-
PDF
- Split View
-
Views
-
Cite
Cite
Yen-Yu Lu, Shih-Yu Huang, Yung-Kuo Lin, Yao-Chang Chen, Yi-Ann Chen, Shih-Ann Chen, Yi-Jen Chen, Epicardial adipose tissue modulates arrhythmogenesis in right ventricle outflow tract cardiomyocytes, EP Europace, Volume 23, Issue 6, June 2021, Pages 970–977, https://doi.org/10.1093/europace/euaa412
- Share Icon Share
Abstract
Ventricular arrhythmia (VA) frequently occurs in fatty infiltrative cardiomyopathy or epicardial adipose tissue (EAT) abundant hearts. Right ventricular outflow tract (RVOT), commonly covered with EAT, is vital for VA genesis. This study explored whether EAT contributes to RVOT arrhythmogenesis.
Conventional microelectrodes and whole-cell patch clamp were used to record electrical activity and ionic currents in rabbit RVOT tissue preparation or isolated single cardiomyocytes with or without (control) connected EAT. Epicardial adipose tissue-connected (N = 6) RVOT had more portions of fibrosis than did control (N = 5) RVOT (160.3 ± 23.2 vs. 91.9 ± 13.4 μm2/mm2, P < 0.05). Epicardial adipose tissue-connected RVOT cardiomyocytes (n = 18) had lower negative resting membrane potential (−68 ± 1 vs. −73 ± 2 mV, P < 0.05); smaller action potential (AP) amplitude (108 ± 4 vs. 135 ± 6 mV, P < 0.005); and longer 90%, 50%, and 20% of AP duration repolarization (361 ± 18 vs. 309 ± 9 ms, P < 0.05; 310 ± 17 vs. 256 ± 13 ms, P < 0.05; and 182 ± 19 vs. 114 ± 24 ms, P < 0.05, respectively) than did control (n = 13) RVOT cardiomyocytes. Moreover, compared with control RVOT cardiomyocytes, EAT-connected RVOT cardiomyocytes had larger transient outward potassium currents, similar delayed rectifier potassium currents, smaller L-type calcium currents, and inward rectifier potassium currents. After ajmaline (10 μM, a sodium channel blocker) superfusion, high VA inducibility was observed through rapid pacing in EAT-connected RVOT but not in control RVOT.
Epicardial adipose tissue exerts distinctive electrophysiological effects on RVOT with a propensity towards VA induction, which might play a role in lipotoxicity pathogenesis-related ventricular arrhythmogenesis.
Epicardial adipose tissue (EAT) might play a vital role in the genesis of cardiac arrhythmia in lipotoxicity pathogenesis-related ventricular arrhythmogenesis.
Right ventricular outflow tract (RVOT), commonly covered with EAT, is vital for ventricular arrhythmia (VA) genesis.
Epicardial adipose tissue could modulate electrical activities of RVOT through a larger transient outward potassium currents, similar delayed rectifier potassium currents, smaller L-type calcium currents, and inward rectifier potassium currents.
Epicardial adipose tissue exerts distinctive electrophysiological effects on RVOT with a propensity towards VA induction after ajmaline (10 μM, a sodium channel blocker) superfusion and rapid pacing.
Introduction
The occurrence of ventricular arrhythmia (VA) in fatty infiltrative cardiomyopathy or epicardial adipose tissue (EAT) abundant heart suggests the arrhythmogenic potential of EAT.1 Obesity or metabolic syndrome is associated with an increasing EAT amount or EAT phenotype modulation.2,3 Fatty infiltration may impair heart function and provide a substrate for arrhythmogenesis by modulating cardiac electrophysiology through the effects of adipocytokines or cell interaction.4–6 Studies have revealed that epicardial adipocytes can directly modulate the electrophysiology of atrial cardiomyocytes and increased arrhythmogenesis.7,8 However, the role of EAT in VA remains unclear. A study showed that the amount of pericardial fat in patients is highly correlated with the frequency of ventricular premature beats,9 suggesting the arrhythmogenic potential of EAT.
The right ventricular outflow tract (RVOT) is an essential factor underling VA genesis in idiopathic ventricular tachycardia (VT), Brugada syndrome, and long QT syndrome.10–13 In our previous study, we found that RVOT myocytes contain distinctive electrophysiological characteristics with a large Ca2+ content, transient outward current (Ito), large Phase 1 notch, and high incidence of ouabain-induced VT.14 The RVOT is frequently covered with EAT, which is in the vicinity of the ablation target of Brugada syndrome,15 suggesting a correlation between EAT and ventricular arrhythmogenesis. A clinical study revealed that in patients with Brugada syndrome, ventricular fibrillation is frequently induced at the RVOT-free wall region but less frequently at the RVOT septal region, right ventricle apex, or left ventricle.16 Because the distribution of EAT on the epicardium surface is uneven, the resulting electrophysiological change may cause regional or transmural electrophysiological heterogeneities. Systemic proarrhythmic effects induced by RV pericardial fat may play a crucial role in VA development. The expanded EAT in obese individuals, an essential source of inflammatory mediators, has been reported to potentially underlie the arrhythmogenesis of cardiomyocytes.1,4,7,8 Accordingly, this study aimed to investigate whether EAT modulates electrophysiological characteristics and ionic currents in RVOT myocytes, which may underlie the mechanism of lipotoxicity-related VA.
Methods
Isolation of single cardiomyocytes
The investigation was approved by the local review board (LAC-2019-0571, Taipei Medical University Institutional Animal Care and Use Committee) and conformed to the Institutional Guide for the Care and Use of Laboratory Animals and the Guide for the Care and Use of Laboratory Animals published by the US National Institutes of Health. As described previously,7 male New Zealand white rabbits (weighing 2–3 kg) were sedated with zoletil (10 mg/kg; Virbac, Carros, France) and xylazine (5 mg/kg; Bayer, Leverkusen, Germany) administered through intra-muscular injection. Furthermore, anesthetization was confirmed based on a lack of corneal reflex and motor response to pain stimuli. A midline thoracotomy was then performed, and the heart and lungs were removed as described previously. Hearts were mounted on a Langendorff apparatus to be superfused in an antegrade manner with oxygenated normal Tyrode’s solution at 37°C containing (in mM) NaCl (137), KCl (5.4), CaCl2 (1.8), MgCl2 (0.5), HEPES (10), and glucose (11) adjusted to pH 7.4 with NaOH. After the hearts were cleaned of blood, the perfusate was replaced with oxygenated Ca2+-free Tyrode’s solution containing 300 units/mL of collagenase type I (Sigma, St. Louis, MO, USA) and 0.25 units/mL of protease type XIV (Sigma) for 8–12 min. The free wall of the RVOT (area within 5 mm below the pulmonary valve) was excised and was cut along the border of EAT in experimental group (namely, RVOT with EAT) and roughly the same region without EAT in control group (RVOT without EAT) as shown in Figure 1. Subsequently, the RVOT-free wall was gently rotated in 50 mL of Ca2+-free oxygenated Tyrode’s solution until single cardiomyocytes were obtained. The solution was then gradually changed to normal oxygenated Tyrode’s solution. Cardiomyocytes were allowed to stabilize in the bath for at least 30 min before experiments.
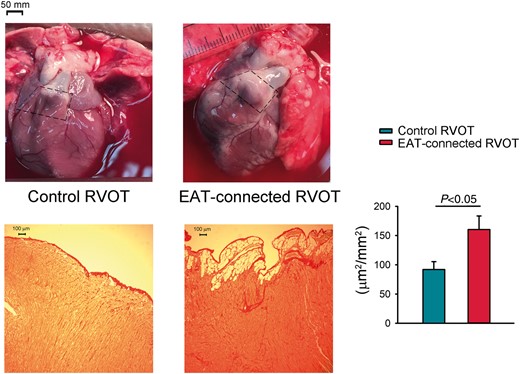
Pictures illustrate RVOT with and without connection to the adipose tissue. The selected area marked by dash line are examples of RVOT with (EAT-connected RVOT) and without connected EAT (control RVOT). Fibrotic remodelling of the subepicardium in RVOT samples with (right lower) or without EAT (left lower). The extent of fibrotic was assessed on Sirius Red stained sections. Bar figure represent subepicardial fibrosis (portions of fibrosis area/epicardial tissue) in EAT-connected (N = 6) and control (N = 5) RVOT specimens. *P < 0.05. EAT, epicardial adipose tissue; RVOT, right ventricular outflow tract.
Electrophysiological study
As previously described,7,14 a whole-cell patch clamp was used with an Axopatch 1D amplifier (Axon Instruments, Foster City, CA, USA) at 35 ± 1°C for obtaining single isolated cardiomyocytes. The borosilicate glass electrodes (optical density, 1.8 mm) used had tip resistances of 3–5 MΩ. Before the formation of the membrane-pipette seal, tip potentials were zeroed in Tyrode’s solution. Junction potentials between the bath and pipette solution (9 mV) were corrected for action potential (AP) recordings. Action potentials were recorded in the current-clamp mode, and ionic currents were measured in the voltage-clamp mode. A small hyperpolarizing step was delivered from a holding potential of −50 mV to a testing potential of −55 mV for 80 ms at the beginning of each experiment. The area under the capacitative current was divided by the applied voltage step to obtain the total cell capacitance. Normally, 60–80% series resistance is electronically compensated. Action potentials were elicited from isolated cardiomyocytes without spontaneous activity at a driven rate of 1 Hz for 20 beats. The resting membrane potential (RMP) was measured during the period between the last repolarization and the onset of subsequent AP. The AP amplitude (APA) was obtained from the RMP to the peak of AP depolarization. The AP duration (APD) at 90%, 50%, and 20% repolarization was measured as APD90, APD50, and APD20, respectively. In cells with APs with a spike-and-dome morphology, the magnitude of Phase 1 notch was measured as the membrane potential between the peak of Phase 0 and the end of Phase 1. Micropipettes were filled with a solution containing the following (in mM): for L-type Ca2+ current (ICa-L), CsCl (130), MgCl2 (1), MgATP (5), HEPES (10), EGTA (10), NaGTP (0.1), and Na2 phosphocreatine (5) at a pH of 7.2 with CsOH; for sodium current (INa), CsCl (133), NaCl (5), EGTA (10), MgATP (5), TEACl (20), and HEPES (5) at a pH of 7.3 with CsOH; for late sodium current (INa-Late), CsCl (130), Na2ATP (4), MgCl2 (1), EGTA (10), and HEPES (5) at a pH of 7.3 with NaOH; for the rapid component of delayed rectifier potassium currents (IKr-tail), KCl (120), MgCl2 (5), CaCl2 (0.36), EGTA (5), HEPES (5), glucose (5), K2-ATP (5), Na2 phosphocreatine (5), and Na-GTP (0.25) at a pH of 7.2 with KOH; and for AP and Ito, KCl (20), K aspartate (110), MgCl2 (1), MgATP (5), HEPES (10), EGTA (0.5), LiGTP (0.1), and Na2 phosphocreatine (5) at a pH of 7.2 with KOH.
INa was recorded during depolarization from a holding potential of −120 mV to test potentials ranging from −80 to 0 mV in 5 mV steps for 40 ms at a frequency of 3 Hz at room temperature (25 ± 1°C) with an external solution containing (in mM) NaCl (5), CsCl (133), MgCl2 (2), CaCl2 (1.8), nifedipine (0.002), HEPES (5), and glucose (5) at pH 7.3.
INa-Late was recorded at room temperature with an external solution containing (in mM) NaCl (130), CsCl (5), MgCl2 (1), CaCl2 (1), HEPES (10), and glucose (10) at a pH of 7.4 with NaOH by using a step/ramp protocol (−100 mV step to +20 mV for 100 ms, and then ramp back to −100 mV over 100 ms). INa-late was measured from the baseline to the peak of the tetrodotoxin (30 µM)-sensitive portions of current traces obtained when voltage was ramped back to −100 mV.
The ICa-L was measured as an inward current during depolarization from a holding potential of −50 mV to test potentials ranging from −40 to +60 mV in 10 mV steps for 300 ms at a frequency of 0.1 Hz using a perforated patch clamp with amphotericin B. NaCl and KCl in the external solution were, respectively, replaced with tetraethylammonium chloride and CsCl.
Ito was studied using a double-pulse protocol. A 30 ms pre-pulse from −80 to −40 mV was used to inactivate sodium channels, followed by a 300 ms test pulse to +60 mV in 10 mV steps at a frequency of 0.1 Hz. CdCl2 (200 μM) was added to Ca2+-free normal Tyrode’s solution to inhibit ICa-L as described previously. Ito was measured as the difference between the peak outward current and the steady-state current.
Inward rectifier potassium current (IK1) was activated from −40 mV to test potentials ranging from −20 to −120 mV in 10 mV steps for 1 s at a frequency of 0.1 Hz under an infusion of CdCl2 (200 μM) and 4-aminopyridine (2 mM) in the extracellular solution.
IKr-tail was measured as the outward current density at the end of 3 s, and depolarization was observed from −40 to +60 mV in 10 mV steps at a frequency of 0.1 Hz during the infusion of chromanol 293B (30 µM) in the external solution containing (in mM) NaCl (140), KCl (1.5), NaH2PO4 (0.33), MgCl2 (0.2), CaCl2 (0.2), CdCl2 (0.2), HEPES (5), and glucose (7.5) at pH 7.4 with NaOH.
Right ventricular outflow tract and epicardial adipose tissue preparations and electro-pharmacological study
As shown in Figure 1, RVOT preparations with and without attached EAT (EAT-connected RVOT and control RVOT, respectively) were used. One end of the preparation was pinned with needles on top of the epicardial fat to the bottom of a tissue bath of Tyrode’s solution with a composition (in mM) of NaCl (137), KCl (4), NaHCO3 (15), NaH2PO4 (0.5), MgCl2 (0.5), CaCl2 (2.7), and dextrose (11) with pH adjusted to 7.4 through titration with NaOH. The other end was connected to a Grass FT03C force transducer with a silk thread. The tissue strips were superfused at a constant rate (3 mL/min) with Tyrode’s solution, which was bubbled with 97% oxygen and 3% carbon dioxide gas at 37°C, and preparations were allowed to equilibrate for 10 min before the electrophysiological study. Tissue preparations were connected to a WPI Duo 773 electrometer by using a 150 mg load. The endocardial side of preparations faced upward, and mechanical events were recorded using Tektronix TDS2000C digital storage oscilloscope and Gould TA11 recorder (OH, USA). Signals were recorded digitally with 16-bit accuracy at a rate of 125 kHz. For electrical stimulation, a 1 ms pulse was provided through a Grass S88 stimulator by using a Grass SIU5B stimulus isolation unit. Ajmaline (10 μM, sodium channel blocker, Sigma-Aldrich, St. Louis, MO, USA) was used to superfuse the tissue for 30 min. High-frequency electrical stimuli (20 Hz) were used to induce VAs.
Histological study of rabbit samples
Right ventricular outflow tract with or without EAT-connected samples, fixed in 4% paraformaldehyde and embedded in paraffin, were used for the quantification of fibrosis, fatty infiltrates, and inflammatory cells. After deparaffinization, sections were stained with Sirius Red and Haematoxylin Eosin. The fibrotic extent of fatty infiltrates was assessed on Sirius Red stained sections. The fibrosis/subepicardium area portions within each sample were localized and measured in the cross-sectional areas of them. Data were averaged from five random selected subepicardial regions in each specimen of the three specimens obtained from each animal. The extensiveness of fibrotic remodelled subepicardium was assessed using automated digital processing (Image-Pro Plus, Media Cybernetics, USA).
Statistical analysis
All quantitative data are expressed as the mean ± standard error of the mean. Statistical significance between different groups was determined using a paired or unpaired t-test depending on the results of the normality test. Nominal variables were compared using a χ2 analysis with Pearson’s correlation. A value of P <0.05 was considered statistically significant.
Results
Histological study of epicardial adipose tissue-connected or control right ventricular outflow tract specimens
Figure 1 reveals samples of RVOT with or without EAT connected and their histological study. There were significantly more portions of fibrosis infiltration in the subepicardial region of the RVOT with EAT-connected specimens in comparison with RVOT without EAT-connected specimens (160.3 ± 23.2 vs. 91.9 ± 13.4 μm2/mm2, P < 0.05).
Electrophysiological characteristics of epicardial adipose tissue-connected or control right ventricular outflow tract myocytes
As shown in Figure 2, EAT-connected RVOT myocytes had significantly higher RMP, lower APA, and larger APD90, APD50, and APD20 than did control RVOT myocytes. However, the Phase 1 notch was similar in EAT-connected RVOT myocytes and in control RVOT myocytes. Figure 3 shows the effects of EAT on potassium currents. We found that compared with control RVOT myocytes, EAT-connected RVOT myocytes had larger Ito, similar IKr-tail, and smaller IK1. Moreover, Figure 4 reveals that ICa-L was smaller in EAT-connected RVOT myocytes. However, no significant difference was noted in INa or INa-Late between EAT-connected and control RVOT myocytes (Figure 5).
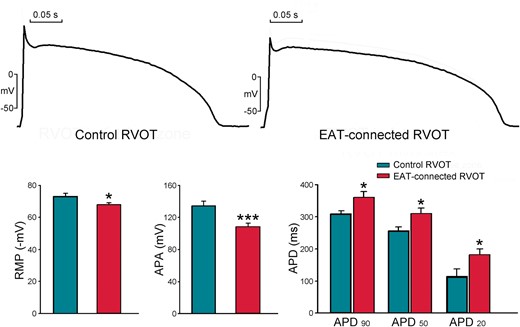
Effects of EAT adipocytes on the electrical activity of RVOT cardiomyocytes. Examples and average data of RMP, APA, and APD90, APD50, and APD20 in EAT-connected (n = 18) and control (n = 13) RVOT myocytes. *P < 0.05 and ***P < 0.005. APA, action potential amplitude; EAT, epicardial adipose tissue; RMP, resting membrane potential; RVOT, right ventricular outflow tract.
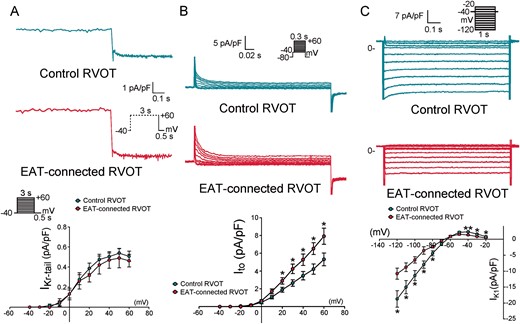
Effects of EAT adipocytes on the potassium currents of RVOT cardiomyocytes. (A) Examples and average data of delayed rectifier current (IKr-tail) in EAT-connected (n = 9) and control (n = 11) RVOT myocytes. (B) Examples and average data of Ito in EAT-connected (n = 12) and control (n = 12) RVOT myocytes. (C) Examples and average data of IK1 in EAT-connected (n = 13) and control (n = 12) RVOT myocytes. *P < 0.05. Inset shows the various clamp protocols. EAT, epicardial adipose tissue; RVOT, right ventricular outflow tract.
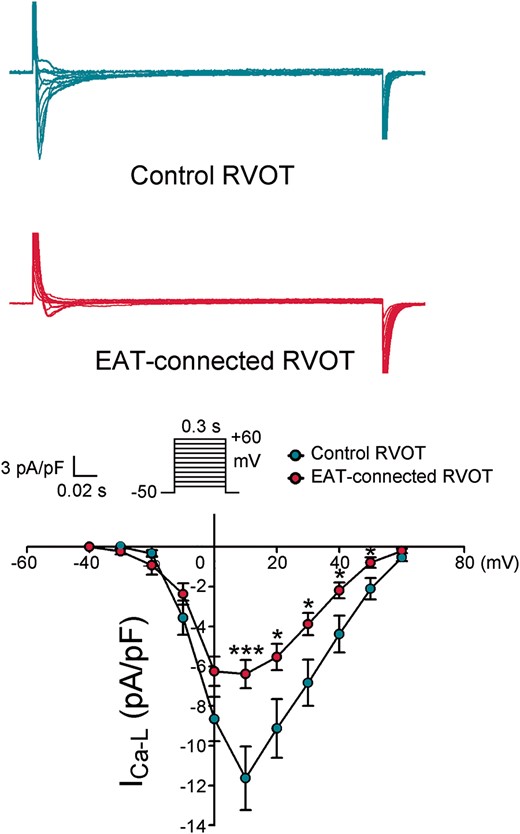
Effects of EAT on ICa-L in RVOT cardiomyocytes. Examples of current tracings and the I–V relationship of ICa-L in EAT-connected (n = 14) and control (n = 11) RVOT myocytes. **P < 0.01. Inset shows the various clamp protocols. EAT, epicardial adipose tissue; RVOT, right ventricular outflow tract.
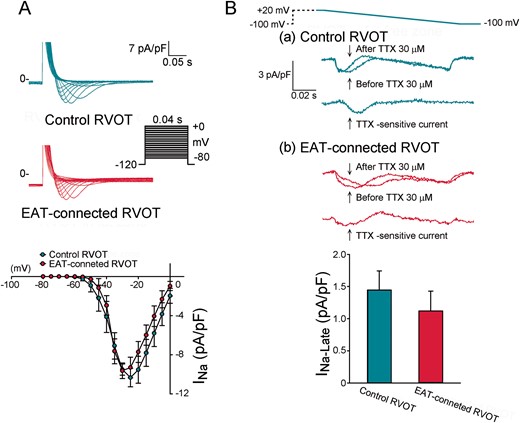
Effects of EAT on INa and INa-late in RVOT cardiomyocytes. (A) Current traces and I–V relationship of the INa in EAT-connected (n = 9) and control (n = 9) RVOT myocytes. (B) Current traces and I–V relationship of INa-late in EAT-connected (n = 10) and control (n = 10) RVOT myocytes. Inset shows the various clamp protocols. EAT, epicardial adipose tissue; RVOT, right ventricular outflow tract.
Effects of ventricular arrhythmia inducibility in right ventricular outflow tract with epicardial adipose tissue
Figure 6 reveals examples of VA inducibility in control RVOT and EAT-connected RVOT. Through burst RV rapid pacing (20 Hz), the incidence of VA occurrence in EAT-connected and control RVOTs was non-significantly different (12.5% vs. 0%, P = NS). However, after ajmaline (10 µM) superfusion, burst pacing could induce non-sustained VA in EAT-connected RVOT but not in control RVOT (100% vs. 0%, P < 0.005). These findings suggest that EAT-connected RVOT is highly vulnerable to the proarrhythmic effect of sodium channel blockade.
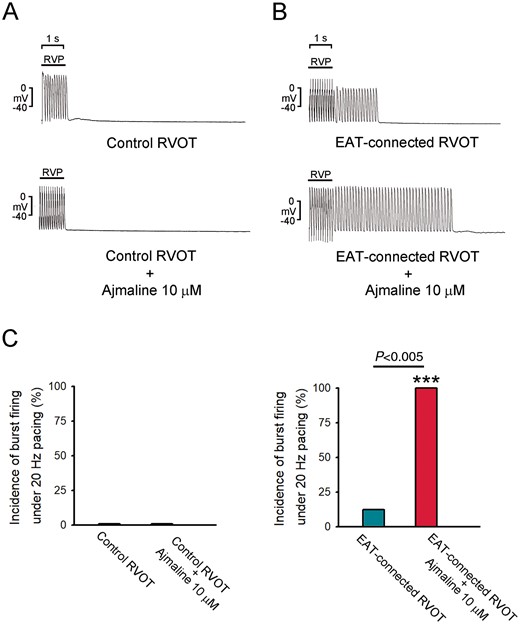
Effects of VA inducibility in EAT-connected and control RVOTs. (A) Examples of burst pacing alone in EAT-connected and control RVOTs. (B) Examples of burst pacing plus ajmaline (10 μM) superfusion in EAT-connected (N = 8) and control RVOTs (N = 6). (C) Average data of VA induction in different RVOT preparations. ***P < 0.005 (vs. control RVOTs plus ajmaline 10 μM). EAT, epicardial adipose tissue; RVOT, right ventricular outflow tract; VA, ventricular arrhythmia.
Discussion
This is the first study to demonstrate that the electrophysiological property of RVOT, the most common site of ventricular premature contraction (VPC), and VA, could be influenced by EAT with ionic currents and subsequent electrical activity modulation. Most importantly, these modulations could enhance VA induction in RVOT, which would, at least in part, explain the cluster of VA in RVOT and the pathogenesis of lipotoxicity-related VA.
Our previous investigation revealed that EAT prolonged the APD in left atrial myocytes, suggesting a higher arrhythmogenic activity of EAT than of adipose tissue of other locations.7 Additionally, EAT of failed hearts has more fat-induced atrial arrhythmia than EAT of healthy hearts, indicating that heart failure may have a high risk of atrial arrhythmia due to changes in EAT adipocytokines.8 In this study, we found that EAT-connected RVOT myocytes had longer APD and increased RMP, suggesting that EAT may change RVOT electrophysiological characteristics, which was similar to EAT effects on left atrial myocytes.7 Additionally, we found a smaller APA in EAT-connected RVOT myocytes than in control RVOT myocytes. Positive RMP in EAT-connected RVOT may lead to high arrhythmogenesis due to the decreasing threshold of abnormal triggered activity, which is in line with the clinical observation that triggering premature ventricular contractions initiated VA are at the RVOT in patients with Brugada syndrome.13
Although burst pacing alone could not induce increased VA in EAT-connected RVOT compared with control RVOT, in the presence of ajmaline, burst pacing could induce significantly more VA in EAT-connected RVOT than in control RVOT. Right ventricular outflow tract is the most common site of VA and is also frequently covered with EAT.10–16 Therefore, these findings highlight the crucial role of EAT in lipotoxicity-related VA. Moreover, the uneven distribution of EAT along with the prolonged APD might create regional heterogeneity of APD, providing a functional substrate for VA. Antzelevitch et al.17 suggested a mechanism of VA named Phase 2 re-entry, which was associated with the difference in the APD of the ventricular epicardial wall, leading to the propagation of excitation from one site of a long APD with Phase 2 dome to another site of a short APD without Phase 2 dome, thus generating a re-entrant cycle. Although the Phase 1 notch is not bigger in EAT-connected RVOT, the prolonged APD still indicates a potential environment for VA. Moreover, the less negative RMP and prolonged APD might lead to abnormal triggered activity and might account for, at least in part, the occurrence of VPCs.
The study of ionic currents revealed that EAT influenced some potassium currents. IK1 was more depressed in EAT-connected RVOT than in control RVOT, which might lead to less negative RMP. A large Ito, an essential factor in APD difference generation, had higher propensity for a large Phase 1 notch, although not shown in this study, probably due to inadequate difference. Because Ito may shorten the APD, which was contradictory to our finding, the enhanced Ito might be a physiological response of repolarization homeostasis to antagonize APD prolongation. Ito exists in large amounts at the epicardial site of the right ventricle, which might lead to Phase 2 re-entry as in the model of Brugada syndrome as suggested in the repolarization disorder hypothesis.17 A large Ito in EAT-connected RVOT myocytes might potentiate the clinical finding that ventricular fibrillation is mostly induced in the RVOT-free wall, a site frequently covered with EAT. IKr-tail was not different between RVOT with EAT and control RVOT. Accordingly, the APD prolongation in RVOT with EAT would not be caused by IKr-tail, and the decreased ICa-L might be responsible for the long APD in EAT-connected RVOT.
The depolarization disorder hypothesis involved conduction slowing with late potentials, which reflect delayed and fragmented ventricular conduction and might serve as the ablation target in RVOT of Brugada syndrome.15 Sodium channel dysfunction has been described in the RVOT of Brugada syndrome, with conduction delay in the RVOT area.18,19 However, in our study, sodium currents, including INa and INa-Late, were similar in both EAT-connected RVOT and control RVOT groups, indicating that slow conduction by EAT might not be due to the modulation of the sodium current. Nevertheless, EAT and the associated increased fibrosis could anatomically disrupt cell-to-cell electrical conduction of myocytes, which might result in slow conduction, facilitating arrhythmogenesis. Additionally, burst pacing plus ajmaline superfusion in EAT-connected RVOT induced much more VA, which indicate that suppression of sodium current by ajmaline in conjunction with large Ito caused by EAT might create ventricular arrhythmogenesis even in a healthy heart.
The fatty heart is frequently noted in metabolic disorders as well as diastolic dysfunction.20 The proposed mechanisms of how fatty infiltration promoting ventricular arrhythmogenicity includes provoking automaticity and degenerating adjacent myocardial cells were at least partially supported by this study. Taken together, EAT might enhance VA occurrence through increased trigger activity or re-entrant circuit genesis due to functional or electrical disturbance caused by an anatomical substrate (anatomical block to the propagation of an electrical impulse).
Study limitations
Classical glass micro-electrode is the gold standard to record AP, however, is hindered by adjacent epicardial fat while performing on epicardium. Although the contractility of myocytes given the role played by ICa-L in the excitation–contraction coupling process, however, the contractility of endocardial or epicardial site of RVOT could not be differentiated in current preparation. Despite the fact that VA could be induced by burst pacing, we did not perform programmed electrical stimulation to study the inducibility of VA. Future experiments are mandatory to explore the role of EAT in the inducibility of VA in different settings. In the patch clamp experiment, calcium channel blockers, the agents used to record unitary potassium channel may potentially modulate kinetics of potassium currents. Therefore, the application of CdCl2 in this study may underestimate the EAT effect on potassium currents.
Conclusions
Epicardial adipose tissue may prolong AP duration and give rise to more positive RMP through the smaller L-type calcium currents, inward rectifier potassium currents and larger transient outward potassium currents, which provide potential substrate for ventricular arrhythmogenesis and higher inducibility of VA in RVOT with EAT connected after ajmaline superfusion and rapid pacing, which might play a role in lipotoxicity-related ventricular arrhythmogenesis.
Funding
This work was supported by grants from the Ministry of Science and Technology (MOST107-2314-B-038-101-MY3, MOST107-2314-B-038-097-MY2, MOST108-2314-B-281-008, MOST108-2314-B-038-118, MOST108-2314-B-281-007-MY3, and MOST108-2314-B-016-048), Taipei Medical University-Wan Fang Hospital (107-wf-swf-02, 107-wf-eva-13, 108-wf-eva-06, 108-wf-swf-01, 109-wf-eva-04, 109-wf-eva-18, and 109-wf-swf-09), Cathay General Hospital (105CGH-TMU-08 and 108CGH-TMU-05), Chi-Mei Medical Center (108CM-TMU-04 and CMNDMC10911), and the Ministry of National Defense-Medical Affairs Bureau (MND-MAB-110-085), Taiwan.
Conflict of interest: none declared.
Data availability
The data underlying this article will be shared on reasonable request to the corresponding author.
References
Author notes
Yen-Yu Lu and Shih-Yu Huang authors contributed equally to the study.