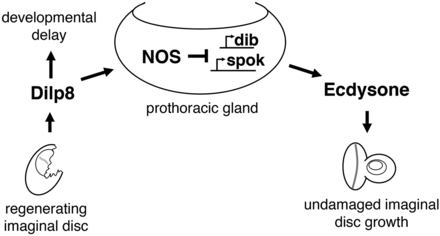
Model for allometric growth regulation during the regeneration checkpoint. Growth is coordinated between regenerating and undamaged imaginal discs through the PG. During the larval growth period, Dilp8 secreted from regenerating imaginal discs activates nitric oxide synthase in the prothoracic gland, inhibiting ecdysone biosynthesis and reducing undamaged imaginal disc growth. Dilp8-dependent developmental delay is produced through a NOS-independent mechanism.
An essential component of this growth coordination is the secreted peptide Dilp8, which is released by damaged tissues and is both necessary and sufficient to regulate the growth of distal tissues during the regeneration checkpoint (Colombani et al. 2012; Garelli et al. 2012). Dilp8 shares structural similarity to insulin-like peptides, which function to stimulate growth by activating the insulin receptor. However, in contrast to insulin-like peptides, Dilp8 acts to limit growth. A simple model explaining Dilp8 function would be that Dilp8 acts directly as an antagonist to insulin receptor activity, thus reducing growth in undamaged tissues. However, we show that the growth response to checkpoint activation of polyploid larval tissues differs from imaginal discs (Figure S4). The growth of polyploid larval tissues is very sensitive to changes in insulin signaling; therefore, these results are inconsistent with Dilp8 regulating imaginal disc growth by antagonizing systemic insulin signaling.
We show here that NOS functions in the PG to regulate the growth of imaginal discs during the developmental checkpoint. We demonstrate that growth coordination during the regeneration checkpoint increases NO production in the PG and is dependent on NOS gene function in the PG. Although constitutive expression of NOS in the PG might produce effects earlier in development that might alter our interpretations, we also demonstrate that both transient pulses of NOS during the third instar and targeted NOS activation in the PG produce the same effects: inhibition of imaginal disc growth by limiting ecdysone signaling. We show that NOS activity in the PG reduces ecdysone production through the transcriptional inhibition of the P450 enzymes disembodied and spookier, which are necessary for ecdysone biosynthesis. Although it has been known that NOS activity is capable of regulating growth of imaginal discs (Kuzin et al. 1996), the experiments that we describe here elucidate the mechanism of this growth regulation.
The activity of NOS described here contrasts with published experiments demonstrating that NO signaling inhibits E75 activity in the PG, thus promoting larval exit (Cáceres et al. 2011). However, experiments from Caceres et al. demonstrate that earlier NOS expression in the PG during larval development produces small larvae that arrest at the second larval instar stage of development. This arrest can be partially rescued either by ecdysone feeding (Cáceres et al. 2011) or by reducing the level of GAL4-UAS-driven NOS expression by raising larvae at a lower temperature (Figure S2E). Additionally, pharmacological increase of NO levels in larvae can produce larval developmental delays (Lozinsky et al. 2012, 2013). Together, these observations suggest that NOS activity earlier in larval development might inhibit rather than promote ecdysone signaling during the larval growth period. Finally, we observe that E75B is not expressed in larvae that have activated the regenerative checkpoint (Figure S5B), suggesting that the NOS-dependent pathway that has been described by Cáceres et al. (2011) is not active during the regeneration checkpoint.
We have focused on the role of NOS during the growth phase of the third larval instar (76–104 hr AED) and have found that heat-shock-mediated pulses of NOS activity during this period of development inhibit growth and ecdysone signaling, while pulses of NOS activity at the end of larval development do not inhibit growth or ecdysone signaling (Figure 4). Based on these results, we conclude that there are distinct roles for NOS in the PG during different phases in development; NOS activity during postlarval feeding promotes ecdysone synthesis through inhibition of E75, whereas NOS activity during the larval growth phase limits ecdysone synthesis and signaling by reducing the expression of ecdysone biosynthesis genes through a yet-to-be defined mechanism. Some possible mechanisms are through regulation of the growth of the PG or via activation of cGMP-dependent pathways.
Furthermore, we demonstrate that ecdysone is essential for imaginal disc growth. Most studies have supported a model in which ecdysone acts as a negative regulator of growth based on two observations: (1) the final pulse of ecdysone at the end of the third larval instar shortens developmental time and therefore reduces final organ size and (2) increased ecdysone signaling can antagonize Dilp synthesis in the fat body (Colombani et al. 2005; Mirth et al. 2005; Delanoue et al. 2010; Nijhout and Grunert 2010; Boulan et al. 2013). However, when measuring the effects of ecdysone on growth, many previous studies have focused on measuring either the growth of the larvae (which, as we observe, does not always reflect the growth of the imaginal tissues) or on measuring the final size of adults (a function of both growth rate and time). When one either examines clones expressing mutant alleles of ecdysone receptor (Delanoue et al. 2010) or measures the growth of entire imaginal discs directly following ecdysone feeding as we have done here, ecdysone signaling can be shown to promote imaginal disc growth.
During the regeneration checkpoint, both growth coordination and the delay in developmental timing are dependent on reduced ecdysone levels. Therefore, we might expect both delay and growth inhibition to be dependent on the same pathways. However, we clearly demonstrate that the genetic requirements for these two systemic responses to damage are distinct. NOS is necessary for growth regulation following tissue damage, but is not necessary for the developmental delay. While we do observe that overexpression of NOS in the PG produces developmental delay, our results suggest that this is through a mechanism different from the delays produced during the regeneration checkpoint. Therefore, Dilp8 secretion from damaged imaginal discs produces developmental delay and growth restriction through distinct mechanisms.
Finally, our observations suggest that regenerative growth, which is able to proceed despite reduced ecdysone signaling, may have different growth requirements than undamaged tissues. Understanding these differences in growth regulation could provide valuable insights into the mechanistic distinctions between regenerative and developmental growth.
Acknowledgments
This work was funded in part by a March of Dimes Basil O'Connor Starter Scholar award to AH (#5-FY12-60), and the National Institutes of Health to AH (RO1 GM099803) and JSJ (T32 GM008136). We thank D. Castle, D. DeSimone, P. Adler, J. Hirsh, and D. G. Halme for their suggestions on the manuscript.
Footnotes
Communicating editor: I. K. Hariharan
Supporting information is available online at www.genetics.org/lookup/suppl/doi:10.1534/genetics.115.178053/-/DC1.
Literature Cited
Colombani, J., Andersen D. S., and Leopold P., 2012 Secreted peptide Dilp8 coordinates Drosophila tissue growth with developmental timing. Science 336: 582–585.
Garelli, A., A. M. Gontijo, V. Miguela, E. Caparros, and M. Dominguez, 2012 Imaginal discs secrete insulin-like peptide 8 to mediate plasticity of growth and maturation. Science 336: 579–582.