-
PDF
- Split View
-
Views
-
Cite
Cite
Piergiuseppe Agostoni, Michele Emdin, Fabiana De Martino, Anna Apostolo, Marco Masè, Mauro Contini, Cosimo Carriere, Carlo Vignati, Gianfranco Sinagra, Roles of periodic breathing and isocapnic buffering period during exercise in heart failure, European Journal of Preventive Cardiology, Volume 27, Issue 2_suppl, 1 December 2020, Pages 19–26, https://doi.org/10.1177/2047487320952029
- Share Icon Share
Abstract
In heart failure, exercise – induced periodic breathing and end tidal carbon dioxide pressure value during the isocapnic buffering period are two features identified at cardiopulmonary exercise testing strictly related to sympathetic activation. In the present review we analysed the physiology behind periodic breathing and the isocapnic buffering period and present the relevant prognostic value of both periodic breathing and the presence/absence of the identifiable isocapnic buffering period.
Introduction
In heart failure (HF), exercise-induced periodic breathing (PB) – also known as oscillatory exercise ventilation (EOV) – is defined by an oscillatory pattern of ventilation which can last for the entire exercise or for a part of it. The isocapnic buffering period is the period of exercise between the anaerobic threshold and the respiratory compensation point (Figure 1). It is the part of exercise during which lactic acid is buffered by bicarbonates and pH is maintained. Its length depends on two factors: the amount of available carbon dioxide (CO2) and the threshold of arterial carbon dioxide tension (PaCO2) needed to increase ventilation by chemoreceptors. PB and the isocapnic buffering period are two features of ventilation during exercise highly dependent on chemoreflex regulation. Both carry relevant information on HF severity and prognosis. The presence of PB and of the isocapnic buffering period are mutually exclusive, but both can be absent. PB is related to an imbalance among circulation, muscle function and chemoreflex response, while end tidal carbon dioxide pressure (PetCO2) or, when measured, arterial partial pressure carbon dioxide (PaCO2), during the isocapnic buffering period are mirrors of the sympathetic activity, and more specifically of the chemoreflex activity. The presence of PB suggests low cardiac output, limited CO2 reserve and sympathetic overactivation; a low PetCO2 during the isocapnic buffering period also suggests sympathetic overactivation, while buffering capacity, which translates into the amount of oxygen consumption (VO2) during the isocapnic buffering period, is directly related to exercise performance. In a maximal exercise, the absence of the isocapnic buffering period is indicative of reduced exercise performance and hyperventilation, either voluntary or related to environmental conditions, such as high altitude, or to specific disease, such as pulmonary hypertension.1,,–4
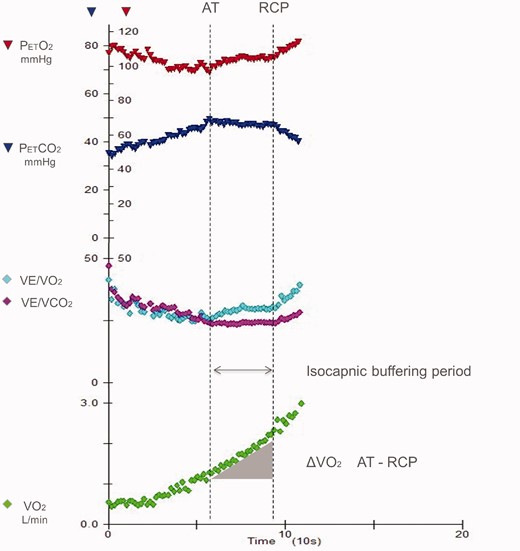
Ventilation, oxygen consumption (VO2) and minute ventilation (VE)/carbon dioxide production (VCO2) and derived parameter changes during exercise. The isocapnic buffering period is identified between the anaerobic threshold (AT) and the respiratory compensation point (RCP). Reproduced from Carriere et al.17 with permission.
PB is characterised by cyclic fluctuations of minute ventilation (VE), tidal volume, VO2, carbon dioxide production (VCO2), PetCO2 and end tidal oxygen pressure (PetO2). PB may be either persistent along the entire exercise or present only in its early phases, usually up to the anaerobic threshold. PB is currently described in terms of amplitude and interval (length) of the single VE oscillation, and of the duration of the abnormal VE phenomenon during exercise, but a wide variability of PB patterns has been reported. To diagnose and describe PB, several descriptors have been utilised. With regard to cycle length, the following have been reported: (a) the distance between two nadirs; (b) the period of observed oscillation divided by the number of oscillations; and (c) the mean value of the interpeak interval for each cycle at rest.5 The amplitude of the VE oscillations is calculated as: (a) the difference between the peak of the oscillation of VE and the average of the VE of the two surrounding nadirs; (b) the variation coefficient; (c) the correlation coefficient; and (d) a percentage of the mean of the rest VE.5 For the definition of PB presence, a duration of at least two-thirds of the entire exercise protocol, or 60% or greater, or 50% or greater have been proposed.5 Therefore, no gold standard exists so far, and an accepted PB definition is still lacking, which makes comparisons between studies difficult.6 Of note, the cardiopulmonary exercise testing (CPET) data averaging system used may cancel the evidence of PB. Thus, whenever the presence of PB is suspected, breath-by-breath data evaluation is needed as the first step of CPET analysis. With regard to PB, one of the major unsolved questions is what oscillates first between ventilation and circulation; in other words, whether it is ventilation or circulation that leads to oscillation. A few pieces of evidence suggest that oscillation in the blood flow is the first initiating mechanism. Pioneering studies by Ben Dov et al.7 have demonstrated that, when aligning VO2, VCO2 and VE, VO2 oscillation anticipates VCO2 and VE oscillations. Of note, oscillation of muscle blood flow can be observed in PB patients by means of near infrared spectroscopy in the skeletal muscle.8Figure 2 shows oscillation of total haemoglobin, oxygenated haemoglobin (O2Hb) and deoxygenated haemoglobin (desatHb) at rest in a patient with severe HF. Of note, desatHb oscillation anticipates that of O2Hb. Two physiological explanations for the observed intramuscular haemoglobin behaviour can be advanced, which are not mutually exclusive but are likely both to be present. The first is that phasic oscillation of haemoglobin and its components O2Hb and desatHb is due to cardiac output oscillation, and the second is that haemoglobin desaturation increases local nitric oxide and therefore the observed haemoglobin changes are due, at least in part, to cycling of muscle capacitance vessels. Of note, the period (length) of cycling of haemoglobin in the muscles seems to be greater than that observed in VE.
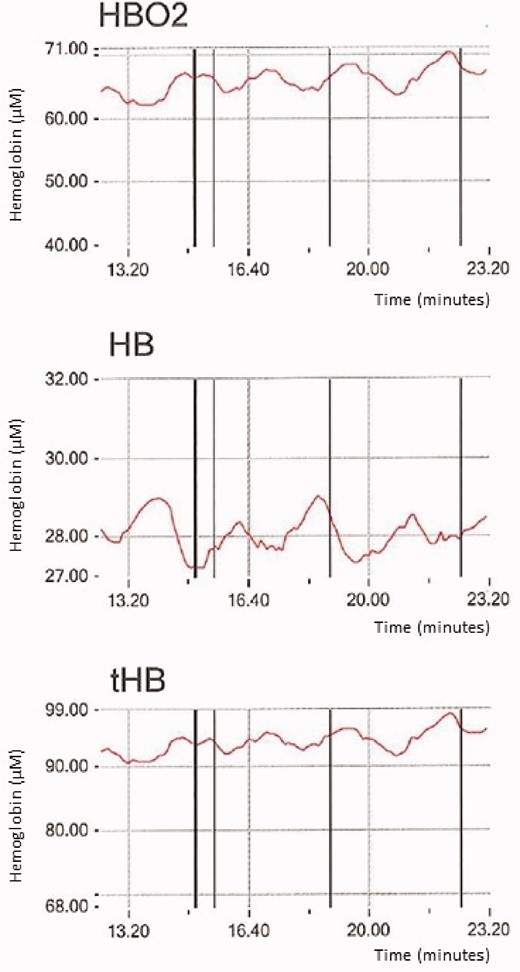
Measurements of near infrared spectroscopy (NIRS) data at rest in a patient with periodic breathing at rest. Fluctuation of oxygenated haemoglobin (HBO2), de-oxygenated haemoglobin (HB) and total haemoglobin (tHB) are reported. The cycle length is approximately 130 seconds.
Exercise-induced periodic breathing and low PetCO2 during isocapnic buffering period in severe HF
A few data are available on the prevalence of exercise-induced PB in patients with HF.9,,–12 In 7004 systolic HF patients from the MECKI score dataset,13,14 PB was present in 16.8% of cases, and mainly in those with the most impaired exercise capacity. In particular, PB was observed in 25.2% of patients with peak VO2 less than 12 ml/min/kg, 16.4% of patients with peak VO2 ranging between 12 and 16 ml/min/kg and 9.6% of those with peak VO2 greater than 16 ml/min/kg. Of note, there is a gender-specific PB behaviour, with a lower number of women with PB compared to men, regardless of HF severity. With regard to the total MECKI score population, at present 7004 cases, PB is present in 13% of female and 18% of male patients. A similar gender-specific PB incidence was reported in HF patients during sleep and in normal subjects at high altitude.9,15,16 There is no accepted explanation for this phenomenon, although differences in peripheral muscle CO2 production, in apnoeic CO2 threshold or in the chemoreflex response to partial pressure oxygen (pO2) and pCO2, probably related to the effect of sex hormones, are possibly responsible for the observed gender differences.
The isocapnic buffering period was identified in 39% of cases in a sizable population (n = 1995) derived from the MECKI score dataset by Carriere et al.17 HF patients with an identified respiratory compensation point (601 out of 1896 cases) were grouped according to peak VO2. PetCO2 during the isocapnic buffering period was lower in patients with more severe HF, and increased as functional capacity improved: lower peak VO2 tertile (n = 110, peak VO2 10.9 ± 1.7 ml/min/kg, PetCO2 during isocapnic buffering period 33.0 ± 4.6 mmHg); middle peak VO2 tertile (n = 222, peak VO2 15.0 ± 1.2 ml/min/kg, PetCO2 during isocapnic buffering period 35.0 ± 4.2 mmHg); higher peak VO2 tertile (n = 269, peak VO2 21.0 ± 3.6 ml/min/kg, PetCO2 during isocapnic buffering period 37.5 ± 4.9 mmHg, P<0.0001). These data speak in favour of a progressively increasing chemoreflex activity in parallel with increasing HF severity.
Physiology of exercise-induced periodic breathing in HF and of the isocapnic buffering period
Little is known about the physiology of exercise-induced PB. Three factors play a pivotal role in exercise-induced PB in HF: hyperventilation, low cardiac output leading to increased circulatory delay, and cerebrovascular reactivity to CO2.18 Other possible poorly explored factors influencing PB in HF are: the relation between PB and Cheyne–Stokes respiration (CSR) at rest,9,10 as well as the effect of posture, which has recently been shown to influence the presence of CSR at rest (a hypothesis which deserves further investigation could be that only those patients having CSR when upright would develop PB).19
Hyperventilation is part of the increased sympathetic activity frequently observed in HF, and it is due to the increased stimulation of intrapulmonary receptors and of chemo and metaboreceptors.20 Due to hyperventilation, PaCO2 falls below the respiratory threshold, so that breath stops due to temporary absence of central drive to respiratory muscles, which ends when an increase of PaCO2 promotes ventilation with a further period of hyperventilation. Moreover, HF patients have a reduced cerebrovascular reactivity to CO2 further increasing breathing instability.18 The increased circulatory delay, which is due to a low cardiac output, is considered another factor responsible for the occurrence of exercise-induced PB. Indeed, circulatory delay leads to a temporary misalignment of the chemical signals (low or high PaCO2) to the respiratory response (increased or reduced ventilation).18,21 Notably, albeit frequently suggested, the role of low cardiac output in the pathogenesis of exercise-induced PB presently has almost no scientific evidence. Indeed, no significant differences in cardiac output or in the circulatory delay from lungs to peripheral receptors have been found between HF patients with and without exercise-induced PB.22,23 Similarly, no correlation exists between cardiac output and circulatory delay at rest in patients with severe HF. However, there are no data on the relationship between circulatory delay and cardiac output during physical activity. In a recent series of HF patients who successfully underwent implantation of a left ventricular assist device (LVAD),24 resting cardiac output and circulatory delay were found to be unrelated to each other both at low LVAD pump speed and at high pump speed; of note, these data were obtained at rest and no information is available during exercise. Interestingly, in a group of 15 HF patients with LVAD, exercise-induced PB occurred in nine cases with LVAD at pump speed 2 and in five patients at pump speed 4. Moreover, the presence of exercise-induced PB in this series of patients was associated with a lower cardiac output at rest. A reduction in exercise-induced PB due to HF improvement was reported by Ribeiro et al., who showed that exercise-induced PB disappeared after HF improvement in a series of five patients.25 Another observation about PB whose cause is still undefined is that, in approximately 60% of cases, PB disappears during a progressive effort before exhaustion. Among the three main causes of PB – hyperventilation, low cardiac output and cerebrovascular reactivity to CO2 – low cardiac output is the only one that should change during exercise, shortening the delay of the signal between alveoli and carotid chemoreceptors. It must be recognised that this is pure speculation, albeit physiologically plausible. Of note, Schmid et al. showed that, in 52% of patients in whom exercise-induced PB ceased during exercise; that is, before the end of exercise, in the last part of exercise when ventilation cycling ceased the VO2 versus work relationship increased, it was still in 24% of cases, and it decreased in 24% of cases.26 Notably, the evaluation of the VO2 versus work relationship allows an understanding of the amount of O2 delivery to the working muscle, because a reduction of this relationship during exercise is suggestive of a reduction of cardiac output, either due to exercise-induced cardiac ischaemia or to mitral insufficiency.27,28 An increase in the VO2 versus work relationship during exercise is a rare event, and it was only reported in patients in whom PB ceased during exercise, specifically in approximately 50% of cases. This implies a higher O2 delivery to the working muscles, possibly due to a reduction of the work of breathing. In other words, PB seems to be associated with an increased work of breathing, and its disappearance allows an increase of VO2 delivery to the exercising muscles so that external work increases. Other aspects of PB physiology are of interest.
For example, there are several pieces of evidence that exercise-induced PB can be eliminated inducing hyperventilation by adding a further ventilatory stimulus such as hypoxia, inhalation of CO2, or dead space increase. Indeed, if a hypoxic stimulus is suddenly added during constant low workload exercise, PB disappears. Similarly, Apostolo et al.29 showed that, when 2% CO2 is inhaled, PB suddenly ceases. However, if the added respiratory stimulus is smaller, as with 1% CO2 instead of 2% CO2, periodic breathing persists. Finally, when an external dead space is added, PB disappears early during exercise or it does not appear at all, according to the amount of added dead space, 250 or 500 ml, respectively.30 However, added dead space is associated with a lower exercise performance probably due to a further increase of the work of breathing.
Similarly, the physiology of the isocapnic buffering period has been studied but rarely considered in the clinical field. The absence of isocapnic buffering has a strong negative prognostic power. In a recent analysis on patients belonging to the MECKI score dataset, Carriere et al.17 also showed that the amount of VO2 during the isocapnic buffering period is directly related to exercise performance in HF patients. Accordingly, a high VO2 increase during the isocapnic buffering period implies a limited CO2 production due to lactic acid buffering, but also a relevant amount of bicarbonates for H+ buffering. The value of PetCO2 during the isocapnic buffering period reveals chemoreflex activation – the lower the PetCO2, the higher the reflex activation. Of note, in HF, PetCO2 levels during the isocapnic buffering period can be modulated by β-blockers. In particular, β1–β2 blockers cause a higher PetCO2 during the isocapnic buffering period by reducing chemoreflex activity.31,32
Prognostic role of exercise-induced periodic breathing and of the isocapnic buffering period
PB is a robust CPET risk index in distinct HF cohorts,9,10,12,33,,,,–38 including heart transplantation candidates, and in patients chronically treated with β-blockers. PB and several CPET-derived parameters have proved to be predictive in HF,39 but most have been studied in binary analyses, considering risk indexes in isolation, disregarding the potential value of combining variables. In addition, few observations have been validated yet. So far, only peak VO2, VE/VCO2 slope, and PB (representing the so-called 2008 European Society of Cardiology (ESC) model) have been validated.40 Hence, albeit difficult to compute properly, PB should always be taken into account during CPET, if macroscopically evident at visual or analytical inspection. Recently, Rovai et al.41 re-analysed the MECKI score database and not only confirmed a relevant prognostic role of PB in HF patients but also showed that PB was equally present in patients with heart failure with reduced ejection fraction (HFrEF) and heart failure with mid-range ejection fraction (HFmrEF). Of note, PB maintained its negative prognostic role in HFmrEF patients, albeit only after 18 months of follow-up. Indeed, for short follow-up periods, no prognostic differences between HFmrEF patients with and without PB were observed (Figure 3).41 In brief, PB should be considered as an ominous sign in HF.
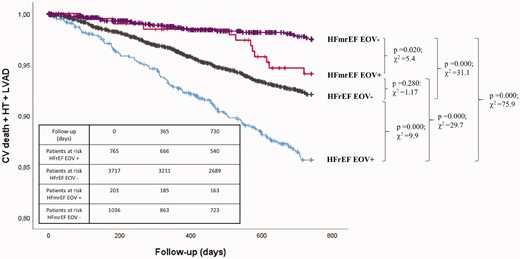
Survival and presence of exercise oscillatory ventilation in patients with heart failure with reduced ejection fraction (HFrEF) and in patients with heart failure with mid-range ejection fraction (HFmrEF). Kaplan–Meier survival curves of study endpoint (cardiovascular death, urgent heart transplant or left ventricular assist device (LVAD) implantation) stratified according to the presence or absence of exercise oscillatory ventilation (EOV+ and EOV–) in patients with HFrEF and in patients with HFmrEF. Comparison among the groups (HFmrEF EOV– vs. HFmrEF EOV+: P = 0.020, χ2 = 5.4; HFmrEF EOV– vs. HFrEF EOV–: P = 0.000, χ2 = 31.1; HFmrEF EOV– vs. HFrEF EOV+: P = 0.000; χ2 = 75.9; HFmrEF EOV+ vs. HFrEF EOV–: P = 0.280, χ2 = 1.17; HFmrEF EOV+ vs. HFrEF EOV+: P = 0.000, χ2 = 29.7; HFrEF EOV– vs. HFrEF EOV+: P = 0.000; χ2 = 9.9). Reproduced from Rovai et al.41 with permission.
Limited information is available on the prognostic role of the isocapnic buffering period and on measurements obtained during the isocapnic buffering period. Indeed, in an analysis of the MECKI score dataset, Carriere et al.42 showed relevant differences in prognosis in subjects in whom the anaerobic threshold and the respiratory compensation point were not identified (Figure 4). This suggests that the persistence of a normal exercise physiology bears a relevant prognostic impact regardless of the values of VO2, VCO2, or VE. No data are presently available on the prognostic role of PetCO2 during the isocapnic buffering period. However, PetCO2 during the isocapnic buffering period is directly associated with peak VO2. Therefore, its prognostic power should simply be a confirmatory observation, which has the major advantage of being observed even if the true peak VO2 has not been reached.
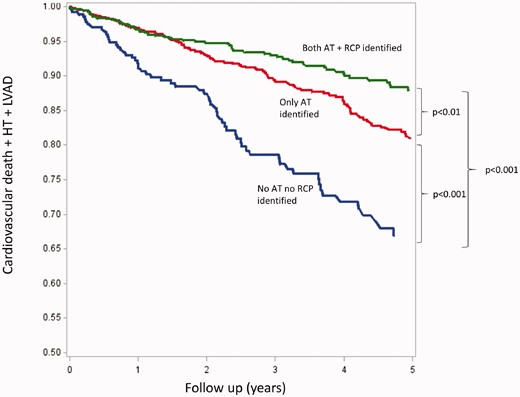
Five-year survival, assessed by the composite of cardiovascular death, urgent heart transplantation (HT) and left ventricular assist device (LVAD) in groups 1 (neither anaerobic threshold (AT) nor respiratory compensation point (RCP) detectable), 2 (detectable AT but no RCP) and 3 (both AT and RCP detectable), respectively. Reproduced from Carriere et al.42 with permission.
Conclusions
Exercise-induced PB and the analysis of the respiratory compensation point both bear relevant information on ventilation during exercise and both deserve attention when interpreting a CPET. Indeed, both show data associated with prognosis and may provide information useful to guide HF treatment or LVAD set-up. More studies are needed to assess these specific ventilatory patterns in specific sets of HF patients as well as the effects of therapy on them.
PA, ME and GS contributed to the conception or design of the work. PA and GS drafted the manuscript. ME, FDM, AA, MM, MC and CC critically revised the manuscript. All authors gave final approval and agree to be accountable for all aspects of the work ensuring integrity and accuracy.
The author(s) declared no potential conflicts of interest with respect to the research, authorship, and/or publication of this article.
The author(s) received no financial support for the research, authorship, and/or publication of this article.
References
Vignati C, Apostolo A, Cattadori G, et al. Lvad pump speed increase is associated with increased peak exercise cardiac output and vo2, postponed anaerobic threshold and improved ventilatory efficiency. Int J Cardiol 2017; 230: 28–32.
Comments