-
PDF
- Split View
-
Views
-
Cite
Cite
Timothy M Markman, Mirmilad Khoshknab, Saman Nazarian, Catheter ablation of atrial fibrillation: cardiac imaging guidance as an adjunct to the electrophysiological guided approach, EP Europace, Volume 23, Issue 4, April 2021, Pages 520–528, https://doi.org/10.1093/europace/euaa249
- Share Icon Share
Abstract
Catheter ablation is increasingly utilized to treat patients with atrial fibrillation (AF). Despite progress in technology and procedural strategy, there remain significant limitations with suboptimal outcomes. The role of imaging has continued to evolve, and multimodality imaging now presents an important opportunity to make substantial progress in the safety and efficacy of ablation. In this review, we discuss the history of imaging in the ablation of AF with a specific focus on the ability of cardiac computed tomography and magnetic resonance imaging to characterize anatomy, arrhythmogenic substrate, and guide ablation strategy. We will review the progress that has been made and highlight many of the limitations as well as future directions for the field.
Introduction
Atrial fibrillation (AF) is the most common arrhythmia and imparts significant worldwide morbidity and mortality. Catheter ablation has been established as an effective treatment for decreasing AF burden, improving quality of life, and improving mortality rates in patients with heart failure.1–3 Technical advances and improvements in ablation strategy have improved the safety and efficacy of AF ablation over the past several decades. Pre- and intra-procedural ultrasound-based imaging has been utilized to improve procedural safety, especially transoesophageal echocardiogram to rule out the presence of left atrial thrombus and intracardiac echocardiogram (ICE) to guide transseptal puncture and monitor for complications. The use of advanced cardiac imaging modalities including computed tomography (CT) and magnetic resonance imaging (MRI) has also evolved from simple characterization of left atrial anatomy to identification of arrhythmogenic substrate and ablation effects. Pre-procedural imaging is capable of enhancing the ablation strategy and avoidance of procedural complications. While many questions remain about how best to use advanced imaging, it is clear that imaging has revolutionary potential for the field of AF ablation. In this review, we will discuss the history of imaging and future potential utilization for AF ablation.
Echocardiography
Echocardiography remains the dominant imaging modality in cardiology and has a critical role in the evaluation of patients prior to catheter ablation for AF. Transthoracic echocardiography is safe, inexpensive, and easily performed with an accurate estimation of cardiac anatomy, left ventricular function, chamber sizes, intracardiac pressure gradients, and valvular function. Transthoracic echocardiography, however, is limited in its ability to characterize the left atrial appendage (LAA) and transoesophageal echocardiography (TOE) is often necessary before catheter ablation of AF to rule out the presence of thrombus. More recently, ICE has also been shown to be effective at identifying thrombus, a fundamental contraindication to catheter ablation of AF.4 However, the ability of ICE to comprehensively view the entire appendage has not been systemically compared to TOE and its diagnostic indices must be further delineated.
Procedural transoesophageal echocardiogram (TOE) and ICE can effectively characterize the relationship between the pulmonary veins and the left atrium. Both modalities provide excellent visualization of these structures and can be used to guide ablation. While TOE is limited by the need for real-time assistance of a second proceduralist, ICE can be performed by the electrophysiologist simultaneous to performing the ablation. Furthermore, an ICE-guided approach can be used to spare radiation from standard fluoroscopic approaches, which have long been used to guide transseptal puncture and identify pulmonary vein anatomy.5 Intracardiac echocardiogram can also be used to continuously monitor for complications such as pericardial effusion during the procedure. While the use of ICE has become more prevalent particularly in the USA, TOE remains the standard approach in many centres to guide transseptal puncture. Despite the promise of CT and MRI that will be discussed in this review, echocardiography remains the foundation for pre and intra-procedural imaging and all patients should have at least a transthoracic echocardiogram performed prior to undergoing an AF ablation.
Anatomic characterization
Characterization of left atrial structure and function has been shown to predict the risk of cardioembolic and cardiovascular disease.6,7 In patients with AF, thorough three-dimensional imaging evaluation of the left atrium can provide significant benefit in pre-procedural planning. While left atrial size can be estimated with a transthoracic echocardiogram, three-dimensional evaluation of the chamber with CT or MRI provides a more accurate estimation of volume,8 which is a significant predictor of stroke risk as well as AF recurrence after ablation and may help guide ablation or anticoagulation strategy.9,10 While no definitive recommendations exist about ablation strategy based on left atrial size, additional ablation beyond pulmonary vein isolation (PVI) may be required to maintain sinus rhythm in these patients. The effects of left atrial maze ablation are partially attributable to the creation of discontinuities within atrial tissue to eliminate sufficient excitable mass from supporting simultaneously re-entering wavefronts. Therefore, it stands to reason that larger atrial myocardial mass would require more complex ablation strategies to create discontinuities in conduction. Additionally, anatomic characterization enables lesion titration in regions with excess wall thickness, adjacent adipose tissue containing ganglionated plexi, or neighbouring myocardial or vascular tissue (Figure 1). Furthermore, anatomic and functional tissue characterization via advanced imaging is likely to transform stratification methodologies to assess the need for oral anticoagulation. Such methodologies will also be important to assess the safety of oral anticoagulation discontinuation even with the maintenance of sinus rhythm, given the high risk of cardioembolic phenomenon associated with severe left atrial enlargement and dysfunction.10

(A)—Axial, (B)—coronal, and (C)—sagittal three-dimensional LGE-CMR image planes showing major anatomic associations with the left atrium. Ao, aorta; Br, bronchus; CMR, cardiac magnetic resonance; CS, coronary sinus; Eo, Esophagus; LA, left atrium; LGE, late gadolinium enhancement; LV, left ventricle; PA, right main pulmonary artery; RA, right atrium; RIPV, right inferior pulmonary vein; RSPV, right superior pulmonary vein.
Beyond size, three-dimensional imaging reveals substantial variation in morphology of the left atrial chamber especially the LAA and roof. The LAA is known to be critically important in the development of thrombus and subsequent cardioembolic risk.11 Several morphological variants have been well described and the shape, number of lobes, and size of the LAA have all been shown to correlate with embolic risk.12,13 While imaging evaluation of the LAA can certainly help rule out the presence of thrombus at the time of the study and predict stroke risk, careful attention to the ostium of the appendage also provides valuable information for catheter ablation. The ostium of the LAA has a critical relationship with the left pulmonary veins where a ridge marks the target for ablation lesions to be delivered during wide antral PVI.14 Identification of this ridge and its relationship to the pulmonary veins can be useful to guide ablation strategy in a region with anatomic variability and frequently limited catheter stability.15
The left atrial roof is of critical importance during AF ablation both during PVI and posterior wall isolation, where a roofline is performed with ablation lesions connecting the right and left superior pulmonary veins. Ablation on the left atrial roof is limited by several features including concern by operators of possible perforation, resulting in less contact force being applied.16 The roof tissue, posterior to the aortic root and LAA, is also relatively thicker than the rest of the atrial muscle making transmural lesion formation more challenging.17 It is therefore helpful that three-dimensional imaging can characterize roof morphology, which may be flat, concave, or convex and may contain pouches, limiting continuity of linear ablation and increasing the risk of impedance rises and steam-pop phenomenon.15,18 Pre-procedural identification of these anatomic variants by the operator may decrease the likelihood of complications.
Nuanced understanding of the anatomic relationship between the pulmonary veins and the left atrium is critical for successful ablation. While the critical relationship between the pulmonary veins and the left atrium can often be characterized intra-procedurally with ICE imaging and electroanatomic mapping, pre-procedural three-dimensional imaging can decrease the procedural variability and allow for pre-operative planning. The introduction of fast anatomical mapping and multipolar catheters has led to decreased utilization of image registration during AF ablation. It is notable, however, that identification of early small pulmonary vein branches is not ensured with fast anatomical mapping. Additionally, the smoothing algorithms of fast anatomic mapping software often create errors at the pulmonary vein ostia, which have to be ‘erased’. Without great attention to detail, such errors can direct lesions away from intended ostial targets and advanced image integration can mitigate such errors. Both CT and MRI can characterize the number of pulmonary veins in addition to their size, location, and geometry. Especially valuable is their ability to identify variant anatomy, such as accessory pulmonary veins, which can be the source of AF triggers and require isolation.19 The accessory veins most commonly arise near the right-sided pulmonary veins but can occur anywhere including the atrial roof.20 This variant anatomy must be understood by the operator to ensure that all veins are isolated. In addition, small or early branching veins may be identified, alerting the electrophysiologist to the risk of pulmonary vein stenosis when ablating near the ostium of these vessels.21
Beyond PVI, additional ablation is frequently required for patients with persistent AF or atypical atrial flutter. The development of flutter is associated with right and left atrial volume as assessed by CT or MRI.22 When atrial flutter occurs, it is often necessary to perform linear ablation such as a septal mitral line connecting the mitral annulus to the right superior pulmonary vein or laterally to the left inferior pulmonary vein, or posterior wall isolation with roof or floor lines between the right and left superior and inferior pulmonary veins, respectively. The critical anatomic relationships necessary to perform these procedures can be additionally understood with CT or MRI evaluation.
Substrate characterization
While substantial anatomic information about the left atrium can be obtained with pre-procedural imaging, much of the same information can be identified with careful fluoroscopic, ICE, and electroanatomic mapping evaluations. The critical value of imaging was established with the discovery that the left atrial substrate could be characterized by MRI. Initially, Peters et al.23 demonstrated that MRI could identify ablation lesions and subsequent scar with late gadolinium enhancement (LGE) following ablation. In addition to focal scar identified with LGE, T1 mapping, which quantifies contrast-enhanced T1 relaxation time, was shown to quantify the presence of diffuse fibrosis.24 These techniques, which were based on well-established strategies identifying focal and diffuse fibrosis in the left ventricle, are more challenging in the left atrium in part due to the relative thinness of left atrial tissue.
Electrophysiologists must be aware of the practical limitations in accurately assessing the left atrial myocardium in light of the wall thickness, which challenges the limits of current spatial resolution and signal to noise ratio. Other challenges include artefacts related to cardiac implantable devices, and motion artefact due to breathing or irregular rhythms both of which can be somewhat mitigated with established protocols.25,26 Accurate substrate assessment is particularly reliant on image quality and appropriate determination of inversion time. In addition, image intensity normalization is critical as MRI signal is increased by the presence of gadolinium retention in fibrotic tissue but is also affected by other parameters including dosing and timing of contrast, renal function, body size, and surface coil proximity.27,28 We have previously developed a standardized measure of image intensity, the image intensity ratio, to normalize the LGE intensity to the mean blood pool intensity, which allows for accurate assessment of abnormal substrate.27 An additional strategy, the calculation of standard scores or z-scores of myocardial intensity, recently utilized by our imaging laboratory is particularly useful because it (i) allows the calculation of the probability of an intensity occurring within the normalized myocardial intensity distribution and (ii) enables the comparison of two scores that are from different patients or from the same patient over different image acquisition times.29 These strategies have recently been employed in commercialized software and will likely make substrate identification more reliable. We implore the utilization of z-scores across the field so that results will be comparable across imaging laboratories. Additionally, standardization of imaging sequences across scanner platforms and close communication between electrophysiologists and imagers is critical to enhance the reliability and utility of left atrial substrate characterization. In summary, high-quality image acquisition along with additional respiratory gating and pulse sequence optimization is critical to characterize thinner muscular tissue in the setting of AF ablation.
Following the development of reliable strategies to characterize left atrial substrate, MRI evaluation of left atrial LGE was shown to predict successful ablation strategies with more extensive LGE predicting the need for more ablation than PVI alone and mild LGE predicting overall favourable ablation outcomes.30,31 In the Delayed-Enhancement MRI Determinant of Successful Radiofrequency Catheter Ablation of Atrial Fibrillation (DECAAF) study, prospective evaluation of left atrial LGE before ablation was highly associated with ablation outcomes with excessive fibrosis predicting a high likelihood of recurrent arrhythmia. Given generally suboptimal outcomes following first-time ablation for AF and the increasing understanding that different ablation strategies may be necessary for certain patients, these data provided critical insight into the potential value of pre-procedural MRI. A recent study has suggested that anatomic targeting of LGE detected gaps may provide an alternate strategy to treat recurrent arrhythmias following AF ablation.32 We have found this strategy to be a helpful adjunct to traditional mapping techniques, particularly because some regions with LGE and critical importance to tachycardia circuitry do not exhibit low-voltage (Figure 2). Such areas of mismatch are often found in regions with higher wall thickness where the presence of a scar is masked by the voltage of neighbouring healthy myocardium.

Anteroposterior (AP) and posteroanterior (PA) electroanatomic map and segmented colour-coded late gadolinium enhancement cardiac magnetic resonance (LGE-CMR) images from a patient with prior pulmonary vein isolation now undergoing atrial tachycardia mapping and ablation. Red regions on the electroanatomic map correspond to <0.1 mV and on LGE-CMR segmentation correspond to image intensity ratio (IIR) > 1.2. During atrial tachycardia, the shortest post pacing interval minus tachycardia cycle length was found in the septal mitral annulus and the arrhythmia terminated during a septal mitral line ablation. Notably, while there is general agreement between low-voltage and LGE regions, some mismatches exist, particularly in the anterior limbus of the septum and near the mitral annulus, where wall thickness is higher, and scar may not be as reliably identified with voltage mapping.
Stroke remains the most devastating complication of AF, and left atrial characteristics have previously been shown to be associated with stroke risk. While the atrial size and LAA morphology may be drivers of some of the cardioembolic risk, functional atrial myopathy likely predisposes to stasis and thrombus formation.33,34 With the ability to characterize left atrial substrate, left atrial LGE has also been shown to have a significant association with stroke risk in patients with AF.35 Left atrial LGE is also associated with stroke in patients with embolic stroke and no history of AF, which may reflect a causal pathway for embolism independent of AF or may reflect the often occult status of asymptomatic AF.36,37 Prospective studies are necessary to determine the role of MRI identified LGE in characterizing stroke prevention strategies beyond the well-established CHA2DS2-VASc score.38
Imaging-guided ablation
Although it is clear that PVI alone is an insufficient ablation strategy for many patients with AF, it is unknown what supplementary ablation to perform and in which patients to be more aggressive. The effect of additional empiric linear ablation or elimination of abnormal fractionated electrograms has not reliably improved outcomes.39 The association between LGE and ablation outcomes has led to an interest in the modification of abnormal left atrial substrate. Targeting electrophysiologically defined substrate has encouraging preliminary results.40,41 The DECAAF II study is currently underway to prospectively evaluate the role of targeting MRI defined LGE in patients with persistent AF undergoing first AF ablation (Clinical Trial NCT02529319). The results of this trial could substantially alter the conventional ablation strategy in patients with persistent AF, a population with disappointing outcomes, and no ablation strategy that provides clear benefit beyond PVI.
The physiological basis for ablation targeting pre-existing fibrosis is similar to that observed in ventricular arrhythmias. Following myocardial infarction, for example, infarcted tissue forms a scar made-up of electrically inert fibrotic tissue with interconnected myocardial fibrils that remain electrically active. This provides the heterogeneous myocardial tissue necessary for functional re-entry.42 Similarly, fibrotic atrial tissue is associated with delayed conduction velocity and predisposition to AF.43,44 We have shown that areas of fibrosis identified by LGE on MRI also demonstrate decreased local conduction velocity.45 In addition to slow conduction, areas of LGE in patients with no prior ablation have also been shown to be associated with abnormal local electrograms even in areas with normal measured voltage.29 Regions of normal voltage and abnormal fractionated electrograms may represent highly heterogeneous conduction particularly important in enhancing the initiation and maintenance of functional re-entry and perpetuating AF. Furthermore, such regions may be challenging to reliably identify during ablation with high-resolution multipolar mapping techniques focusing on areas of abnormal voltage. These results further suggest that MRI identified substrate and the areas of abnormal conduction it represents could be optimal targets for ablation in patients with pre-existing LGE. Importantly, we have also demonstrated that the image attenuation ratio derived from contrast-enhanced multidetector CT is associated with left atrial scar substrates. Due to its relative ease of acquisition, CT may provide a useful alternative to substrate identification in the setting of AF.46 An added advantage with CT is high sensitivity and specificity for the identification of fat deposits overlaying the left atrial epicardium. Such deposits not only contain ganglionated plexi as potential targets during AF ablation but are also associated with electrogram fractionation as electrophysiologic substrates for AF.47 This may be used to guide the ablation of ganglionated plexi for the purpose of autonomic neuromodulation, especially in patients with vagally mediated AF. Epicardial adipose tissue may also be associated with both inflammatory cytokines and fibrotic remodelling in patients with AF.48 Prospective evaluation is necessary to determine whether targeting these regions as identified by pre-procedural imaging is of benefit in the ablation of AF.
When utilizing MRI, it is important to clarify that left atrial LGE can occur both from pre-existing fibrosis reflecting atrial myopathy and from lesions delivered during ablation. We have shown that these two types of LGE are significantly different on MRI, with ablation lesion-induced LGE exhibiting a thinner wall profile and having a higher image intensity, which correlates to a higher scar density.49 This distinction is important as it highlights the ability of ablation in a region of pre-existing fibrosis to further modify the substrate, inactivating the remaining electrically active fibrils and render it entirely electrically inert so that it cannot participate in AF initiation or propagation.
Novel imaging-guided strategies
Several novel approaches utilizing imaging to guide the ablation of AF are under investigation. Recently, computational modelling of left atria characterized by MRI in patients with persistent AF was used to guide ablation strategy. In this study, Boyle et al.50 performed cardiac MRI on ten patients prior to catheter ablation of persistent AF and used a novel computational method to identify ablation targets based on regions of fibrosis that were anticipated to be critical to AF sustenance. The study was not designed to evaluate AF recurrence rates, and more data is needed; however, the concept is encouraging as the field looks for ways to improve procedural efficacy and identify critical ablation targets.
Numerous strategies have been investigated to prevent atrioesophageal fistula formation following AF ablation. Fistulas form due to the proximity of the targeted posterior left atrial tissue and the oesophagus, and resultant injury from lesion delivery. Fortunately, these complications are rare although no protective strategy has reliably prevented their development. Oesophageal temperature monitoring has become standard; however, no clear temperature safety threshold has been established and temperature may continue to rise after lesion termination due to thermal latency.51 Mechanical deflection of the oesophagus with transoesophageal echocardiogram probes, stylets, or pericardial balloons have also been attempted.52,53 It is noteworthy, however, that atrioesophageal fistulas have been reported even following surgical AF ablation with bipolar radiofrequency, despite lifting the pulmonary vein away from the oesophagus and focusing energy away from it.54 Such reports strongly suggest that the mechanism is at least partially attributable to post-ablation inflammation which secondarily involves the oesophagus. Therefore, delivery of lesions to left atrial foci distant from the oesophagus may provide optimal safety. We are now evaluating a novel technique using standard pre-procedural imaging localization of the oesophagus to target lesion delivery with maximal distance from the oesophagus (Figure 3).

Posteroanterior views from two patients that underwent pulmonary vein isolation guided by oesophageal image integration. The two images highlight significant variability in oesophageal location and lesion placement targeted to minimize oesophageal contact while isolating the veins. Studies to assess the safety and efficacy of such an approach are underway.
Ultimately, the value of advanced imaging will only be fully realized once (i) radiation and need for wearing lead protection are eliminated, (ii) volume changes and registration misalignments associated with historic images are mitigated, (iii) real-time oesophageal location in the setting of peristalsis is illuminated, and (iv) real-time feedback regarding lesion creation, arrhythmia substrate modification, and collateral injury is visualized. To achieve these goals, our group introduced the concept of real-time MRI for EP procedural guidance in 2008.55 The technology of real-time MRI in the EP lab has seen major advances over the past decade. Several centres have reported typical atrial flutter ablations under MRI guidance;56,57 however, significant improvement in work flow, noise, and image artefact control with suitable electrogram recordings, mapping, cardioversion, and ablation in the strong electromagnetic field will need to be resolved before its wide acceptance. We applaud academic and industry collaborations to advance the field of real-time MRI guidance and believe the motivation for its use will continue to enhance the utility of new mapping strategies and ablation energy sources.
Current techniques for acquisition and segmentation of computed tomography and magnetic resonance images for atrial fibrillation ablation
Integration of pre-acquired CT and MR images with electrogram data greatly enhances the visual understanding of complex electroanatomic relationships, with growing utility as AF ablation evolves to include the identification of scar substrates, non-pulmonary vein triggers, and epicardial pulmonary vein and posterior wall connections. Here, we review practical considerations for the selection of imaging mode and integration into the procedural workflow.
Cardiac CT has a substantially shorter acquisition time than cardiac MRI and may be the preferred alternative for claustrophobic patients and those with difficulty holding their breath. Typical acquisition times for detailed cardiac CT images are on the order of 15–20 s. In contrast, cardiac MRI requires several minute-long acquisitions that vary in length depending upon sequence settings and patient heart rate. Additionally, when evaluating chamber dimensions, pulmonary vein, coronary artery, and other vascular boundaries, cardiac CT provides superior spatial resolution. If utilizing CT, the relative timing of scan acquisition from the injection is critical to ensure adequate contrast concentration within the left atrium. Heart rate control (ideally less than 60 b.p.m.) and minimization of heart rate variability is critical to optimize image quality and minimize radiation. Breath-holding during CT image acquisition is necessary to reduce image artefact. Image artefact is also minimized with the use of advanced multidetector CT scanners. Tube modulation or ‘prospective triggering’ should be used to lower or turn off the tube current (and reduce radiation exposure) during phases that are unlikely to be used for image reconstruction. The optimal phase for the reconstruction of the image for AF ablation will be end diastolic, thus allowing improved image registration with electroanatomic mapping systems.
The primary advantage of cardiac MRI compared to CT, when preparing for AF ablation, is the lack of ionizing radiation. This issue is not insubstantial given the potential cumulative radiation exposure to the patient. Additionally, the experience with and the resolution of cardiac MRI for imaging myocardial scar as a substrate for arrhythmia far surpasses that with cardiac CT, and cardiac MRI is capable of multi-planar imaging whereas three-dimensional reconstruction and volume rendering are necessary to visualize unconventional planes when using CT. Finally, patients with renal insufficiency, history of multiple myeloma, and risk of contrast-induced nephropathy, recent radioactive iodine therapy and hyperthyroidism would be better served with cardiac MRI and gadolinium contrast vs. iodinated CT contrast. When performing cardiac MRI in patients with AF, sub-optimal images can be obtained due to difficulties with electrocardiogram gating and respiratory motion. The use of single-shot images may optimize results but the primary sequences for AF procedures are MR angiography to visualize the end-diastolic dimensions of cardiac chambers and vascular structures of interest, and LGE inversion recovery sequences to visualize scar. Our preferential technique for MR angiography is time-resolved angiography where accurate timing of contrast bolus arrival is not essential. Atrial LGE imaging requires specialized software for respiratory gating and variations in pulse sequences to visualize the thinner muscular tissue. Such a pulse sequence may not be readily available at all centres and is not routinely performed unless requested. When available, such images allow the integration of pre-existing atrial scar into the procedural space.
Once adequate images are acquired, segmentation must be performed to extract important features for import into the electroanatomic mapping platform. Current mapping systems employ built-in segmentation software for typical CT and MR images. However, standalone software (Argus, SEGMENT, MASS, Matlab, etc.) can also be utilized to segment not only relevant anatomy but also LGE, as summarized for a representative case in Figure 4 using ADAS 3D software (ADAS3D Medical). When segmenting the left atrium, particular attention must be made to contouring the appropriate myocardial borders. We find this process to be greatly enhanced by transferring contours from MR angiography or T2-weighted black blood images, which accentuate left atrial myocardial borders. Additionally, particular attention must be paid to the normalization of LGE intensity as discussed above to accurately identify abnormal substrate. Deep learning has recently emerged as a promising technique to aid image reconstruction although refinement of these techniques is necessary before application in clinical practice.
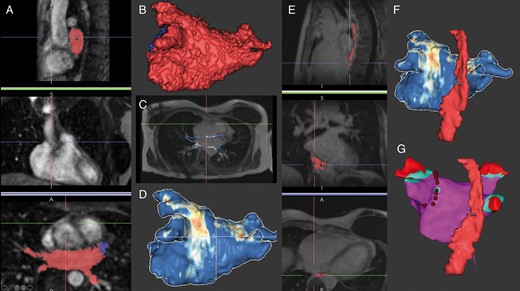
The process of MR image segmentation using ADAS 3D software (ADAS3D Medical) for integration during AF ablation. (A) Sagittal, coronal, and axial MR angiography images with semi-automatic segmentation of the left atrium using an intensity threshold (red mask: left atrium, blue mask: LAA) and resulting in the left atrial segmentation in (B). In (C), the contour is applied to the LGE image and normalized intensity measurements of the left atrial myocardium are colour coded to produce the anatomic and scar model segmentation in (D). (E) Manual oesophageal segmentation in sagittal, coronal, and axial planes resulting in the three-dimensional model in (F). Ultimately, the segmented model is imported into the electroanatomic mapping system and registered to procedural coordinates from ultrasound or fast anatomic mapping chamber boundaries. In (G), the left atrial voltage map shows healthy tissue in purple, voltage <0.2 mV in blue, <0.1 mV in red, radiofrequency lesions surrounding the left sided pulmonary veins as red dots, and the oesophagus anatomy as registered from the MR image. LAA, left atrial appendage, LGE, late gadolinium enhancement; MR, magnetic resonance.
Conclusion
Advanced imaging modalities such as cardiac CT and MRI provide valuable information to guide the management of patients with AF. Traditionally, these three-dimensional imaging techniques were utilized to identify anatomy with a focus on identifying abnormal variants and preventing procedural complications. More recently, the ability to characterize abnormal left atrial substrate has been demonstrated. While this requires imagers to overcome substantial technological obstacles, there is reason for optimism that substrate evaluation and real-time MRI guidance may ultimately enhance procedural strategy and safety, improving ablation outcomes for patients with AF.
Conflict of interest: S.N. is a consultant to Siemens, CardioSolv, and Circle Software and principal investigator for research funding to the University of Pennsylvania from Biosense-Webster, Siemens, ImriCor, and the National Institutes of Health. M.K. and T.M.M. report no relevant financial disclosures.
Authors
Timothy M. Markman, MD – Dr Markman received a Doctorate in Medicine from the Johns Hopkins University School of Medicine. He completed residency in the Osler Internal Medicine Training Program at the Johns Hopkins Hospital and subsequently completed fellowship training at the University of Pennsylvania in Cardiovascular Disease. Currently, he is a fellow in Clinical Cardiac Electrophysiology at the University of Pennsylvania. His research focuses on the role of the autonomic nervous system in arrhythmia with the goal of developing novel treatment strategies.
Saman Nazarian, MD, PhD – Dr Nazarian received a Doctorate in Medicine from Stanford University and PhD in Clinical Epidemiology from the Johns Hopkins Bloomberg School of Public Health. He completed residency at Harvard University’s Brigham and Women’s Hospital, followed by fellowship training in Cardiovascular Disease and Clinical Cardiac Electrophysiology at the Johns Hopkins Hospital. After a decade on the faculty at Johns Hopkins University, he joined the faculty at the University of Pennsylvania Perelman School of Medicine where he continues his clinical cardiac electrophysiology practice and is a faculty investigator and educator. Dr Nazarian runs an active clinical research program, funded by the US National Institutes of Health, with the goal of improving treatment strategies to reduce cardiac arrhythmia related morbidity and mortality.
Mirmilad Khoshnab, MD – Dr Khoshknab received a Doctorate in Medicine from the Azad Tabriz University School of Medicine. He then completed a post-doctoral research fellowship at Johns Hopkins University. Currently, he is a research fellow in Cardiac Electrophysiology at the University of Pennsylvania Perelman School of Medicine. His research focuses on advanced imaging techniques and image processing for cardiac electrophysiology interventions related to atrial fibrillation and ventricular tachycardia.
References
Authors
Biography: Timothy M. Markman, MD – Dr Markman received a Doctorate in Medicine from the Johns Hopkins University School of Medicine. He completed residency in the Osler Internal Medicine Training Program at the Johns Hopkins Hospital and subsequently completed fellowship training at the University of Pennsylvania in Cardiovascular Disease. Currently, he is a fellow in Clinical Cardiac Electrophysiology at the University of Pennsylvania. His research focuses on the role of the autonomic nervous system in arrhythmia with the goal of developing novel treatment strategies.
Biography: Mirmilad Khoshnab, MD – Dr Khoshknab received a Doctorate in Medicine from the Azad Tabriz University School of Medicine. He then completed a post-doctoral research fellowship at Johns Hopkins University. Currently, he is a research fellow in Cardiac Electrophysiology at the University of Pennsylvania Perelman School of Medicine. His research focuses on advanced imaging techniques and image processing for cardiac electrophysiology interventions related to atrial fibrillation and ventricular tachycardia.
Biography: Saman Nazarian, MD, PhD – Dr Nazarian received a Doctorate in Medicine from Stanford University and PhD in Clinical Epidemiology from the Johns Hopkins Bloomberg School of Public Health. He completed residency at Harvard University s Brigham and Women s Hospital, followed by fellowship training in Cardiovascular Disease and Clinical Cardiac Electrophysiology at the Johns Hopkins Hospital. After a decade on the faculty at Johns Hopkins University, he joined the faculty at the University of Pennsylvania Perelman School of Medicine where he continues his clinical cardiac electrophysiology practice and is a faculty investigator and educator. Dr Nazarian runs an active clinical research program, funded by the US National Institutes of Health, with the goal of improving treatment strategies to reduce cardiac arrhythmia related morbidity and mortality.