-
PDF
- Split View
-
Views
-
Cite
Cite
Eric Accili, When Is a Potassium Channel Not a Potassium Channel?, Function, Volume 3, Issue 6, 2022, zqac052, https://doi.org/10.1093/function/zqac052
- Share Icon Share
Abstract
Ever since they were first observed in Purkinje fibers of the heart, funny channels have had close connections to potassium channels. Indeed, funny channels were initially thought to produce a potassium current in the heart called IK2. However, funny channels are completely unlike potassium channels in ways that make their contributions to the physiology of cells unique. An important difference is the greater ability for sodium to permeate funny channels. Although it does not flow through the funny channel as easily as does potassium, sodium does permeate well enough to allow for depolarization of cells following a strong hyperpolarization. This is critical for the function of funny channels in places like the heart and brain. Computational analyses using recent structures of the funny channels have provided a possible mechanism for their unusual permeation properties.
An Unusual Potassium Current in the Heart
Since the beginning, hyperpolarization-activated cyclic-nucleotide “HCN” channels have been associated closely with potassium channels. Initially, the current produced by HCN channels was identified in Purkinje fibers, which was active during diastole and thought to be carried mainly by potassium.1,2 This current was called IK2, to distinguish it from the inwardly rectifying potassium current IK1 and from another potassium current, which was active during the peak of the action potential, called IK.2,3 However, the reversal potential for IK2 and its dependence on potassium was confusing and not necessarily indicative of this cation as the sole carrier.4 When it was studied in parallel with a similar current in cells of the sinoatrial node,5,6 it became clear that both IK2 and this sinoatrial current were activated by hyperpolarization, blocked by cesium, not blocked by barium, and, remarkably, carried by potassium and sodium.7–10 This led to a dramatic reinterpretation of IK2 as the funny current, found in both Purkinje fibers and sinoatrial pacemaker cells. Movement of sodium through funny channels and into the cell during diastole changed the way that pacemaking was thought to occur.11 The funny current was later identified in neurons where it displayed the same unusual characteristics, including the considerable permeability to sodium.12,13
For a time, the funny channel was classified as a nonselective cation channel despite its similarities to potassium channels (see Table 14.5).14 Funny channels allowed potassium to flow more easily than sodium, with permeability ratios (PNa/PK) ranging from about 0.2 to 0.4, and they were inhibited by cesium, like potassium channels; but, unlike potassium channels, they were known to lack sensitivity to barium or TEA (tetraethylammonium) and allowed larger organic like ammonium or methylammonium to permeate.10,14–19 Potassium channels are also far less permeable to sodium and they generally have a larger single-channel conductance than funny channels.14,20,21 Finally, the whole-cell conductance of the funny current was found to increase steeply when extracellular potassium is raised within a range of concentrations that may be found in the body.16,17,19
Discovery of Ion Channel Genes Provides Answers and Raises More Questions
The identification of ion channel genes brought the funny channel back into the potassium channel fold. This may have begun with the identification of genes in plants that code for hyperpolarization-activated, potassium-selective channels such as KAT1, which open and close in a way that is eerily similar to those processes in funny channels.22,23 The cloning of the funny channel genes revealed strong similarities in primary sequence, as well as evolutionary ties, to voltage-gated potassium channels.24–28 A striking similarity is the “GYG” triplet, the potassium channel amino acid signature sequence,29 which is found in the outer pore of funny channels as well as in most known voltage-gated potassium channels (Figure 1A). This triplet, together with two upstream residues that may include threonine, serine, valine, or isoleucine, forms the “selectivity filter,” which is thought to be responsible for high selectivity and rapid movement of potassium.30
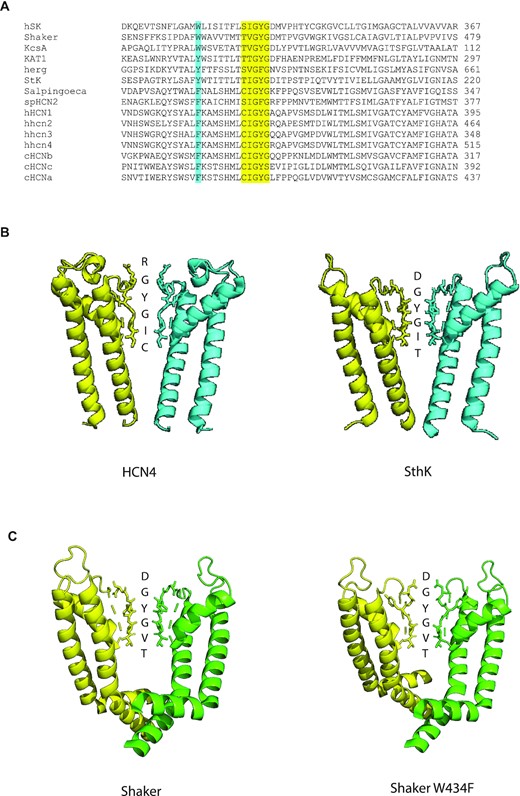
The structure of the pore region of funny channels and other channels containing the GY/FG triplet within the selectivity filter. (A) Amino acid sequence of the pore region in funny channels and other channels containing the GY/FG triplet. The selectivity filter amino acids are highlighted in yellow. The W434 residue of the Shaker channel and the equivalent residue in the other channels are shown in turquoise. The channels shown are the human SK potassium channel, the Shaker voltage-gated potassium channel from Drosophila melanogaster, the KcsA potassium channel from Streptomyces lividans, the KAT1 potassium channel from Arabadopsis thaliana, the human ether-a-go-go potassium channel, SthK, from Spirochaeta thermophila, SR HCN, the funny channel from the single-celled choanoflagellate Salpingoeca rossetta, SpHCN2, one of the funny channel isoforms found in the sea urchin Strongylocentrotus purpuratus, the human HCN1 channel, the human HCN2 channel, the human HCN3 channel, the human HCN4 channel, cHCNb channel from Ciona intestinalis, cHCNc channel from C. intestinalis, and cHCNb channel from C. intestinalis. (B) The solved structure of the pore region of the rabbit HCN4 channel (left; pdb # 7np4) and the SthK channel (right; pdb # 6cjq). Two of four subunits are shown. The regions shown for each subunit span the S5 and S6 transmembrane segments. The five amino acid residues of the selectivity filter and the first residue following the selectivity filter on the outer rim are shown in stick form and are identified by the letter next to it. (C) The solved structure of the pore region of the Shaker potassium channel (pdb # 7sj1) and the Shaker W434F potassium channel (pdb # 7sip) from D. melanogaster. Two of four subunits are shown. The regions shown for each subunit span the S5 and S6 transmembrane segments. The five amino acid residues of the selectivity filter and the first residue following the selectivity filter on the outer rim are shown in stick form and are identified by the letter next to it.
The residues surrounding the “GYG” sequence in the pore are different between eukaryotic funny channels and potassium channels. Mutational studies showed that substitution of a cysteine residue by threonine, which are found at that most inner position in the selectivity filter of funny and potassium channels, respectively, actually made the HCN4 and HCN2 channels less selective for potassium.31,32 Moreover, substitution of this cysteine with alanine in the HCN2 channel did not affect the reversal potential,31 which suggested that the sulfur side group of the former did not interact with permeating cations and that there may be fewer cation binding sites in the selectivity filter. Substitution of cysteine for the innermost threonine residue also did not render the KcsA channel less selective for potassium.33 Thus, regions outside of the selectivity filter influence the relative preference for cations to permeate.
From an evolutionary point of view, it is not known whether the ancestral funny/potassium channel was highly selective for potassium over sodium. Such selectivity may have arisen from less selective channels that contained the “GYG” triplet but gained preference for potassium progressively with subsequent changes outside of this region. Studies on the G protein-coupled inwardly rectifying potassium channel (GirK) channel support this possibility.34 A nonselective version of the GirK channel was transferred to yeast that requires high levels of potassium to survive. In the yeast that subsequently grew in low levels of potassium, mutant GirK channels acquired new mutations well outside of the selectivity filter, which restored some selectivity for potassium and allowed some yeasts to survive. These experiments showed that regions outside of the “GYG” triplet contribute to selectivity for potassium and also demonstrated that this could have been gained progressively and quickly from a less selective ancestor.
Alternatively, funny channels may have arisen from a highly potassium-selective ancestor, which may be more probable based on the following observations. Channels that contain the “GY/FG” triplet exist in eubacterial, archaebacterial, and eukaryotic genomes, which include the single-celled choanoflagellates.35 Most of the “GY/FG”-containing channels that have been studied are very selective for potassium. These include channels in the KAT1 family of plants and the ether-a-go-go (eag) family of animals, both of which contain a cyclic nucleotide binding domain, are highly selective for potassium, and, together with cyclic nucleotide-gated (CNG) channels, are phylogenetically the most closely related channels to funny channels.27,28 Recently, channels have been identified in bacteria that are evolutionarily closest to CNG/eag/HCN channels but possess the canonical selectivity filter residues of potassium-selective channels and one of these, from S. thermophila and called SthK (Figure 1), is very selective for potassium over sodium.36,37 The predominance of potassium selectivity in “GY/FG”-containing channels, including those most closely related to funny channels, and a closely related channel in bacteria that is highly selective for potassium over sodium may be more easily explained by assuming a common ancestor that is also highly selective for potassium over sodium.
Solved Structures Yield More Answers and More Surprises
Based on what was known from functional studies and comparisons between sequences of cloned genes, it was suspected that the structure of the pore would be different between funny and potassium channels. But it was surprising to see such structures, obtained for HCN1 and HCN4 by cryogenic electron microscopy (cryo-EM), because of how different they were from potassium channels and how the difference centres on the conserved “GYG” sequence38,39 (Figure 1B). The “GYG” triplet is oddly shaped in these structures as the outermost glycine residue and tyrosine residue are angled away from the central pore. Therefore, what would be the first two potassium-specific binding sites in a potassium channel are not formed in these funny channels. The two inner sites are formed and the sulfur side groups of the above-mentioned cysteine residue do point away from the central pore as anticipated, which makes the most inner site wider than a typical potassium channel.30 This structure immediately suggests that sodium may permeate more easily once it enters the funny channel pore because its exit is less likely to be impeded by a potassium ion.39
To more deeply understand the pore properties of the HCN4 channel, analyses of permeation were carried out.38 These analyses were assisted by a structure of the HCN4 channel that was solved by cryo-EM in an open state as well as in a closed state. It is unusual to observe an open state at a cross-membrane voltage of, presumably, 0 mV, but it is consistent with previous studies showing that some current flowed when HCN2 channels were at rest.40 The computational analyses showed that potassium, on its own, crosses the pore more easily than does sodium, which was not found to cross at all on its own. The passage of potassium was still relatively infrequent, corresponding to the small single channel conductance. When mixed together, both sodium and potassium traversed the pore, but the former did so less often, in agreement with the PNa/PK ratios obtained previously. The trajectory taken by the ions did not correspond to restricted movement into and between the two formed sites. The cations resided at three locations; between water and the carbonyl of isoleucine, at the plane of the carbonyl of the inner cysteine, between two water molecules and finally at what would be the most inner site. Thus, conduction appeared to follow a soft “knock-on” mechanism, whereby the movement of potassium was coordinated by water, which co-permeates. In summary, the atomic mechanism of permeation in the HCN4 channel shares basic features with that for potassium-selective channels, but the distinctive structure of the selectivity filter yields striking differences, which explain functional differences observed by experiment.
Bauer et al.41 provide important insight into the mechanism of cation conduction in the open HCN4 pore by calculating the potential of mean force (PMF) within the selectivity filter as well as by estimating transition rates through the channel. With only potassium, they found that the energetic barriers were high but, notably, may be lowered by having a potassium ion in the cavity. This is a reasonable explanation for the small single channel conductance (1 pS), which is observed experimentally with high levels of potassium on the internal and external sides of the plasma membrane.20 With only sodium, the energy barrier is so high that this ion remains in the selectivity filter for prolonged periods, which inhibits further movement. Potassium ions were found to bind more strongly to the selectivity filter than sodium ions, which are also dislodged more easily by potassium as opposed to another sodium ion. This may be due to the unusual flexibility of the selectivity filter and altered interactions when different ions enter. These findings would help to explain the greater selectivity for potassium as well as the significant permeation of sodium in mixed potassium and sodium solutions as compared to the smaller or negligible conductance in sodium-only solutions.
In general, these findings appear to be consistent with recent MD simulations and calculations of potential mean force of the closed HCN1 channel.42 In particular, they found the predominance of a single site in the selectivity filter, flexibility of the selectivity filter and the coordination of cation movement within the filter by water.
Although the role for water continues to be debated,43 recent computational studies in potassium channels such as KcsA and a mutant NaK channel with four internal binding sites have suggested that potassium is fully dehydrated and moves through the pore using a hard knock-on mechanism.44,45 By contrast, the movement of sodium through these channels occurs with some water and this ion may bind to the selectivity filter between sites identified in structures, which limits its conduction. Notably, a NaK channel with only three sites allows water to enter the selectivity filter and appears to be nonselective between sodium and potassium.45 Thus, more surprising is that the funny channel is more selective for potassium than might be expected given the fewer number of sites within the selectivity filter.
The insightful study by Bauer et al., raise a number of interesting questions. For example, there are differences in some funny channel isoforms in the sequence of amino acids that surround the selectivity filter as well as within the selectivity filter (Figure 1). Do these differences in sequence result in differences in permeation and selectivity between isoforms? What residues contribute to permeation and how do they do so? The conductance of HCN2 and HCN4, but to a lesser extent in HCN1, is increased by extracellular chloride and a residue on the outer rim of the pore may be important for this effect.46 Does this effect occur by promoting conduction? Does a rise in the extracellular potassium concentration increase conductance by promoting movement of cations through a single channel? Is the mechanism by which these cations permeate changed by disease-associated mutations in funny channels47,48? These questions as well as the overall view proposed by Bauer et al., could be examined by mutating key residues, carrying out simulations on mutant pores to predict possible outcomes, and then testing those predictions by experiment.31,32
The simulations provide a powerful way to examine the evolution of permeation in the funny channel family of ion channels. The selectivity filter of funny channels is “CIGYG” in most known isoforms but not all.49,50 In a funny isoform from the sea urchin S. purpuratus (SpHCN2), the selectivity filter sequence is "SIGFG" but the selectivity profile for this isoform is not known. A phenylalanine substitution within the triplet is also found in the eag family (“GFG,” see herg in Figure 1) but those channels remain selective for potassium. In one of the three funny channel genes that are found in two species of Ciona, the selectivity filter is “CIGYS.” Also, the residue on the outer rim of the pore, mentioned above, varies quite a bit among the funny channel isoforms (see Figure 1). The question of differences in permeation between isoforms becomes even more intriguing when the nature of the chemical environment surrounding the individual channels across taxa and tissues is taken into account.
How is it that the “GYG” triplet assumes such differences in structure between known funny channels and potassium channels? It is clear that residues outside of this triplet are responsible but which residues and regions, and what are the critical interactions? Evolutionary analyses, perhaps using selected channels that are either closely related to funny channels or even considered as funny channel isoforms, in combination with functional experiments and simulations may provide some insight. For example, the SthK channel from bacteria is very similar to eukaryotic CNG and HCN channels based on their primary sequence but it is highly selective for potassium over sodium and possesses a selectivity filter that is more like typical potassium-selective channels in structure (Figure 1B). Ether-a-g–go channels, too, are closely related to funny channels and CNG channels, as well as the SthK channel, but, like the SthK channel, they are very selective for potassium.51–53 Interestingly, sodium is also thought to block ion flow through the herg channel pore; this can be relieved by extracellular potassium, which leads to increases in conduction when the extracellular concentration of this cation is increased.54 More broad functional analyses of funny channels and closely related channels, in all kingdoms, would be enlightening because they may or may not have the same permeation properties as known funny channels.
It is notable that the unusual conformation of the selectivity filter of the HCN channel is similar to that for the Shaker W434F potassium channel, which is a voltage-gated and depolarization-activated channel. The W434F version of the Shaker channel is thought to be, functionally, in a C-type-inactivated state.55,56 C-type inactivation in voltage-gated potassium channels is a reduction in ion flow that occurs normally upon depolarization of the membrane potential.57 The selectivity filter of Shaker is thought to undergo a change in structure during this C-type inactivation process, which is also marked by an increase in the permeability of sodium.58,59 Structures for the W434F Shaker channel and the Shaker wild type were recently solved by cryo-EM.60 The selectivity filter for the wild-type Shaker channel is similar to those for other potassium-selective channels, including the SthK channel (Figure 1A and C). The W434F filter retains the two inner sites but the pore becomes wider where the tyrosine residue of the “GYG” is swung outward, like the selectivity filter for the HCN channel and unlike selectivity filter for the wild-type Shaker channel (Figure 1C). Notably, a phenylalanine is also found near the selectivity filter, in the pore helix and at the position equivalent to W434 in the HCN isoforms, but it is not found in any of the potassium-selective channels, even those that are closely related to HCN (Figure 1A). Thus, this could be a site that contributes to the unusual shape of the HCN selectivity filter. Together, the findings may even suggest that HCN channels are simply inactivated voltage-gated potassium channels with modifications.
Although permeation has not been directly compared between HCN and Shaker W434F, there are similarities. Macroscopic sodium currents are observed in both Shaker W434F and HCN2-expressing Chinese hamster ovary cells when potassium is not present in either the external or internal solution.31,59 Outward currents are also dramatically reduced in Shaker W434F when potassium, as well as sodium, is present inside the cell suggesting that the former inhibits the latter. By contrast, inward permeation of sodium through funny channels is thought to require external potassium when potassium is present inside the cell and outward current is also observable when potassium and sodium are present both inside and outside.18,61 Experimental studies could help to appropriately compare and contrast permeation of sodium and potassium in these two channels as would further simulations of conduction, which focused on potassium, but not sodium, in the study of Shaker W434F.60 Simulations that were done in the W434F mutant, at large depolarizations, suggested that conduction was impaired, but not blocked, and that a larger energetic barrier for potassium movement resulted from the dilation of the selectivity filter.
The simulations by Saponaro et al., and some in Bauer et al., were carried out at very negative voltages (≤−250 mV), which were necessary because of the small conductance within the 1–2 μs time window. Free energy landscape, as well as the movement of ions and their interactions with each other, also depends on the magnitude of the voltages encountered. For example, it is known that cesium inhibits funny current to a greater extent at more negative voltages due to block of the conduction pathway,17 which suggests that conduction and interactions between ions and protein may depend on voltage. The simulations were also carried out on a pore that is open under resting conditions. It may be that the pore structure differs when hyperpolarized, when the voltage sensor and associated regions have moved. The HCN selectivity filter could undergo further changes in conformation, which regulate conduction in a way that depends on voltage and longer times, as has been shown, for example, in the bacterial sodium channel.62 When it becomes possible, it will be interesting to find out whether the mechanisms proposed by Bauer et al., and Saponaro et al., such as the block by sodium, the co-permeation of water, the flexibility of the pore, and facilitation of conduction by potassium, hold for longer simulations, between different isoforms and at voltages and ionic conditions that occur in the body.44
In Summary
After a tumultuous beginning, it is now known that funny channels are part of a larger family of voltage-gated channels, which includes many close members that are highly selective for potassium. Potassium does pass more easily than sodium in funny channels and they possess a potassium channel-like pore, which includes a selectivity filter that contains the ubiquitous “GYG” triplet. However, the considerable permeation of sodium endows funny channels with unique contributions to cell function. Funny channels also have a very small single-channel conductance, smaller than known potassium channels, and their conductance has an unusual dependence on extracellular potassium and chloride, which could also be of consequence functionally wherever they are expressed. It has been difficult to understand these unusual properties and how they are controlled at the atomic level by specific structures, in different isoforms and under different conditions. The computational analyses of Bauer et al., along with those of Saponaro et al., and Ahrari et al., made possible by the recently solved funny channel structures,38,39 have provided (finally!) a structural and functional model of permeation that can be effectively tested by experiment, modeling, and simulation.
Funding
National Science and Engineering Council of Canada (NSERC) Canadian Institutes of Health Research (CIHR) The Heart and Stroke Foundation of Canada (HSFC)
Conflict of Interest Statement
None declared.
Data Availability Statement
No new data were generated or analyzed in support of this research.