-
PDF
- Split View
-
Views
-
Cite
Cite
Shaonil Binti, Philip T Edeen, David S Fay, Loss of the Na+/K+ cation pump CATP-1 suppresses nekl-associated molting defects, G3 Genes|Genomes|Genetics, Volume 14, Issue 12, December 2024, jkae244, https://doi.org/10.1093/g3journal/jkae244
- Share Icon Share
Abstract
The conserved Caenorhabditis elegans protein kinases NEKL-2 and NEKL-3 regulate membrane trafficking and are required for larval molting. Through a forward genetic screen, we identified a mutation in catp-1 as a suppressor of molting defects in synthetically lethal nekl-2; nekl-3 double mutants. catp-1 encodes a membrane-associated P4-type ATPase involved in Na+–K+ exchange. A previous study found that wild-type worms exposed to the nicotinic agonist dimethylphenylpiperazinium (DMPP) exhibited larval arrest and molting-associated defects, which were suppressed by inhibition of catp-1. By testing spectrum catp-1 alleles, we found that resistance to DMPP toxicity and the suppression of nekl defects did not strongly correlate, suggesting key differences in the mechanism of catp-1-mediated suppression. Through whole-genome sequencing of additional nekl-2; nekl-3 suppressor strains, we identified 2 additional coding-altering mutations in catp-1. However, neither mutation, when introduced into nekl-2; nekl-3 mutants using CRISPR, was sufficient to elicit robust suppression of molting defects, suggesting the involvement of other loci. Endogenously tagged CATP-1 was primarily expressed in epidermal cells within punctate structures located near the apical plasma membrane, consistent with a role in regulating cellular processes within the epidermis. Based on previous studies, we tested the hypothesis that catp-1 inhibition induces entry into the predauer L2d stage, potentially accounting for the ability of catp-1 mutants to suppress nekl molting defects. However, we found no evidence that loss of catp-1 leads to entry into L2d. As such, loss of catp-1 may suppress nekl-associated and DMPP-induced defects by altering electrochemical gradients within membrane-bound compartments.
Introduction
Ecdysozoa, animals that undergo molting, encompass the major phyla Nematoda (roundworms) and Arthropoda (insects, chelicerates, crustaceans, and myriapods), as well as several smaller phyla including Tardigrada (tardigrades) and Nematomorpha (parasitic horsehair worms) (Aguinaldo et al. 1997; Ewer 2005; Telford et al. 2008). Common to ecdysozoans is a multilayered exoskeleton, the cuticle, which is an apical extracellular matrix secreted primarily by the epidermis (Sundaram and Pujol 2024). The material composition and mechanical properties of the cuticle can vary widely among different species and life stages. Whereas the cuticle is essential for movement, protection, and other basic life functions, it can pose limitations on developmental processes including animal growth. To accommodate growth and morphological changes, ecdysozoans undergo cycles of molting (ecdysis), whereby a new cuticle is synthesized underneath the old cuticle, which is shed. In the case of the nematode Caenorhabditis elegans, the shedding process occurs at the termination of each larval stage (L1, L2, L3, and L4), with the new cuticle allowing for body size expansion when resources are replete, or long-term survival (as dauer larvae; L3d) when conditions are deemed adverse (Singh and Sulston 1978; Fielenbach and Antebi 2008; Lazetic and Fay 2017b).
Progression through molting requires the coordination of several cellular processes including the ordered transcription of hundreds of molting-associated genes; the secretion and construction of a new cuticle; and the detachment, degradation, and partial recycling of the old cuticle and precuticle structures (Johnstone and Barry 1996; Page and Johnstone 2007; Lazetic and Fay 2017b; Miao et al. 2020; Tsiairis and Grosshans 2021; Sundaram and Pujol 2024). Correspondingly, mutations that disrupt these processes within the epidermal cells that underlie and secrete the cuticle can lead to defective molting and larval arrest. These include mutations affecting several steroid hormone receptors required for the cyclic transcription of molting genes, mutations in individual cuticle components and apical extracellular matrix regulators, and mutations that affect membrane trafficking including endocytosis and exocytosis (Asahina et al. 2000; Gissendanner and Sluder 2000; Kostrouchova et al. 2001; Frand et al. 2005; Monsalve and Frand 2012; Lazetic and Fay 2017b; Johnson et al. 2023). In addition, loss of individual plasma membrane proteins can lead to molting arrest. One example is LRP-1/megalin, an epidermal apolipoprotein receptor required for the internalization of environmental sterols via clathrin-mediated endocytosis (Yochem et al. 1999; Kang et al. 2013). Because cholesterol uptake is essential for the synthesis of steroid hormones in C. elegans, a failure to internalize LRP-1 may preclude the normal transcriptional activation of molting genes (Merris et al. 2003; Entchev and Kurzchalia 2005; Martin et al. 2010; Lazetic and Fay 2017b; Joseph et al. 2020; Binti et al. 2022).
Through genetic screens, we previously identified 2 C. elegans NimA-related kinases, NEKL-2 and NEKL-3, as essential for the completion of molting (Yochem et al. 2015). Specifically, null alleles of nekl-2 or nekl-3 lead to penetrant molting defects and larval arrest at the L1/L2 transition, whereas partial reduction-of-function alleles cause larvae to arrest at L2/L3. NEKL-2 is most similar to human NEK8 and NEK9, whereas NEKL-3 is orthologous to NEK6 and NEK7. Consistent with these findings, our genetic screens identified conserved ankyrin repeat partners of NEKL-2 (MLT-2/ANKS6 and MLT-4/INVS) and NEKL-3 (MLT-3/ANKS3) as required for molting, which we showed are necessary for proper NEKL subcellular localization (Lazetic and Fay 2017a). Further studies have indicated roles for NEKL-2 and NEKL-3 at multiple steps of membrane trafficking, including clathrin-mediated endocytosis and endosomal transport, and have implicated human NEK6 and NEK7 in analogous trafficking functions in tissue culture cells (Joseph et al. 2020, 2023).
In previous work, we described a genetic screen to identify suppressors of molting defects in nekl mutants (Joseph et al. 2018). Specifically, we combined weak aphenotypic reduction-of-function alleles of nekl-2 and nekl-3 to create synthetically lethal nekl-2(fd81); nekl-3(gk894345) double mutants, which were used to identify suppressors of nekl synthetic lethality. Our screen led to the identification of mutations affecting several core components of membrane trafficking as well as ECM modifiers and cell signaling components (Joseph et al. 2018, 2022; Lazetic et al. 2018). In addition, our studies demonstrated that mutations and environmental conditions that promote induction of the dauer pathway can suppress a subset of nekl alleles that arrest at the L2/L3 transition (Binti et al. 2022). Namely, entry into the uncommitted predauer state, L2d, can be sufficient to suppress molting defects and to partially restore the expression of molting-associated genes.
Here, we report a novel nekl suppressor mutation affecting CATP-1, a P4-type Na+–K+ pump. CATP-1 functions to maintain an electrochemical gradient across membranes by exporting 3 Na+ ions and importing 2 K+ ions during each ATP-driven catalytic cycle (Kaplan 2002; Morth et al. 2011; Clausen et al. 2017). Loss of catp-1 suppresses larval arrest caused by exposure of worms to dimethylphenylpiperazinium (DMPP) (Ruaud and Bessereau 2007), a nicotinic agonist that uncouples the coordinated timing of cell divisions with molting cycles (Ruaud and Bessereau 2006). This study also provided evidence that CATP-1 is expressed and functions in the epidermis, consistent with a possible role in molting. In addition, evidence suggested that CATP-1 may be an effector of the dauer pathway, potentially linking to our finding that dauer induction can partially bypass the requirement for NEKL kinases (Binti et al. 2022). More recently, CATP-1 was shown to function in glial cells that associate with mechanosensory neurons involved in touch sensitivity (Johnson et al. 2020). Our current studies demonstrate that loss of CATP-1 ion pump activity can suppress molting defects in nekl-2(fd81); nekl-3(gk894345) mutants. In contrast to expectations, however, our data do not support the model that catp-1-mediated suppression occurs through induction of the dauer pathway, raising the possibility that changes in ion balance may impact molting through other mechanisms including possible effects on membrane trafficking.
Materials and methods
Strains, maintenance, mutagenesis
C. elegans strains were cultured on nematode growth medium (NGM) spotted with E. coliOP50 and were maintained at 22°C (unless otherwise stated) according to standard protocols (Stiernagle 2006). All strains used in this study are listed in Supplementary File 1. Mutagenesis was carried out using ethyl methanesulfonate (fd168, fd170) or N-ethyl-N-nitrosourea (ENU; fd274) as previously described (Kutscher and Shaham 2014; Joseph et al. 2018).
Genome sequencing
Whole-genome sequencing (WGS) of strains WY1286 (5 × backcross), WY1744 (3 × backcross), and WY1768 (1 × backcross) was carried out as described (Joseph et al. 2018). After sequencing of WY1286, the sibling subtraction method (Joseph et al. 2018) was carried out to reduce the number of candidate causal mutations.
CRISPR/Cas9 genome editing
Standard methods were used to design and generate desired genomic changes (Arribere et al. 2014; Kim et al. 2014; Paix et al. 2014, 2015; Fay et al. 2021; Ghanta et al. 2021). Oligos including sgRNAs, repair templates, and amplification primers used in this study are listed in Supplementary File 2. CATP-1–fluorescent protein fusion reporters were generated using methods from Dickinson et al. (2015) and Dickinson and Goldstein (2016). All CRISPR-generated mutations were also confirmed by sequencing.
catp-1(ok2585) haploinsufficiency assay
Tests for dominance/haploinsufficiency were carried out as previously described (Joseph et al. 2018). Briefly, nekl-2(fd81); nekl-3(gk894345); fdEx286 males were crossed to nekl-2(fd81) catp-1(ok2585); nekl-3(gk894345); fdEx286 hermaphrodites and cross-progeny males were scored for the presence of the nekl-3+GFP+ rescuing extrachromosomal array (fdEx286). Haploinsufficiency would be expected to result in suppressed nekl-2(fd81) catp-1(ok2585)/+; nekl-3(gk894345) adult males that do not require the array. Of the 101 adult males cross-progeny scored, 101 contained fdEx286, strongly arguing against suppression by ok2585 haploinsufficiency.
DMPP treatment and arrest assay
A 75-mM stock solution of DMPP was prepared by adding 0.239 gof DMPP to 10 ml of (reverse-osmosis) water and heating to 50°C to solubilize. DMPP plates were prepared by adding 2 ml of DMPP to 198 ml of autoclaved NGM solution for a final DMPP concentration of 0.75 mM. 5 ml was poured into individual 35-mm plates, allowed to solidify, and spotted with 250 µl of an overnight OP50 culture. Ten young adult worms were placed on each plate (2 plates per strain) and allowed to lay eggs for 8 h. Counts were made of viable/arrested worms after ∼3 days.
Image acquisition and analysis
Confocal fluorescence images were acquired using an Olympus IX83 inverted microscope with a Yokogawa spinning-disc confocal head (CSU-W1). z-Stack images with a step size of 0.2 µm were acquired using a 100×, 1.35 N.A. silicone oil objective. cellSense 3.1 software (Olympus Corporation) was used for image acquisition. DIC images and fluorescence images were obtained using a Nikon Eclipse epifluorescence microscope using 10×, 0.25 N.A. and 40×, 0.75 N.A. objectives. Image acquisition was controlled by Openlab 5.0.2 software (Agilent Inc.). Before they were imaged, animals were mounted on 3% agarose pads and anesthetized using 1 mM of levamisole in M9 buffer. Image processing and analysis were carried out using FIJI software (Schindelin et al. 2012).
Synchronization and analysis of molting
Assays for molting arrest and genetic suppression were carried out as described (Lazetic and Fay 2017a; Joseph et al. 2018). To generate synchronized populations of L1 larvae, gravid adults were subjected to treatment with bleach, after which eggs were washed at least 5 times and allowed to hatch in M9 buffer overnight at room temperature with gentle rotation (Porta-de-la-Riva et al. 2012). Hatched larvae were transferred to NGM/OP50 plates, and pharyngeal pumping or Pmlt-10::GFP–PEST (Frand et al. 2005) expression was recorded every hour at 20°C using an Olympus MVX10 MacroView microscope equipped with a 2 × objective. Molting was defined as when ≤50% animals (n = 10) were pumping or when ≥50% expressed Pmlt-10::GFP–PEST.
Statistical analysis and data availability
All statistical tests were performed using GraphPad Prism 10 following established protocols (Fay and Gerow 2013).
Results
Loss of the cation exchanger CATP-1 suppresses molting defects in nekl-2; nekl-3 mutants
In a genetic screen for suppressors of nekl-2(fd81); nekl-3(gk894345) synthetic lethality, we isolated strain WY1286 [nekl-2(fd81) fd170; nekl-3(gk894345)] (Joseph et al. 2018). Whereas 2% of nekl-2(fd81); nekl-3(gk894345) (hereafter nekl-2; nekl-3) mutants developed into viable adults because of molting defects, > 50% of nekl-2fd170; nekl-3 triple mutants progressed to adulthood within ∼3 or 4 days (Fig. 1a, b, and f). Backcrossing of WY1286 further suggested that genetic suppression was caused by a single-locus and autosomal recessive mutation. Consistent with this, WGS, in conjunction with the sibling subtraction method (Joseph et al. 2018), indicated that the suppressor mutation was on chromosome I and identified 3 point mutations predicted to alter protein coding sequences within the distal right arm of chromosome I. These included a G > A transition resulting in a premature Q905Stop in CATP-1 (CAG > TAG) (Fig. 1d; Supplementary Fig. 1), a C > T transition causing an A341T substitution in F22G12.4 (GCA > ACA), and a G > A transition leading to a G253E substitution in TAF-1 (GGA > GAA). Introduction of an equivalent catp-1 Q905Stop mutation into nekl-2; nekl-3 mutants using CRISPR/Cas9 methods (fd304) (Farboud and Meyer 2015; Farboud et al. 2019; Fay et al. 2021) led to adult viability of 58%, similar to that observed for nekl-2catp-1(fd170);nekl-3 animals (Fig. 1d–f). These findings indicate that catp-1(fd170) is the causative suppressor mutation in strain WY1286. We note that catp-1(RNAi) failed to suppress molting defects in nekl-2; nekl-3 mutants, possibly due to insufficient knockdown of CATP-1 by this method (also see below). In addition, we did not observe obvious morphological defects in catp-1 single mutants, although catp-1 strains took slightly longer to reach adulthood as compared to wild type (see below).

Mutations in catp-1 lead to suppression of nekl molting and DMPP-induced arrest. DIC and GFP image overlays of a) nekl-2(fd81); nekl-3(gk894345) and b) nekl-2(fd81); catp-1(fd170); nekl-3(gk894345) strains. Whereas the GFP-positive adult in a carries the fdEx286 extrachromosomal array (Ex+) containing rescuing wild-type nekl-3 genomic sequences and the sur-5::GFP reporter, the nonrescued GFP-negative sibling larva (Ex−) is terminally arrested at the L2/L3 molt. In contrast, ∼50% of nekl-2(fd81) catp-1(fd170); nekl-3(gk894345) worms (b) do not exhibit molting defects and reach adulthood. c) Images of molting-defective wild-type (N2) worms on DMPP plates. Arrowhead in the left panel indicates cuticle covering the buccal cavity. Small arrows in the right panel indicate cuticular material surrounding the tail region; large arrow, constriction of the midbody, which is observed in other molting-defective larvae. d) Schematic of the catp-1 genomic locus showing the locations of the relevant mutants generated for this study. Alternatively spliced forms of catp-1a and catp-1b, which differ at C-terminal exons 15 and 16 only, are indicated. e) Protein diagram of CATP-1a indicating the predicted locations of TM domains; intracellular and extracellular regions; and protein domains including actuator (A1, A2), phosphorylation (P1, P2), and ATP-binding (N) domains. The locations of several key amino acid variants are also indicated. f) Percentage of viable adults for the indicated genotypes, which include nekl-2(fd81); nekl-3(gk894345) mutations in addition to the indicated mutations in catp-1; WT indicates control strain containing a wild-type catp-1 allele. g) Percentage of viable adults following DMPP treatment for the indicated genotypes. Error bars in e and f indicate 95% confidence intervals; P-values were calculated using Fisher’s exact text; ****P < 0.0001.
catp-1 is predicted to encode a P4-type ATPase alpha subunit, which in conjunction with beta and gamma subunits forms a membrane-associated cation pump (Ruaud and Bessereau 2007). In C. elegans, CATP-1 is most similar to CATP-2 and CATP-3—both are 41% identical and 61% similar to CATP-1—and CATP-1 is 30% identical and 49% similar to CATP-4. With respect to human family members, CATP-1 is most similar to the alpha type IIC subfamily members ATP1A2 and ATP1A3, which function as ATP-dependent Na+/K+ exchangers (Kuhlbrandt 2004; Ruaud and Bessereau 2007; Farley 2012). Acting at the plasma membrane, members of this subfamily export 3 Na+ ions and internalize 2 K+ ions during each catalytic cycle, thereby producing an inside–outside electrochemical gradient. The predicted domain structure of CATP-1a includes 10 transmembrane (TM) domains, along with bipartite intracellular actuator (A1/A2) and phosphorylation (P1/P2) domains and a contiguous intracellular ATP-binding (N) domain (Fig. 1e; Supplementary Fig. 1). A second predicted isoform, CATP-1b, has an altered C terminus and contains sequences through the first 8 TM domains (Fig. 1d; Supplementary Fig. 1). The Q905Stop mutations [(catp-1(fd170) and catp-1(fd304)] are predicted to remove the C-terminal 216 and 140 amino acids of CATP-1a and CATP-1b isoforms, respectively (Fig. 1d; Supplementary Fig. 1).
To determine whether elimination of CATP-1 cation pump activity is relevant to its role in the context of nekl suppression, we used CRISPR/Cas9 to modify a highly conserved aspartic acid residue (D409) within the conserved D–K–S/T–G–T sequence of the P1 domain (Fig. 1d; Supplementary Fig. 1) (Ohtsubo et al. 1990; Ruaud and Bessereau 2007). During the cation exchange process, this aspartic acid is transiently phosphorylated, leading to the formation of a phosphoenzyme intermediate and a change in conformational state (Post and Kume 1973; Morth et al. 2011; Clausen et al. 2017). Notably, CATP-1 D409E robustly suppressed nekl-2; nekl-3 synthetic lethality (∼80% viable; Fig. 1f), indicating that reduction or loss of the CATP-1 ion pump activity is likely critical to its mechanism of nekl suppression. The greater suppression level observed for CATP-1(D409E) relative to that induced by the Q905Stop mutation could be due to partial activity retained by of the truncated protein produced by the CATP-1(Q905Stop) mutation. Taken together, our results indicate that reduction or loss of CATP-1 cation exchange function leads to the suppression of nekl-2; nekl-3 molting defects.
We next tested 2 previously isolated alleles of catp-1 to determine whether they could suppress nekl-2; nekl-3 lethality. catp-1(kr17) was originally identified as a suppressor of DMPP toxicity anWBVar02159856d contains a ∼1.5-kb Mos1 transposon insertion in the 15th exon of catp-1 (Fig. 1d). The insertion leads to a stop codon after I978, within an extracellular loop following the 7th TM domain of CATP-1 (Fig. 1e; Supplementary Fig. 1) (Ruaud and Bessereau 2007). We also tested a putative null deletion allele of catp-1(ok2585), which contains a 2,132-bp deletion spanning intron 6 to exon 10 removing a majority of the catalytic domain (V296–Q717; Fig. 1d and e; Supplementary Fig. 1) (C. elegans Deletion Mutant Consortium 2012). Somewhat surprisingly, neither kr17 nor ok2585 conferred suppression when introduced into the nekl-2; nekl-3 background, indicating that suppression is dependent on the specific properties of individual catp-1 alleles (Fig. 1f). Additionally, catp-1(ok2585) failed to suppress the single-mutant hypomorphic alleles nekl-3(sv3) and mlt-4(sv9) (Supplementary Fig. 2a). As a further test, we generated a CRISRP-based deletion allele (fd309) that removes portions of intron 3 and exon 4 (Fig. 1d and e; Supplementary Fig. 1). This deletion would be predicted to result in exon skipping and the termination of CATP-1 following position K99. Although catp-1(fd309) conferred weak suppression of nekl-2; nekl-3 mutants, the lack of robust suppression observed for all 3 catp-1 alleles suggests that strong reduction-of-function mutations, including nulls, may be relatively ineffective at suppressing nekl molting defects (Fig. 1f). One possible explanation for this observation is that strong loss of CATP-1 function may lead to the compensatory upregulation of catp-2, as has been reported for catp-1(kr17) (Johnson et al. 2020). At the same time, we failed to detect genetic suppression in nekl-2catp-1(ok2585)/+; nekl-3 worms, in which catp-1(ok2585) was heterozygous, suggesting that a ∼50% reduction in CATP-1 activity (haploinsufficiency) is not sufficient for suppressing nekl-2; nekl-3 molting defects (see Materials and methods).
catp-1 mutations identified in 2 other sequenced strains are insufficient for suppression
WGS of 2 other suppressor strains, WY1768 (nekl-2; fd168; nekl-3) and WY1744 (nekl-2fd274; nekl-3), identified 2 additional mutations predicted to alter CATP-1. Whereas strain WY1744 exhibited 34% adult viability, strain WY1768 was ∼85% viable, placing it among the strongest suppressors identified by our screen (Fig. 1c and d; Supplementary Fig. 2b) (Joseph et al. 2018). Both strains were sequenced after minimal backcrossing and without the sibling subtraction method. Whereas WGS of WY1744 identified 9 coding mutations, 7 of which were on chromosome I, sequencing of WY1768 identified 14 coding mutations, of which only 3 were on chromosome I (Supplementary Fig. 2c and d).
In the case of strain WY1744, the mutation in catp-1 led to an R668C substitution in a residue directly adjacent to a highly conserved arginine required for ATPase activity (R669; Fig. 1c and d; Supplementary Fig. 1) (Jacobsen et al. 2002; Ruaud and Bessereau 2007; Morth et al. 2011; Clausen et al. 2017). Nevertheless, BLAST searches indicate that R668 is less highly conserved than R669 within nematodes; the equivalent position in CATP-1 in Caenorhabditis bovis and Wuchereria bancrofti is a cysteine and a lysine, respectively. The mutation in strain WY1768 alters the conserved 5′ splice site of catp-1a intron 15 (GT > AT; Fig. 1d). Failure to incorporate exon 16 would remove the 62 C-terminal amino acids of CATP-1a, including portions of the ninth TM domain, the 10th TM domain, and the C-terminal 21 amino acids (Fig. 1d; Supplementary Fig. 1). In contrast, the shorter CATP-1b isoform would not be expected to be altered by the splice-site mutation (Fig. 1d).
CRISPR methods were used to introduce analogous R668C (fd407) and catp-1a intron-15 splice-site (fd429) mutations into the wild-type catp-1 locus in nekl-2; nekl-3 mutants (Fig. 1d and e). Surprisingly, neither mutation conferred robust suppression, although fd407 gave rise to ∼6% adult viability, which was slightly above background (P < 0.001; Supplementary Fig. 2b). These results demonstrate that neither mutation in catp-1 is sufficient to suppress molting defects in nekl-2; nekl-3 mutants and implicate other loci as at least partially required for the observed genetic suppression in these strains. To determine whether the detected mutations in catp-1 might partially contribute to the suppression observed in WY1744 and WY1768 strains, we attempted to revert the catp-1 mutations in these backgrounds using CRISPR methods but were unsuccessful for technical reasons. We also attempted transgenic rescue experiments using catp-1a cDNA under the control of a hypodermal-specific promoter (Y37A1B.5; Phyp7::CATP-1), including a construct that encodes an in-frame N-terminal GFP (Phyp7::GFP::CATP-1). Unfortunately, both catp-1 expression constructs appeared to be highly toxic, even when diluted with co-injection markers. As such, we were unable to determine the extent to which these mutations in catp-1 might contribute to the suppression observed these strains. Nevertheless, our findings highlight the importance of follow-up tests to determine the functional role of mutations discovered by WGS, including mutations in genes that have been shown to be causative based on other alleles.
Suppression of DMPP toxicity by catp-1 mutations
catp-1 was originally identified as a suppressor of larval lethality caused by exposure to the nicotinic agonist DMPP. We were therefore curious to determine whether the catp-1 alleles generated by our study would also suppress DMPP-induced lethality. To test this, we generated strains containing catp-1(fd304) [Q905Stop], catp-1(fd309) [K99FS], catp-1(fd287) [D409E], and catp-1(fd429) [GT > AT splice site] in wild-type backgrounds and assayed DMPP sensitivity along with catp-1(kr17), catp-1(ok2585), and wild type. In wild-type worms, DMPP treatment resulted in a penetrant larval growth arrest and/or growth retardation, with many worms exhibiting molting defects (Fig. 1c). As expected, catp-1(kr17) exhibited robust suppression of DMPP lethality, as previously reported (Fig. 1g; Supplementary Fig. 2e) (Ruaud and Bessereau 2007). Notably, the putative null deletion allele, catp-1(ok2585), conferred the highest level of viability on DMPP, despite failing to suppress nekl-associated molting defects (Fig. 1g; Supplementary Fig. 2e). Moreover, the splice-site mutation, catp-1(GT > AT) conferred moderate resistance to DMPP, indicating that loss of catp-1a coding sequences after exon 15 does impact the function of the catp-1 locus even though this allele was insufficient at suppressing nekl molting defects (Fig. 1f and g; Supplementary Fig. 2e). Likewise, strong suppression was observed for several other alleles including catp-1(Q905Stop) and the N-terminal deletion, catp-1(fd309) (Fig. 1g). Curiously, although the cation pump impaired mutant, catp-1(D409E), was strongest at suppressing nekl defects, it appeared to be the weakest of the tested DMPP suppressors (Fig. 1g), consistent with previous findings that CATP-1 may have pump-independent functions (Ruaud and Bessereau 2007). Overall, our results suggest key differences in the mechanisms by which reduction of CATP-1 function leads to the suppression of nekl-associated molting defects vs DMPP resistance.
CATP-1 is expressed in apical puncta in the epidermis
CATP-1 was previously shown to be expressed and to function in epidermal cells including the hypodermal syncytia in the head (hyp1 to hyp6), midbody (hyp7), and tail (hyp8 to hyp11) (Ruaud and Bessereau 2007). hyp7 is also the tissue focus for NEKL and MLT activities and is the major contributor to the larval and adult cuticles (Yochem et al. 2015; Lazetic and Fay 2017a, 2017b). CATP-1 was also reported to function in glial cells that surround touch-sensory neurons in the head (Johnson et al. 2020). In addition, modENCODE RNA sequencing data available on WormBase indicate that CATP-1 is expressed throughout development, including in embryos and all 4 larval stages, with highest levels corresponding to dauer larvae (Li et al. 2014; Harris et al. 2020; Sternberg et al. 2024).
To further examine the tissue expression and subcellular localization of CATP-1, we obtained endogenous CRISPR-tagged variants in which the CATP-1a isoform was tagged at its C terminus with either GFP or mScarlet. Consistent with previous studies, we observed clear expression of CATP-1::GFP and CATP-1::mScarlet in the hyp7 midbody, as well as in head and tail epidermal cells of young adults (Fig. 2a–g); expression at earlier larval stages was more difficult to analyze because of competing autofluorescence coming from the intestine. With respect to subcellular localization, we observed faint CATP-1::GFP in punctate and reticular structures in the apical region of hyp7 underlying the cuticle, which may correspond to membrane or juxtamembrane accumulations including clathrin-coated pits and/or recycling endosomes (Fig. 2a–c). Likewise, faint CATP-1::mScarlet fluorescence was observed in apical epidermal puncta but was also detected at higher levels in more medial compartments, which may correspond to late endosomes and lysosomes (Fig. 2d and e). This difference in GFP and mScarlet localization is likely explained by the observation that red fluorescent proteins can be cleaved from their translational fusion partners leading to their accumulation in acidic compartments of the endo-lysosome system in worms (Clancy et al. 2023). In addition, CATP-1::mScarlet was observed in punctate structures within the region of the pharynx (Fig. 2f and g). In contrast, both CATP-1::GFP and CATP-1::mScarlet were largely absent from epidermal seam cells (Fig. 2a, b, d, and e). The absence of apparent CATP-1 expression in nervous system–associated structures, such as head glia, may reflect sensitivity limitations of our reporters. Collectively, our data are consistent with CATP-1 playing a role in the epidermis during the molting process.
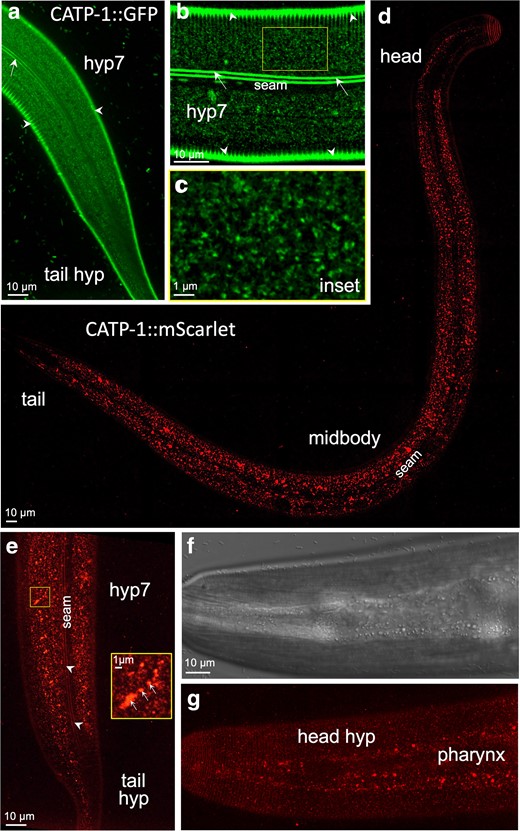
CATP-1 is expressed predominantly in punctate structures within the epidermis. Fluorescence (a–e, g) and DIC (f) images of worms expressing endogenously tagged CATP-1::GFP (a–c) and CATP-1::mScarlet (d–g), in which sequences encoding fluorescent proteins were integrated at the C terminus of the catp-1a isoform. All panels show young adults. a) Apical regions corresponding to the midbody (hyp7 syncytium) and tail (hyp8–11) are indicated. b) Midbody apical section of hyp7 and c) enlarged inset showing punctate and reticular structures. In a)–c), autofluorescence of the dorso-ventral cuticle (white arrowheads) and lateral cuticular alae (white arrows) is indicated, along with the location of the seam cell (b), in which CATP-1::GFP was not detected. d) Whole-body apical image of a young adult expressing CATP-1::mScarlet with relevant regions indicated. e) CATP-1::mScarlet expression in the apical midbody and tail regions along with enlarged inset. Note the presence of fluorescence signals in variably sized punctate structures including accumulation of mScarlet in larger structures that likely correspond to late endosomes and lysosomes (white arrows in inset). White arrowheads in e indicate autofluorescence of cuticular alae. f) DIC and g) corresponding fluorescence images of CATP-1::mScarlet in the apical head region showing expression in both the anterior hypodermis (hyp1–6) and pharynx, in which larger puncta accumulate.
We note that tagging the C terminus of CATP-1b did appear to reduce its activity based on the DMPP resistance assay. Namely, both catp-1::GFP and catp-1::mScarlet alleles conferred resistance to DMPP-induced larval arrest, although the degree of suppression was less than that observed for the putative null allele, catp-1(ok2585) (Supplementary Fig. 1e). In contrast, catp-1::GFP failed to suppress nekl-2: nekl-3 molting defects, indicating that the CATP-1::GFP fusion protein retains partial activity (Fig. 1f).
CATP-1 loss does not appear to trigger entry into L2D
Suppression of DMPP toxicity was previously observed under environmental conditions that induce the formation of dauers and in genetic mutants that cause constitutive dauer entry (Ruaud and Bessereau 2006; Ruaud et al. 2011). Data from these studies further suggested that DMPP resistance may correlate with entry into L2d, a predauer stage from which worms can either transition into dauers (L3d) or resume continuous development by entering L3. Notably, the duration of L2d is typically ∼1.2–2-fold longer than that of L2, depending on the strength and nature of the inducing signal, and suppression of DMPP toxicity by daf-2 mutants is positively correlated with the extent of L2 elongation (Golden and Riddle 1984; Ruaud et al. 2011; Karp 2018; Binti et al. 2022). Consistent with this, catp-1(kr17) was reported to extend the length of L2 by ∼1.6-fold but to have no effect on the duration of L1 or other larval stages (Ruaud and Bessereau 2007).
Recently, we showed that induction of L2d is also sufficient to suppress molting defects in ∼50% of nekl-2; nekl-3 mutants (Binti et al. 2022). We thus hypothesized that loss of catp-1 may suppress nekl-2; nekl-3 molting defects by inducing L2d. As a first test, we introduced a dauer-defective allele of daf-5 (e1386) (Riddle et al. 1981) into nekl-2catp-1; nekl-3 mutants to inhibit entry into L2d. Notably, we had previously shown that daf-5(e1386) significantly reduces the ability of dauer-inducing conditions to suppress nekl-2; nekl-3 molting defects (Binti et al. 2022). However, we observed no difference in the level of suppression in nekl-2catp-1; daf-5; nekl-3 mutants vs nekl-2catp-1; nekl-3 mutants (Fig. 1e), suggesting that loss of catp-1 does not suppress nekl molting defects through an L2d mechanism or that loss of catp-1 induces L2d via a mechanism that is independent of DAF-5 function.
To further test for the induction of L2d in catp-1 mutants, we examined the timing of larval molts in wild type and catp-1 mutants using 2 methods. In one assay, we followed molts by scoring pharyngeal pumping in a synchronized population of worms released from early L1-larval arrest; loss of pumping occurs when animals enter the lethargus phase of the molting cycle and is commonly used to measure the duration of larval stages (Singh and Sulston 1978; Lazetic and Fay 2017b). For pumping studies, we used the previously characterized catp-1(kr17) allele as well as the putative null allele, catp-1(ok2585), which we found strongly suppressed DMPP toxicity (Fig. 1c and d; Supplementary Fig. 2e) (Ruaud and Bessereau 2007). Although we observed a slight lengthening of both L1 and L2 by ∼1.1- to 1.2-fold in both catp-1 alleles, no strong or specific effect was observed at the L2 stage (Fig. 3a and c). As a further assessment, we timed molts using the Pmlt-10::GFP–PEST marker, which peaks in expression for several hours during each molting cycle (Frand et al. 2005). Based on this marker, catp-1(ok2585) mutants displayed a ∼1.2-fold increase in the length of L1 but exhibited little or no increase in the length of L2 (Fig. 3b and c). We do note, however, that catp-1(ok2585) mutants exhibited peaks of Pmlt-10::GFP–PEST expression during each molt that were substantially shorter in duration than wild type, although the meaning of this observation is unclear. Overall, we failed to detect a specific lengthening of the L2 stage, nor were strong effects on stage length observed at L3 or L4 stages based on the Pmlt-10::GFP–PEST marker (Fig. 3b and c).
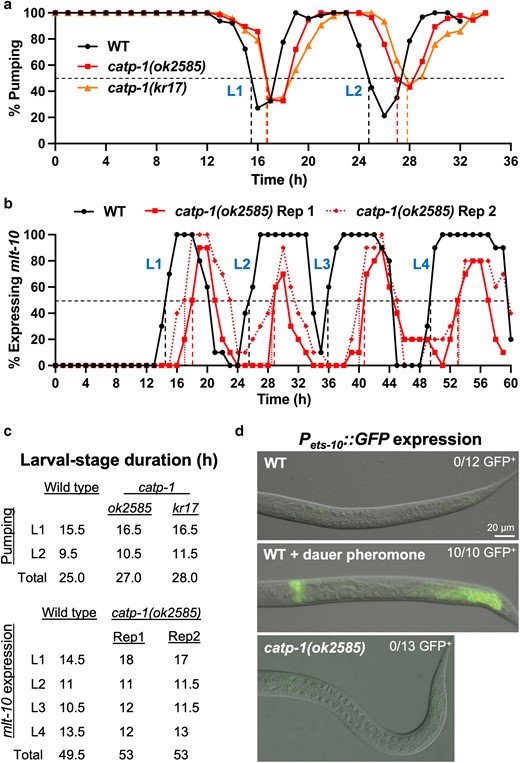
Loss of catp-1 does not substantially extend the length of the L2 stage nor does it induce an L2d marker. a) Cessation of pharyngeal pumping was used to measure the duration of L1 and L2 stages in the indicated backgrounds. b) Pmlt-10::GFP–PEST expression was used to measure the lengths of larval stages in wild-type and catp-1 larvae; 2 replicates are shown for catp-1. Dashed vertical lines in a and b indicate the point at which ≥50% of larvae had entered lethargus for a given stage. c) Quantification of larval-stage lengths for a and b. d) Expression of Pmlt-10::GFP–PEST in L2 larvae under the indicated conditions. In contrast to wild type with dauer pheromone, neither untreated wild type nor catp-1 mutants express the L2d marker. For b) and c), strains were grown at 20°C to match the temperatures used in studies by Ruaud and Bessereau (2007).
As a final test for L2d induction, we determined whether an established marker for L2d is inappropriately expressed under normal growth conditions in catp-1(ok2585) mutants. Pets-10::GFP is mostly undetectable in fed wild-type larvae but is expressed robustly after wild-type larvae are treated with dauer-inducing pheromones (Fig. 3d) (Shih et al. 2019; Binti et al. 2022). We found that catp-1(ok2585) larvae did not express the L2d marker under normal growth conditions. Taken together, our data failed to support the model that loss of CATP-1 activity induces L2d, suggesting that suppression of DMPP toxicity and nekl-associated molting defects by catp-1 mutations occurs through another mechanism.
Discussion
Here, we show that loss of the CATP-1 Na+/K+ exchanger led to the suppression of molting defects in nekl-2; nekl-3 mutants. We also show that a mutation designed to specifically abolish CATP-1 ion pump activity was sufficient to cause robust suppression. This is consistent with a recent report implicating CATP-1 pump activity in glia (Johnson et al. 2020) but contrasts with data from Ruaud and Bessereau (2007) suggesting pump-independent functions for CATP-1 within the context of DMPP toxicity suppression (Johnson et al. 2020). We note that our finding that a CRISPR allele of catp-1(D409E) only weakly suppressed DMPP toxicity could reflect CATP-1 pump-independent functions or may be due to partial inactivation of the pump by this mutation. Consistent with findings by Ruaud and Bessereau (2007), we observed expression of CATP-1 throughout the epidermis Moreover, we observed CATP-1 in punctate and reticular structures at or near the apical surface of epidermal cells, suggesting that CATP-1 may function at the plasma membrane and possibly endosomes, broadly consistent with previous studies on P4-type Na+/K+ pumps (Kaplan 2002; Clausen et al. 2017). Collectively, our data indicate that nekl molting defects may be partially alleviated by altering the concentration or distribution of intracellular solutes or by changes in electrochemical gradients.
One unexpected finding from our genetic analysis was that presumed strong reduction-of-function alleles of catp-1 were not effective at suppressing molting defects in nekl-2; nekl-3 mutants. In contrast, all tested alleles of catp-1 were at least partially effective at reducing the toxicity of DMPP, with the presumed null allele of catp-1(ok2585) appearing to confer the greatest resistance levels. The former observation may be explained in part by a previous report showing that catp-2, a paralog of catp-1, is transcriptionally upregulated >2,000-fold in catp-1(kr17) mutants (Johnson et al. 2020). Thus, upregulation of catp-2 in strong reduction-of-function catp-1 alleles could potentially compensate for loss of CATP-1 function, thereby preventing suppression. This model predicts that sufficient upregulation of catp-2 would not occur in alleles of catp-1 that are capable of suppressing nekl defects.
Based on prior studies of catp-1 and other DMPP toxicity suppressors (Ruaud and Bessereau 2006, 2007; Ruaud et al. 2011), we tested the hypothesis that loss of CATP-1 leads to nekl suppression by inducing a predauer L2d state, which we recently showed can bypass L2/L3 molting defects in nekl-2; nekl-3 mutants (Binti et al. 2022). Our genetic evidence, however, did not support this model. Nevertheless, we did not assess L2 length in catp-1 mutants capable of suppressing nekl defects, leaving open the possibility that certain alleles of catp-1 can induce L2d in a manner that is independent of DAF-5. However, we were also unable to recapitulate a previous finding that loss of catp-1 leads to a marked lengthening of the L2 stage, a hallmark of L2d (Ruaud and Bessereau 2007). Although we have no simple explanation to account for these differences, we note that disparities could potentially arise from altered growth conditions or uncharacterized mutations present in strain backgrounds. It is also worth noting that catp-1 was suggested to suppress DMPP toxicity through a dauer-independent mechanism (Ruaud and Bessereau 2007). Correspondingly, although the suppression of DMPP toxicity by other dauer-inducing mutations correlated strongly with increased L2 duration, it did not correlate with the ability of these mutants to induce mature dauer larvae (Ruaud and Bessereau 2007; Ruaud et al. 2011). Thus, while not fully resolved, our collective data appear to suggest roles for CATP-1 that are independent of the dauer pathway and L2d induction.
WGS of nekl suppressor strains identified 3 isolates containing unique mutations that alter the coding region of catp-1 [WY1286 (fd170), WY1768 (fd168), and WY1744 (fd274)]. Traditionally, the detection of variants at the same locus in 2 or more independent isolates is thought to imply that the common locus is likely responsible for causing the phenotype of interest. This logic is particularly compelling if mutations at that locus have already been shown to be causative and sufficient, as was the case for catp-1 (WY1286). We therefore anticipated that the mutations impacting the coding region of catp-1 in strains WY1768 and WY1744 would be responsible for the observed suppression. Surprisingly, CRISPR phenocopy of these catp-1 variants failed to suppress nekl-2; nekl-3 molting defects, indicating that they are insufficient for suppression. Nevertheless, it is plausible that these mutations may contribute to the observed suppression and, may even be necessary, in conjunction with other loci. More broadly, these findings stress the importance of taking a cautious approach in cases where a known casual gene is identified as a candidate in other sequenced strains.
It was previously shown that the CATP-1a isoform is sufficient for rescuing both DMPP toxicity (Ruaud and Bessereau 2007) and neuronal sensory phenotypes in catp-1 mutants (Johnson et al. 2020), indicating that CATP-1a may be functionally sufficient in epidermal and glial cells, respectively. Interestingly, the failure of the GT > AT catp-1a intron 15 splice-site mutation to suppress nekl-2; nekl-3 mutants suggests that the CATP-1b isoform may be able to partially compensate for the loss of CATP-1a. We note that an alternative in-frame splice donor sequence (GT) is not present within intronic sequences immediately downstream of the splice-site mutation, and although several upstream GT sequences in exon 15 could potentially serve this role, they would lead to the partial removal of the CATP-1a ninth TM domain. Notably, CATP-1b is not predicted to encode a ninth or tenth TM domain, and functional P4-ATPases have been reported to contain as few as 6 TM domains, with TM1–6 making up the functional core (Kuhlbrandt 2004; Morth et al. 2011). Taken together, our data suggest that CATP-1a and CATP-1b may be partially functionally redundant and that both isoforms may encode functional Na+/K+ pumps.
In prior studies, we have shown that NEKL-2 and NEKL-3 localize to several endosomal trafficking compartments, that depletion of NEKL-2 and NEKL-3 leads to morphologically and functionally altered trafficking compartments, and that mutations in several membrane trafficking factors can suppress molting defects associated with nekl loss (Yochem et al. 2015; Lazetic and Fay 2017a; Lazetic et al. 2018; Joseph et al. 2020; Joseph et al. 2023). Thus, it is possible that loss of catp-1 may lead to a partial suppression of nekl molting defects through direct or indirect effects on membrane trafficking. Some support for this model comes from published studies linking cation levels and electrochemical gradients to effects on membrane trafficking. For example, depletion of intracellular potassium leads to the inhibition of clathrin-coated pit formation, potentially by preventing clathrin from interacting with its adapter proteins (Larkin et al. 1983; Heuser and Anderson 1989; Hansen et al. 1993). Correspondingly, we found that inhibition of AP2 clathrin-adapter activation can suppress nekl molting and trafficking-associated defects (Joseph et al. 2020). Na+/K+ pumps also affect the acidification and function of early endosomes by impacting the movement of H+ across endosomal membranes, and the pharmacological inhibition of Na+/K+ pumps leads to endosomal recycling defects (Fuchs et al. 1989; Rosen et al. 2004, 2006; Feldmann et al. 2007). Finally, ionic gradients have been shown to affect vesicle morphology and motility through changes in membrane tension and altered cytoskeletal dynamics (Nunes et al. 2015; Simunovic and Voth 2015; Murray et al. 2017; Mercier et al. 2020; Saric and Freeman 2021).
In addition to the above-mentioned effects on membrane trafficking, Na+/K+ pumps affect various cell signaling pathways, which could represent another means by which catp-1 loss may impact NEKL functions and molting (Davis et al. 1995; Mohammadi et al. 2001; Liang et al. 2006; Doi and Iwasaki 2008; Matchkov and Krivoi 2016; Clausen et al. 2017; Pivovarov et al. 2018). Likewise, analogous to its function in glia, CATP-1 could potentially modify the activity of neurons that are closely associated with the epidermis, which might conceivably impact the molting process (Johnson et al. 2020). It is also worth noting that intracellular potassium oscillations have been implicated in circadian rhythms in a range of cell types (Feeney et al. 2016; Henslee et al. 2017; Rodriguez et al. 2024) and that connections have been made between genes regulating circadian rhythms and molting in C. elegans (Monsalve et al. 2011; Monsalve and Frand 2012; Lazetic and Fay 2017b; Ragle et al. 2020; Patel et al. 2022; Lamberti et al. 2024). At present, however, it remains to be determined precisely how catp-1 and changes in cation concentration can compensate for nekl reduction of function. Future studies will examine how mutations in catp-1 and other identified nekl suppressors impact membrane trafficking processes and whether they suppress 1 or more membrane trafficking defects associated with nekl loss of function.
Data availability
Data from all experiments are given in Supplementary File 3. All reagents generated in this study will be made available upon request. The authors affirm that all data necessary for confirming the conclusions of the article are present within the article, figures, and tables.
Supplemental material available at G3 online.
Acknowledgments
The authors thank Amy Fluet for editing this manuscript, Laura Bianchi (University of Miami) for reagents and helpful discussions, Adison Linder, Zachary Davis, and Rosa Melinda for experimental support, and the C. elegans Gene Knockout Consortium.
Funding
This work was supported by R35 GM136236 from the National Institute of General Medical Sciences and by an Institutional Development Award (IDeA) from the National Institute of General Medical Sciences under (2P20GM103432). Some strains were provided by the CGC, which is funded by the Office of Research Infrastructure Programs, a program within the National Institutes of Health (P40 OD010440).
Literature cited
Author notes
Shaonil Binti and Philip T. Edeen Equivalent contributions.
Conflicts of interest The author(s) declare no conflicts of interest.