-
PDF
- Split View
-
Views
-
Cite
Cite
Charalambos Antoniades, Dimitris Tousoulis, Marija Vavlukis, Ingrid Fleming, Dirk J Duncker, Etto Eringa, Olivia Manfrini, Alexios S Antonopoulos, Evangelos Oikonomou, Teresa Padró, Danijela Trifunovic-Zamaklar, Giuseppe De Luca, Tomasz Guzik, Edina Cenko, Ana Djordjevic-Dikic, Filippo Crea, Perivascular adipose tissue as a source of therapeutic targets and clinical biomarkers: A clinical consensus statement from the European Society of Cardiology Working Group on Coronary Pathophysiology and Micro-circulation, European Heart Journal, Volume 44, Issue 38, 7 October 2023, Pages 3827–3844, https://doi.org/10.1093/eurheartj/ehad484
- Share Icon Share
Abstract
Obesity is a modifiable cardiovascular risk factor, but adipose tissue (AT) depots in humans are anatomically, histologically, and functionally heterogeneous. For example, visceral AT is a pro-atherogenic secretory AT depot, while subcutaneous AT represents a more classical energy storage depot. Perivascular adipose tissue (PVAT) regulates vascular biology via paracrine cross-talk signals. In this position paper, the state-of-the-art knowledge of various AT depots is reviewed providing a consensus definition of PVAT around the coronary arteries, as the AT surrounding the artery up to a distance from its outer wall equal to the luminal diameter of the artery. Special focus is given to the interactions between PVAT and the vascular wall that render PVAT a potential therapeutic target in cardiovascular diseases. This Clinical Consensus Statement also discusses the role of PVAT as a clinically relevant source of diagnostic and prognostic biomarkers of vascular function, which may guide precision medicine in atherosclerosis, hypertension, heart failure, and other cardiovascular diseases. In this article, its role as a ‘biosensor’ of vascular inflammation is highlighted with description of recent imaging technologies that visualize PVAT in clinical practice, allowing non-invasive quantification of coronary inflammation and the related residual cardiovascular inflammatory risk, guiding deployment of therapeutic interventions. Finally, the current and future clinical applicability of artificial intelligence and machine learning technologies is reviewed that integrate PVAT information into prognostic models to provide clinically meaningful information in primary and secondary prevention.

Perivascular adipose tissue (PVAT) as a source of imaging biomarkers and a therapeutic target. FAI, fat attenuation index.
Introduction
Obesity is a widely accepted risk factor for cardiovascular disease, as increased body mass index (BMI) has been associated not only with classic cardiovascular risk factors like type 2 diabetes mellitus, hypertension, and hyperlipidaemia but also with coronary artery disease, heart failure, atrial fibrillation, and stroke, independently of other risk factors.1–3 This was initially believed to be due to the detrimental effects of adipose tissue (AT)-derived molecules (e.g. adipokines) on the human arterial wall and heart.1 However, during the last decade, it has become clear that the association between obesity and cardiovascular disease is much more complex than originally anticipated. Epidemiological studies have shown a U-shaped relationship between BMI and survival, with higher short- and mid-term survival rates observed among overweight individuals (BMI 25–30 kg/m2), while ‘normal’ body weight (BMI 20–24.9 kg/m2) only related with better long-term survival (>15 years).2 This paradoxically ‘protective’ effect of obesity was more evident in individuals with chronic diseases, including heart failure, renal failure, or cancer.4 This ‘obesity paradox’ was originally explained by the inability of BMI to capture fat distribution in the body, since visceral obesity was felt to be particularly detrimental, while gluteal/subcutaneous fat has a neutral effect on cardiovascular risk prediction. Visceral obesity was therefore defined as metabolically unhealthy obesity, while gluteal obesity was defined as metabolically healthy obesity.5 However, the most important lesson to be learned from studying the ‘obesity paradox’ was that AT may actually sense systemic signals of cachexia related to chronic diseases, the latter would initiate lipolysis, a phenomenon driving weight loss in chronic conditions like cancer or heart failure, leading to misinterpretations of the relationship between body fat mass and survival.4 These considerations imply that AT can be used as a biosensor of the severity of chronic diseases, turning it into a potential source of diagnostic or prognostic biomarkers. Therefore, understanding the biological nature of the cross-talk between AT and the cardiovascular system is an unmet need, that will almost certainly lead to the discovery of new therapeutic targets and the development of novel diagnostic biomarkers in cardiovascular medicine.
Defining the different adipose tissue depots: consensus terminology
Human AT is classified into three main types: white (WAT, responsible for energy storage), brown (BAT, responsible for ‘shivering thermogenesis’), and beige (that includes adipocytes with intermediate phenotype between that of white and brown), and is distributed in the human body, as shown in Figure 1.6,7

Imaging of human adipose tissue depots by computed tomography. Axial (A), coronal (B), and sagittal (C) views of the chest showing subcutaneous, visceral abdominal, thoracic (including the pericardial and epicardial) adipose tissue. Reconstruction of perivascular adipose tissue (PVAT) around an epicardial coronary artery (D) and thoracic aorta (E). PVAT is defined as the adipose tissue lying within a radial distance from the outer vessel wall equal to the vessel diameter (or at a maximum distance of 2 cm in the case of large vessels with diameter >2 cm, like the aorta).
Anatomically, WAT can be sub-divided into visceral adipose tissue (VAT) and subcutaneous adipose tissue (SAT). SAT is located within the reticular dermis of the skin. In males, SAT is mainly located over the trunk, whilst in females is predominantly distributed around the hips and lower limbs.7 Abdominal SAT is sub-classified as superficial and deep SAT layers, the latter being phenotypically closer to VAT.7
Visceral adipose tissue includes the abdominal and thoracic AT. Abdominal VAT is located within the abdominal cavity, surrounding the visceral organs, and it is further sub-divided into mesenteric peritoneal and retroperitoneal (perirenal) AT. Thoracic VAT includes the epicardial AT (EAT), which is enclosed between the cardiac surface and the visceral pericardium, the pericardial AT (external to the pericardium and surrounding the cardiac silhouette), as well as the non-pericardial thoracic AT (located anywhere inside the thoracic cavity, but outside the pericardium; this includes and the pericardial depot) (Figure 1).8 Perivascular AT (PVAT)9–11 around the coronary arteries has distinct biological properties compared to the rest of EAT, far from the arterial wall.12 However, as there is gradual transition between PVAT and non-PVAT within EAT, without any anatomical structure separating the two, the definitions need to be agreed on. Indeed, in the recent literature,13–19 PVAT is defined as the layer of AT located within a distance equal to the luminal diameter of the artery, a definition adopted by the working group.10 This definition applies to the PVAT surrounding any artery in the human body up to a luminal diameter of 2 cm (see Figure 1). For arteries with luminal diameter >2 cm (such as the aorta), PVAT extents up to 2 cm from the outer surface of the vessel.
Biological differences between adipose tissue depots
The nomenclature of human AT depots does not only serve the purposes of the anatomical classification, but also highlights the important phenotypic and biological differences between AT depots (Table 1). For example, VAT contains smaller, less differentiated adipocytes with significantly higher infiltration by macrophages compared to the subcutaneous depot.20 VAT is highly active in terms of adipokine secretion, and its secretome closely correlates with systemic metabolic status and insulin resistance. In contrast, SAT contains larger, well-differentiated adipocytes and is less active in terms of adipokine secretion.21 Peri-vascular adipose tissue has a unique role in the physiology of the cardiovascular system, given its anatomical proximity to the vascular wall (Table 2). In this article, we will focus on the role of PVAT in cardiovascular physiology and will discuss its role as a therapeutic target and/or as a source of diagnostic biomarkers in clinical practice.
Subcutaneous adipose tissue (SAT): in the hypodermis (i.e. just below the skin); may include the subcutaneous thoracic, abdominal, gluteal, or femoral adipose tissue |
Visceral adipose tissue (VAT): the adipose tissue located inside the abdominal or thoracic cavity, between the viscera; sub-classified into thoracic adipose tissue (pericardial, non-pericardial, epicardial, and perivascular) and abdominal adipose tissue (intraperitoneal, retroperitoneal) |
Thoracic adipose tissue (ThAT): the visceral adipose tissue of the chest comprising of pericardial and non-pericardial thoracic adipose tissue depots, epicardial adipose, and peri-vascular adipose tissue (around any vessel like the coronaries, the aorta, the internal mammary arteries, etc.) |
Epicardial adipose tissue (EAT): the adipose tissue between the visceral pericardium and the myocardium. That includes the peri-vascular adipose tissue around the coronary arteries |
Pericardial adipose tissue: the adipose tissue of the thorax, surrounding the heart located outside the pericardial sac (does not include peri-aortic adipose tissue) |
Paracardial adipose tissue: a term that has been used to refer to adipose tissue close to the heart. Should be abandoned since it has a less clear meaning |
Perivascular adipose tissue (PVAT): the adipose tissue that lies around a vessel in a radial distance from the vascular wall equal to one luminal diameter of the adjacent vessel. For arteries with diameter >2 cm, peri-vascular adipose tissue is defined by convention as the adipose tissue within a distance of 2 cm from the outer vessel wall |
Subcutaneous adipose tissue (SAT): in the hypodermis (i.e. just below the skin); may include the subcutaneous thoracic, abdominal, gluteal, or femoral adipose tissue |
Visceral adipose tissue (VAT): the adipose tissue located inside the abdominal or thoracic cavity, between the viscera; sub-classified into thoracic adipose tissue (pericardial, non-pericardial, epicardial, and perivascular) and abdominal adipose tissue (intraperitoneal, retroperitoneal) |
Thoracic adipose tissue (ThAT): the visceral adipose tissue of the chest comprising of pericardial and non-pericardial thoracic adipose tissue depots, epicardial adipose, and peri-vascular adipose tissue (around any vessel like the coronaries, the aorta, the internal mammary arteries, etc.) |
Epicardial adipose tissue (EAT): the adipose tissue between the visceral pericardium and the myocardium. That includes the peri-vascular adipose tissue around the coronary arteries |
Pericardial adipose tissue: the adipose tissue of the thorax, surrounding the heart located outside the pericardial sac (does not include peri-aortic adipose tissue) |
Paracardial adipose tissue: a term that has been used to refer to adipose tissue close to the heart. Should be abandoned since it has a less clear meaning |
Perivascular adipose tissue (PVAT): the adipose tissue that lies around a vessel in a radial distance from the vascular wall equal to one luminal diameter of the adjacent vessel. For arteries with diameter >2 cm, peri-vascular adipose tissue is defined by convention as the adipose tissue within a distance of 2 cm from the outer vessel wall |
Subcutaneous adipose tissue (SAT): in the hypodermis (i.e. just below the skin); may include the subcutaneous thoracic, abdominal, gluteal, or femoral adipose tissue |
Visceral adipose tissue (VAT): the adipose tissue located inside the abdominal or thoracic cavity, between the viscera; sub-classified into thoracic adipose tissue (pericardial, non-pericardial, epicardial, and perivascular) and abdominal adipose tissue (intraperitoneal, retroperitoneal) |
Thoracic adipose tissue (ThAT): the visceral adipose tissue of the chest comprising of pericardial and non-pericardial thoracic adipose tissue depots, epicardial adipose, and peri-vascular adipose tissue (around any vessel like the coronaries, the aorta, the internal mammary arteries, etc.) |
Epicardial adipose tissue (EAT): the adipose tissue between the visceral pericardium and the myocardium. That includes the peri-vascular adipose tissue around the coronary arteries |
Pericardial adipose tissue: the adipose tissue of the thorax, surrounding the heart located outside the pericardial sac (does not include peri-aortic adipose tissue) |
Paracardial adipose tissue: a term that has been used to refer to adipose tissue close to the heart. Should be abandoned since it has a less clear meaning |
Perivascular adipose tissue (PVAT): the adipose tissue that lies around a vessel in a radial distance from the vascular wall equal to one luminal diameter of the adjacent vessel. For arteries with diameter >2 cm, peri-vascular adipose tissue is defined by convention as the adipose tissue within a distance of 2 cm from the outer vessel wall |
Subcutaneous adipose tissue (SAT): in the hypodermis (i.e. just below the skin); may include the subcutaneous thoracic, abdominal, gluteal, or femoral adipose tissue |
Visceral adipose tissue (VAT): the adipose tissue located inside the abdominal or thoracic cavity, between the viscera; sub-classified into thoracic adipose tissue (pericardial, non-pericardial, epicardial, and perivascular) and abdominal adipose tissue (intraperitoneal, retroperitoneal) |
Thoracic adipose tissue (ThAT): the visceral adipose tissue of the chest comprising of pericardial and non-pericardial thoracic adipose tissue depots, epicardial adipose, and peri-vascular adipose tissue (around any vessel like the coronaries, the aorta, the internal mammary arteries, etc.) |
Epicardial adipose tissue (EAT): the adipose tissue between the visceral pericardium and the myocardium. That includes the peri-vascular adipose tissue around the coronary arteries |
Pericardial adipose tissue: the adipose tissue of the thorax, surrounding the heart located outside the pericardial sac (does not include peri-aortic adipose tissue) |
Paracardial adipose tissue: a term that has been used to refer to adipose tissue close to the heart. Should be abandoned since it has a less clear meaning |
Perivascular adipose tissue (PVAT): the adipose tissue that lies around a vessel in a radial distance from the vascular wall equal to one luminal diameter of the adjacent vessel. For arteries with diameter >2 cm, peri-vascular adipose tissue is defined by convention as the adipose tissue within a distance of 2 cm from the outer vessel wall |
Morphological and functional differences between adipose tissue depots in the chest
. | ![]() | |||
---|---|---|---|---|
. | Subcutaneous adipose tissue (SAT) . | Pericardial adipose tissue (PAT) . | Epicardial adipose tissue (EAT) . | Perivascular adipose tissue (PVAT) . |
Relation with sex | More in females | Abdominal obesity in males | No difference | No difference |
Relation with age | Less with age | Increasing with age | Increasing with age | Unclear |
Adipocyte size | Large | Small | Comparable to PAT | Gradient in adipocyte size (smaller closer to the vascular wall) |
Adipocyte differentiation status | Well differentiated | Poorly differentiated | Comparable to PAT | Dependent on vascular biology |
Vascular-stromal cell content | Less rich vs. VAT | Rich vasculature, high immune-cell content | Comparable to PAT | Dependent on vascular biology |
Biological profile | Less metabolically active, thermogenic potential (beiging) | High metabolic activity, inflammatory profile, lipolytic potential | High metabolic activity, inflammatory profile, lipolytic potential | High secretory profile with non-adipocytes (pre-adipocytes and inflammatory cells) driving its secretome |
Association with metabolic status | Neutral/protective | Strong, negatively associated with (IR)—biology driven by systemic stimuli | Strong (less than PAT)—biology largely driven by myocardial signals | Less than EAT—biology largely driven by vascular signals |
Association with cardiovascular risk | Neutral | Strong | Strong | Strong |
. | ![]() | |||
---|---|---|---|---|
. | Subcutaneous adipose tissue (SAT) . | Pericardial adipose tissue (PAT) . | Epicardial adipose tissue (EAT) . | Perivascular adipose tissue (PVAT) . |
Relation with sex | More in females | Abdominal obesity in males | No difference | No difference |
Relation with age | Less with age | Increasing with age | Increasing with age | Unclear |
Adipocyte size | Large | Small | Comparable to PAT | Gradient in adipocyte size (smaller closer to the vascular wall) |
Adipocyte differentiation status | Well differentiated | Poorly differentiated | Comparable to PAT | Dependent on vascular biology |
Vascular-stromal cell content | Less rich vs. VAT | Rich vasculature, high immune-cell content | Comparable to PAT | Dependent on vascular biology |
Biological profile | Less metabolically active, thermogenic potential (beiging) | High metabolic activity, inflammatory profile, lipolytic potential | High metabolic activity, inflammatory profile, lipolytic potential | High secretory profile with non-adipocytes (pre-adipocytes and inflammatory cells) driving its secretome |
Association with metabolic status | Neutral/protective | Strong, negatively associated with (IR)—biology driven by systemic stimuli | Strong (less than PAT)—biology largely driven by myocardial signals | Less than EAT—biology largely driven by vascular signals |
Association with cardiovascular risk | Neutral | Strong | Strong | Strong |
Morphological and functional differences between adipose tissue depots in the chest
. | ![]() | |||
---|---|---|---|---|
. | Subcutaneous adipose tissue (SAT) . | Pericardial adipose tissue (PAT) . | Epicardial adipose tissue (EAT) . | Perivascular adipose tissue (PVAT) . |
Relation with sex | More in females | Abdominal obesity in males | No difference | No difference |
Relation with age | Less with age | Increasing with age | Increasing with age | Unclear |
Adipocyte size | Large | Small | Comparable to PAT | Gradient in adipocyte size (smaller closer to the vascular wall) |
Adipocyte differentiation status | Well differentiated | Poorly differentiated | Comparable to PAT | Dependent on vascular biology |
Vascular-stromal cell content | Less rich vs. VAT | Rich vasculature, high immune-cell content | Comparable to PAT | Dependent on vascular biology |
Biological profile | Less metabolically active, thermogenic potential (beiging) | High metabolic activity, inflammatory profile, lipolytic potential | High metabolic activity, inflammatory profile, lipolytic potential | High secretory profile with non-adipocytes (pre-adipocytes and inflammatory cells) driving its secretome |
Association with metabolic status | Neutral/protective | Strong, negatively associated with (IR)—biology driven by systemic stimuli | Strong (less than PAT)—biology largely driven by myocardial signals | Less than EAT—biology largely driven by vascular signals |
Association with cardiovascular risk | Neutral | Strong | Strong | Strong |
. | ![]() | |||
---|---|---|---|---|
. | Subcutaneous adipose tissue (SAT) . | Pericardial adipose tissue (PAT) . | Epicardial adipose tissue (EAT) . | Perivascular adipose tissue (PVAT) . |
Relation with sex | More in females | Abdominal obesity in males | No difference | No difference |
Relation with age | Less with age | Increasing with age | Increasing with age | Unclear |
Adipocyte size | Large | Small | Comparable to PAT | Gradient in adipocyte size (smaller closer to the vascular wall) |
Adipocyte differentiation status | Well differentiated | Poorly differentiated | Comparable to PAT | Dependent on vascular biology |
Vascular-stromal cell content | Less rich vs. VAT | Rich vasculature, high immune-cell content | Comparable to PAT | Dependent on vascular biology |
Biological profile | Less metabolically active, thermogenic potential (beiging) | High metabolic activity, inflammatory profile, lipolytic potential | High metabolic activity, inflammatory profile, lipolytic potential | High secretory profile with non-adipocytes (pre-adipocytes and inflammatory cells) driving its secretome |
Association with metabolic status | Neutral/protective | Strong, negatively associated with (IR)—biology driven by systemic stimuli | Strong (less than PAT)—biology largely driven by myocardial signals | Less than EAT—biology largely driven by vascular signals |
Association with cardiovascular risk | Neutral | Strong | Strong | Strong |
Understanding the cross-talk between perivascular adipose tissue and the vascular wall
Adipose tissue secretes bioactive molecules, collectively called adipokines that can affect cardiovascular physiology in an endocrine manner (via their release in systemic circulation). Not all depots affect the cardiovascular system in a similar way. The secretome of the highly vascular VAT is released into the bloodstream, exerting primarily detrimental effects on the cardiovascular system, in an endocrine manner. Conversely, the less active SAT has a neutral relationship with cardiovascular risk, while the accumulation of gluteal AT may even translate into a lower cardiovascular risk, as it secretes largely anti-atherogenic adipokines like adiponectin. Beyond its endocrine effects on the cardiovascular system, AT can also affect the heart and the vascular wall directly, in a paracrine (i.e. by diffusion) or vasocrine (i.e. mediated by the local micro-circulation) way, as shown in Figure 2A.12,22

(A) Routes via which remote and local adipose tissue depots affect the cardiovascular system. (B) Illustration of the major components of the bi-directional interplay between the vascular wall and fat in the peri-vascular space. EC, endothelial cell; eNOS, endothelial nitric oxide synthase; PVAT, perivascular adipose tissue; VSMC, vascular smooth muscle cell. (Reused with permission by Kotanidis and Antoniades.23) An animated video web link23 presents how the cross-talk between PVAT and the vascular wall leads to changes in PVAT texture and composition, visible with computed tomography.
PVAT was originally thought to serve only as a structural support tissue providing physical protection to the blood vessels. However, in the past 20 years its role in regulating vessel function has become increasingly recognized, as it releases numerous vasoactive factors (adipokines) with paracrine (outside-in) effects on the vascular wall, in addition to its ability to also sense signals released from the vessel wall (inside-out) that determine its biological behaviour, as part of a cross-talk between the two.24,25
PVAT actually comprises a diverse collection of cell types including adipocytes, neuronal cells (autonomic nerves), immune cells, and the stromal vascular fraction that consists of pre-adipocytes, fibroblasts, endothelial cells, and mesenchymal stem cells derived from the local micro-circulation.26 In clinical studies, PVAT is generally considered to be a sub-type of WAT, but PVAT’s adipocytes are generally smaller in size, with less lipid droplet accumulation and less differentiated adipocytes, with gene expression patterns often similar to those of adipocytes in BAT, particularly in animal models.27,28 PVAT also contains a community of innate and adaptive immune cells, consisting of lymphocyte (T and B cells), macrophages, eosinophils, NK-cells, and mast cells. In the healthy state, macrophages and T-cells form the dominant immune-cell types in PVAT that together with the eosinophils contribute to the anti-inflammatory effects and anti-constrictive effects of PVAT on the vessel wall.27,29
PVAT releases bioactive molecules such as adipokines, anti-inflammatory and pro-inflammatory factors, miRNAs, and others (e.g. hydrogen sulphide, reactive oxygen species, fatty acid metabolites, etc.) and contributes to the regulation of vascular homeostasis.30 Under physiological conditions, PVAT exerts a net vasodilatory, anti-oxidant, and anti-inflammatory effect on the vasculature.24 The vasodilator effects are due to the secretion of adipocyte-derived relaxing factor, adiponectin, apelin, leptin, and omentin, which induce vasodilatation either by acting directly on vascular smooth muscle cells (e.g. adipocyte-derived relaxing factor, leptin, and adiponectin) and/or indirectly by increasing the generation of endothelium-derived vasodilators such as nitric oxide (NO) and endothelium-derived hyperpolarizing factor (e.g. apelin, omentin, leptin, and adiponectin).1 The paracrine anti-oxidant and anti-inflammatory actions of adipokines released from PVAT under physiological conditions likely involve 5′ adenosine monophosphate-activated protein kinase (AMPK) and NO signalling within the adjacent vessel.23,31,32 Table 3 summarizes the cellular sources and the vascular effects of key PVAT-derived adipokines.
Main bioactive components in PVAT: cell origin and biological functions in the vascular bed
Bioactive component . | Main cell origin . | Vasomotor effects . | Inflammation . | Other biological effects . |
---|---|---|---|---|
Adipokine | ||||
Adiponectin | Adipocyte | eNOS-dependent vasodilatation | Anti-inflammatory | Adenosine 5′-monophosphate-activated protein kinase (AMPK) activation |
Leptin | Adipocyte | eNOS-dependent vasodilatation ET-1-mediated vasoconstriction | Pro-inflammatory | Induce oxidative stress |
Apelin | Adipocyte | Unclear: possibly vasodilator in small arteries and vasoconstrictor in veins9,10 | Anti-inflammatory | Increase glucose utilization (insulin-independent effect)12,13 |
Omentin | Adipocyte, stromal cells | Anti-contractile effects | Anti-inflammatory | Increases NO bioavailability Anti-oxidant effects |
Chemerin | Adipocyte | Vasoconstriction | Pro-inflammatory | Induce adhesion molecules in EC—Induces VSMC proliferation |
Visfatin | Stromal cells, Inflammatory cells (macrophage), adipocyte | Vasoconstriction | Pro-inflammatory | Proliferative response in VSMC |
Resistin | Inflammatory cells (macrophage), adipocyte | Vasoconstriction | Pro-inflammatory | Impairs insulin-stimulated glucose uptake |
SFRP5 | Adipocytes | Vasodilatation | Reduces oxidative stress | |
Wnt5a | Adipocytes | Vasoconstriction | Mediates oxidative stress (NADPH oxidase activity) | |
Cytokines & growth factors | ||||
Interleukin-6 | Inflammatory cells (T-cells, macrophage); adipocyte | Vasoconstriction | Pro-inflammatory | Induce oxidative stress in EC and VSMC |
Interleukin-10 | Inflammatory cells (T-cells, macrophage) | Unclear | Anti-inflammatory | Reduce oxidative stress |
IFN-γ | Inflammatory cells (T-cells, NK-cells), stromal cells | Vasoconstriction | Pro-inflammatory | Impairs endothelium-dependent relaxation oxidative stress |
TNF-α | Inflammatory cells; stromal cells; adipocytes | - | Pro-inflammatory | ROS production |
MCP-1 | Inflammatory cells, adipocytes | - | Pro-inflammatory | - |
VEGF | Adipocytes | - | - | VSMC proliferation |
Other bioactive components | ||||
Hydrogen sulphide | Adipocytes, stroma cells (EC) | Vasodilatation | Anti-oxidant effects at physiological levels. At high levels lead to generation of free radicals | |
Nitric oxide | Endothelial cells (via eNOS) and sympathetic nerves (via nNOS) | Vasorelaxation | Anti-inflammatory | - |
Palmitic acid methyl ester | Adipocytes | Vasodilatation | Pro-inflammatory | Induces ICAM-1 expression |
Angiotensin1–7 | Adipocytes | Vasodilatation | Reduces oxidative stress | |
Ceramides (C16/C24) | Adipocytes | Vasoconstriction | Oxidative stress |
Bioactive component . | Main cell origin . | Vasomotor effects . | Inflammation . | Other biological effects . |
---|---|---|---|---|
Adipokine | ||||
Adiponectin | Adipocyte | eNOS-dependent vasodilatation | Anti-inflammatory | Adenosine 5′-monophosphate-activated protein kinase (AMPK) activation |
Leptin | Adipocyte | eNOS-dependent vasodilatation ET-1-mediated vasoconstriction | Pro-inflammatory | Induce oxidative stress |
Apelin | Adipocyte | Unclear: possibly vasodilator in small arteries and vasoconstrictor in veins9,10 | Anti-inflammatory | Increase glucose utilization (insulin-independent effect)12,13 |
Omentin | Adipocyte, stromal cells | Anti-contractile effects | Anti-inflammatory | Increases NO bioavailability Anti-oxidant effects |
Chemerin | Adipocyte | Vasoconstriction | Pro-inflammatory | Induce adhesion molecules in EC—Induces VSMC proliferation |
Visfatin | Stromal cells, Inflammatory cells (macrophage), adipocyte | Vasoconstriction | Pro-inflammatory | Proliferative response in VSMC |
Resistin | Inflammatory cells (macrophage), adipocyte | Vasoconstriction | Pro-inflammatory | Impairs insulin-stimulated glucose uptake |
SFRP5 | Adipocytes | Vasodilatation | Reduces oxidative stress | |
Wnt5a | Adipocytes | Vasoconstriction | Mediates oxidative stress (NADPH oxidase activity) | |
Cytokines & growth factors | ||||
Interleukin-6 | Inflammatory cells (T-cells, macrophage); adipocyte | Vasoconstriction | Pro-inflammatory | Induce oxidative stress in EC and VSMC |
Interleukin-10 | Inflammatory cells (T-cells, macrophage) | Unclear | Anti-inflammatory | Reduce oxidative stress |
IFN-γ | Inflammatory cells (T-cells, NK-cells), stromal cells | Vasoconstriction | Pro-inflammatory | Impairs endothelium-dependent relaxation oxidative stress |
TNF-α | Inflammatory cells; stromal cells; adipocytes | - | Pro-inflammatory | ROS production |
MCP-1 | Inflammatory cells, adipocytes | - | Pro-inflammatory | - |
VEGF | Adipocytes | - | - | VSMC proliferation |
Other bioactive components | ||||
Hydrogen sulphide | Adipocytes, stroma cells (EC) | Vasodilatation | Anti-oxidant effects at physiological levels. At high levels lead to generation of free radicals | |
Nitric oxide | Endothelial cells (via eNOS) and sympathetic nerves (via nNOS) | Vasorelaxation | Anti-inflammatory | - |
Palmitic acid methyl ester | Adipocytes | Vasodilatation | Pro-inflammatory | Induces ICAM-1 expression |
Angiotensin1–7 | Adipocytes | Vasodilatation | Reduces oxidative stress | |
Ceramides (C16/C24) | Adipocytes | Vasoconstriction | Oxidative stress |
eNOS, endothelial nitric oxide synthase; ET-1, endothelin-1; ICAM-1, intercellular adhesion molecule-1; IFN, interferon; MCP, monocyte chemoattractant protein-1; NO, nitric oxide; nNOS, neuronal nitric oxide synthase; ROS, reactive oxygen species; SFRP5, secreted frizzled-related protein 5; TNF, tumour necrosis factor; VEGF, vascular endothelial growth factor; VSMC, vascular smooth muscle cell; Wnt5a, wingless-type MMTV integration site family, member 5A.
Main bioactive components in PVAT: cell origin and biological functions in the vascular bed
Bioactive component . | Main cell origin . | Vasomotor effects . | Inflammation . | Other biological effects . |
---|---|---|---|---|
Adipokine | ||||
Adiponectin | Adipocyte | eNOS-dependent vasodilatation | Anti-inflammatory | Adenosine 5′-monophosphate-activated protein kinase (AMPK) activation |
Leptin | Adipocyte | eNOS-dependent vasodilatation ET-1-mediated vasoconstriction | Pro-inflammatory | Induce oxidative stress |
Apelin | Adipocyte | Unclear: possibly vasodilator in small arteries and vasoconstrictor in veins9,10 | Anti-inflammatory | Increase glucose utilization (insulin-independent effect)12,13 |
Omentin | Adipocyte, stromal cells | Anti-contractile effects | Anti-inflammatory | Increases NO bioavailability Anti-oxidant effects |
Chemerin | Adipocyte | Vasoconstriction | Pro-inflammatory | Induce adhesion molecules in EC—Induces VSMC proliferation |
Visfatin | Stromal cells, Inflammatory cells (macrophage), adipocyte | Vasoconstriction | Pro-inflammatory | Proliferative response in VSMC |
Resistin | Inflammatory cells (macrophage), adipocyte | Vasoconstriction | Pro-inflammatory | Impairs insulin-stimulated glucose uptake |
SFRP5 | Adipocytes | Vasodilatation | Reduces oxidative stress | |
Wnt5a | Adipocytes | Vasoconstriction | Mediates oxidative stress (NADPH oxidase activity) | |
Cytokines & growth factors | ||||
Interleukin-6 | Inflammatory cells (T-cells, macrophage); adipocyte | Vasoconstriction | Pro-inflammatory | Induce oxidative stress in EC and VSMC |
Interleukin-10 | Inflammatory cells (T-cells, macrophage) | Unclear | Anti-inflammatory | Reduce oxidative stress |
IFN-γ | Inflammatory cells (T-cells, NK-cells), stromal cells | Vasoconstriction | Pro-inflammatory | Impairs endothelium-dependent relaxation oxidative stress |
TNF-α | Inflammatory cells; stromal cells; adipocytes | - | Pro-inflammatory | ROS production |
MCP-1 | Inflammatory cells, adipocytes | - | Pro-inflammatory | - |
VEGF | Adipocytes | - | - | VSMC proliferation |
Other bioactive components | ||||
Hydrogen sulphide | Adipocytes, stroma cells (EC) | Vasodilatation | Anti-oxidant effects at physiological levels. At high levels lead to generation of free radicals | |
Nitric oxide | Endothelial cells (via eNOS) and sympathetic nerves (via nNOS) | Vasorelaxation | Anti-inflammatory | - |
Palmitic acid methyl ester | Adipocytes | Vasodilatation | Pro-inflammatory | Induces ICAM-1 expression |
Angiotensin1–7 | Adipocytes | Vasodilatation | Reduces oxidative stress | |
Ceramides (C16/C24) | Adipocytes | Vasoconstriction | Oxidative stress |
Bioactive component . | Main cell origin . | Vasomotor effects . | Inflammation . | Other biological effects . |
---|---|---|---|---|
Adipokine | ||||
Adiponectin | Adipocyte | eNOS-dependent vasodilatation | Anti-inflammatory | Adenosine 5′-monophosphate-activated protein kinase (AMPK) activation |
Leptin | Adipocyte | eNOS-dependent vasodilatation ET-1-mediated vasoconstriction | Pro-inflammatory | Induce oxidative stress |
Apelin | Adipocyte | Unclear: possibly vasodilator in small arteries and vasoconstrictor in veins9,10 | Anti-inflammatory | Increase glucose utilization (insulin-independent effect)12,13 |
Omentin | Adipocyte, stromal cells | Anti-contractile effects | Anti-inflammatory | Increases NO bioavailability Anti-oxidant effects |
Chemerin | Adipocyte | Vasoconstriction | Pro-inflammatory | Induce adhesion molecules in EC—Induces VSMC proliferation |
Visfatin | Stromal cells, Inflammatory cells (macrophage), adipocyte | Vasoconstriction | Pro-inflammatory | Proliferative response in VSMC |
Resistin | Inflammatory cells (macrophage), adipocyte | Vasoconstriction | Pro-inflammatory | Impairs insulin-stimulated glucose uptake |
SFRP5 | Adipocytes | Vasodilatation | Reduces oxidative stress | |
Wnt5a | Adipocytes | Vasoconstriction | Mediates oxidative stress (NADPH oxidase activity) | |
Cytokines & growth factors | ||||
Interleukin-6 | Inflammatory cells (T-cells, macrophage); adipocyte | Vasoconstriction | Pro-inflammatory | Induce oxidative stress in EC and VSMC |
Interleukin-10 | Inflammatory cells (T-cells, macrophage) | Unclear | Anti-inflammatory | Reduce oxidative stress |
IFN-γ | Inflammatory cells (T-cells, NK-cells), stromal cells | Vasoconstriction | Pro-inflammatory | Impairs endothelium-dependent relaxation oxidative stress |
TNF-α | Inflammatory cells; stromal cells; adipocytes | - | Pro-inflammatory | ROS production |
MCP-1 | Inflammatory cells, adipocytes | - | Pro-inflammatory | - |
VEGF | Adipocytes | - | - | VSMC proliferation |
Other bioactive components | ||||
Hydrogen sulphide | Adipocytes, stroma cells (EC) | Vasodilatation | Anti-oxidant effects at physiological levels. At high levels lead to generation of free radicals | |
Nitric oxide | Endothelial cells (via eNOS) and sympathetic nerves (via nNOS) | Vasorelaxation | Anti-inflammatory | - |
Palmitic acid methyl ester | Adipocytes | Vasodilatation | Pro-inflammatory | Induces ICAM-1 expression |
Angiotensin1–7 | Adipocytes | Vasodilatation | Reduces oxidative stress | |
Ceramides (C16/C24) | Adipocytes | Vasoconstriction | Oxidative stress |
eNOS, endothelial nitric oxide synthase; ET-1, endothelin-1; ICAM-1, intercellular adhesion molecule-1; IFN, interferon; MCP, monocyte chemoattractant protein-1; NO, nitric oxide; nNOS, neuronal nitric oxide synthase; ROS, reactive oxygen species; SFRP5, secreted frizzled-related protein 5; TNF, tumour necrosis factor; VEGF, vascular endothelial growth factor; VSMC, vascular smooth muscle cell; Wnt5a, wingless-type MMTV integration site family, member 5A.
Bi-directional cross-talk between peri-vascular adipose tissue and the vascular wall
PVAT is involved in bi-directional interactions with the vascular wall. PVAT responds to paracrine signals from vascular cells, facilitating phenotypic changes of PVAT adipocytes and modifying the composition of their secretome, which exerts paracrine effects back on to the vascular wall. This cross-talk is different in health and disease. In the presence of high vascular oxidative stress, the vascular wall secretes lipid peroxidation products such as 4-hydroxynonenal (4-HNE), which diffuse to the surrounding PVAT, to activate peroxisome proliferator-activated receptor (PPAR)-γ signalling in perivascular adipocytes which in turn leads to up-regulation of adiponectin expression and secretion from PVAT. The latter then suppress vascular oxidative stress as a local defense mechanism against vascular oxidative damage.33–35 In a similar way, PVAT adipocytes sense inflammatory molecules secreted from the vascular wall [e.g. interleukin (IL)-6, tumour necrosis factor (TNF)-α, interferon (IFN)-γ, etc.], and change from storage cells to secretory cells, activating lipolysis and inhibiting adipogenesis.12 In summary, the bi-directional communication (Figure 2B) between vascular cells and PVAT plays a role in regulating vascular function. A better understanding of PVAT-specific secretome changes in response to vascular signals, including adipokines, cytokines, and lipid peroxidation products, is essential to identify new diagnostic biomarkers36 and therapeutic targets in cardiovascular medicine.37
Perivascular adipose tissue as a source of therapeutic targets in cardiovascular disease
The paracrine effects of PVAT, particularly on vascular tone and vascular inflammation, are significantly altered in diseases such as (pre)diabetes, hypertension, and cardiac diseases. Indeed, in pathological conditions, cytokines and classical adipokines dysregulate the expression of critical genes involved in vascular redox balance, such as NADPH oxidases (Nox) or endothelial NO synthase, leading to endothelial dysfunction. For example, while leptin directly induces vasorelaxation, prolonged exposure of endothelial cells to leptin leads to increased oxidative stress, activation of NADPH oxidases, and endothelial dysfunction.38 Resistin and visfatin have also been shown to induce pro-oxidant and pro-inflammatory phenotypes in the vasculature.39
It has been shown that PVAT also secretes recently identified adipokines such as secreted frizzled-related protein 5 (SFRP5) and ligands regulating non-canonical Wnt-signalling (e.g. wnt5a), which play a major role in controlling vascular redox state.40 Also, the metabolic and secretory function of PVAT is regulated by signals originating from vascular cells. For example, it has been shown that cytokines released either from atherosclerotic plaques or by the inflamed vascular wall in the absence of atherosclerotic plaques (such as IL-6 or TNF-α) leads to adipocyte dedifferentiation and decreased lipid storage; these changes go hand in hand with an increased ability to further promote perivascular inflammation by inducing the expression of chemokines such as RANTES, that further drive the development of perivascular inflammation.41 Similarly, in hypertensive mice, eosinophilic granulocytes within PVAT affect vascular resistance and blood pressure.29
Evaluating peri-vascular adipose tissue biology in metabolically healthy vs. unhealthy obesity
Obesity does not always result in metabolic dysregulation, and there are metabolically ‘healthy’ individuals with obesity.42 In general, the metabolically ‘healthy’ individuals with obesity carry an expanded total AT mass in the absence of metabolic diseases such as type 2 diabetes mellitus, dyslipidaemia, or hypertension (such as sumo wrestlers). While the local accumulation of PVAT has been consistently associated with the development of cardiometabolic complications in obesity,43,44 there is evidence that metabolically healthy obesity is accompanied by a more favourable PVAT inflammatory status than metabolically unhealthy obesity.11 The ‘metabolically healthy’ obesity state is more often observed in young, physically active individuals with a good nutritional status and low storage of ectopic and visceral fat.45
Perivascular adipose tissue in diabetes and insulin resistance
PVAT in skeletal muscles influences insulin-stimulated perfusion (via insulin-mediated micro-vascular recruitment), mitochondrial protein expression, and glucose uptake.9 Insulin-mediated micro-vascular recruitment and related vasodilatation expands the endothelial surface area in direct contact with blood, facilitating extraction of glucose and insulin into the muscle interstitium. This vasodilatory effect of insulin strongly depends on locally (in PVAT) produced adiponectin which penetrates the vascular wall either by diffusion (paracrine effect) or possibly through the adipomuscular arterioles (vasocrine effect),9 although the latter remains largely speculative. PVAT is also important for insulin regulation of muscle glucose metabolism, controlling heat shock protein (HSP) expression. HSP 90AB1 is involved in the regulation of glucose and fatty acid metabolism and the expression of the mitochondrial protein complexes engaged in the respiratory chain.46
PVAT is dysfunctional in individuals with insulin resistance and type 2 diabetes mellitus,47 as it loses its ability to affect insulin-induced vasodilatation and to antagonize sympathetic tone, predominately due to impaired adiponectin secretion.47 In mice, inflammation of PVAT impairs insulin-induced vasodilatation during weight gain, contributing to impaired muscle blood flow and insulin resistance.48 Indeed, the removal of PVAT (in vivo animal experiments) causes decreased expression of mitochondrial electron transport chain components, a characteristic of muscle insulin resistance in diabetes.9 Impaired cross-talk between PVAT and micro-vascular endothelium also predisposes to insulin resistance and type 2 diabetes.32 However, the mechanisms by which PVAT biology shifts towards its pro-insulin resistance phenotype are not fully understood. Under diabetic conditions, the biosynthetic activity of para-aortic PVAT shifts towards a pro-inflammatory [increased C-reactive protein (CRP), chemokine (C–C motif) ligand 2, CD36], pro-oxidant (increased aldose reductase and reduced anti-oxidant deference enzymes), and vasoconstrictive state.49 Pro-inflammatory phenotype of PVAT can be induced by high-fat feeding.50 Since the skeletal muscles account for the greatest proportion of insulin resistance in diabetes, it might be speculated that PVAT dysfunction is one of the key steps for the development of insulin resistance and type 2 diabetes, although further data are needed to document this role.
Perivascular adipose tissue in arterial hypertension
Inflammation plays a pivotal role in the pathogenesis of hypertension,51,52 immune-cell activation and infiltration into the vasculature, increases blood pressure,53 and causes target organ damage.54,55 In the vasculature, the PVAT is one of the main sites of immune-cell infiltration in hypertension. Angiotensin II, salt, as well as initial pre-hypertensive increases of blood pressure induce chemokine release from the PVAT which mediates perivascular inflammation. This includes chemokines such as RANTES, IP-10, MCP-1 attracting T-cells as well as macrophages into PVAT.56,57 These cells release effector cytokines implicated in hypertension, such as IFN-γ, IL-17, TNF-α, IL-6, that cause vascular dysfunction, oxidative stress, and vascular stiffness, all of which are critical processes in hypertension.51,57 The role of PVAT inflammation in the regulation of blood pressure provides a further mechanistic link between obesity, atherosclerosis, and hypertension.50,58
Perivascular adipose tissue and sex differences
Women generally exhibit significantly higher pericardial and epicardial fat volumes with declining oestrogen levels following menopause.1 This observation highlights a potential role for ectopic fat depots in sex differences associated with cardiovascular risk.1,8 Although there is a paucity of human data, healthy female pigs show greater PVAT-derived relaxing factors such as adiponectin and lower levels of adipose-derived contracting factor such as thromboxane A2, compared to healthy males.54,55 Additionally, PVAT releases NO and cyclooxygenase metabolites to induce coronary vasodilatation in young female but not male pigs, suggesting a sex-specific effect of PVAT on vascular tone.54 Nevertheless, reduction of oestrogen levels following menopause is associated with a change from prevalent release of vasorelaxing factors towards vasoconstrictive as well as pro-inflammatory adipokines.59 Ovariectomy increased the vasoconstrictor endothelial-dependent responses by reducing endothelial NO and increasing thromboxane A2/cyclooxygenase signalling and attenuated the vasodilatory effects of PVAT, in female rats as compared with sham rats suggesting that oestrogen regulates PVAT-mediated micro-vascular tone.58 Oestrogen receptors are expressed in AT, but their potential role in PVAT remains unknown, while the role of oestrogen-receptor signalling as a therapeutic target within the human PVAT is unclear.
Pharmacological targeting of perivascular adipose tissue
Given its well-established role in the pathophysiology of vascular disease, the PVAT is a rational therapeutic target for new approaches in cardiovascular medicine. Current anti-diabetic treatments partly exert their beneficial cardiovascular effects by acting on AT. There is evidence that PVAT phenotype of animals and humans changes quickly in response to changes in diet50,60 or physical activity,61 while pharmacological anti-diabetic treatments may also affect PVAT’s function. Glucagon-like peptide-1 (GLP-1) receptor expression levels in adipocytes are associated with insulin resistance, and GLP-1 receptor agonists promote the differentiation of adipocytes and restore adipocyte health in cell culture models.62 In humans, the treatment of patients with obesity with the GLP-1 analogue liraglutide has beneficial effects on plasma lipids;63 while maintaining weight loss, liraglutide also lowers apolipoprotein B plasma levels which may help to reduce cardiovascular disease risk64 For example, fasting GLP-1 levels are associated with decreased carotid intima-media thickness;64 since AT-derived sphingolipids induce vascular dysfunction and liraglutide reduces plasma C16:0-ceramide release levels, the anti-atherogenic effects of GLP-1 analogues may be partly related to their effects on AT ceramides.65,66 Sodium–glucose cotransporter 2 inhibitors (SGLT2-i) also exert beneficial effects on human AT. For example, the SGLT2-i empagliflozin promotes the browning of WAT and activates resident M2 macrophages to attenuate obesity-induced inflammation and insulin resistance in animal models.67 In humans, empagliflozin treatment reduces the volume of EAT, which is closely linked to cardiometabolic risk.68 Whether the beneficial effects of SGLT2-I also include effects on PVAT biology and PVAT-vascular wall interactions remains to be seen.69 Statins lower vascular inflammation and this is reflected in respective changes in PVAT phenotype after an acute coronary syndrome (ACS) and statin-treatment initiation.12 Statins increase H2S bioavailability in PVAT and this may contribute to the anti-contractile vascular effects of atorvastatin.70 Other potential strategies to restore PVAT health, include the PPAR-γ agonist rosiglitazone (albeit with known adverse effects in congestive heart failure) and cannabinoid CB1 receptor agonists to promote H2S release from PVAT, or inhibition of PVAT inflammation with melatonin or cytokine antagonists, or enhancers of adiponectin expression.71 There is an unmet need to test PVAT-specific drug-delivery systems, to assess the effectiveness of PVAT as a direct therapeutic target in cardiovascular disease.
Perivascular adipose tissue as a source of diagnostic and prognostic biomarkers
Imaging perivascular adipose tissue: quantity vs. quality
The development of new imaging modalities and the incorporation of computational systems in the analysis and interpretation of medical images has opened new horizons in measuring the volume of AT (e.g. EAT as a surrogate marker of metabolically unhealthy obesity which predicts non-cardiovascular mortality)72 or even its ‘quality’, by using complex image post-processing. The amount and type of information provided by each imaging modality is different but computed tomography (CT) is considered to be the gold standard in visualizing and characterizing PVAT, due to its very high spatial resolution, and the distinct attenuation signals of AT, falling typically between −30 and −190 Hounsfield units (HU). Other imaging modalities still have major limitations; for example, echocardiography can not be used for tissue characterization or for coronary artery imaging; positron emission tomography (PET) suffers from high noise/signal ratio coming from vessels and/or underlying myocardium; and magnetic resonance imaging (MRI) still lacks the anatomical resolution for precise imaging of coronary arteries. Table 4 summarizes the value of different imaging modalities in assessing PVAT phenotype.
Modality . | Measurements characteristics . | Strengths . | Limitations . |
---|---|---|---|
Echocardiography![]() | EAT thickness |
|
|
Computed tomography![]() | EAT thickness, area, volume, attenuation & quality, i.e. peri-vascular FAI, peri-vascular fat radiomic profile |
|
|
Magnetic resonance imaging![]() | EAT thickness, area, volume proton density fat fraction |
|
|
Positron emission tomography![]() | SUV, TBR |
|
|
Modality . | Measurements characteristics . | Strengths . | Limitations . |
---|---|---|---|
Echocardiography![]() | EAT thickness |
|
|
Computed tomography![]() | EAT thickness, area, volume, attenuation & quality, i.e. peri-vascular FAI, peri-vascular fat radiomic profile |
|
|
Magnetic resonance imaging![]() | EAT thickness, area, volume proton density fat fraction |
|
|
Positron emission tomography![]() | SUV, TBR |
|
|
3D, three-dimensional; AI, artificial intelligence; CT, computed tomography; EAT, epicardial adipose tissue; FAI, fat attenuation index; PVAT, peri-vascular adipose tissue; SUV, standardized uptake values; TBR, target-to-background ratio.
Modality . | Measurements characteristics . | Strengths . | Limitations . |
---|---|---|---|
Echocardiography![]() | EAT thickness |
|
|
Computed tomography![]() | EAT thickness, area, volume, attenuation & quality, i.e. peri-vascular FAI, peri-vascular fat radiomic profile |
|
|
Magnetic resonance imaging![]() | EAT thickness, area, volume proton density fat fraction |
|
|
Positron emission tomography![]() | SUV, TBR |
|
|
Modality . | Measurements characteristics . | Strengths . | Limitations . |
---|---|---|---|
Echocardiography![]() | EAT thickness |
|
|
Computed tomography![]() | EAT thickness, area, volume, attenuation & quality, i.e. peri-vascular FAI, peri-vascular fat radiomic profile |
|
|
Magnetic resonance imaging![]() | EAT thickness, area, volume proton density fat fraction |
|
|
Positron emission tomography![]() | SUV, TBR |
|
|
3D, three-dimensional; AI, artificial intelligence; CT, computed tomography; EAT, epicardial adipose tissue; FAI, fat attenuation index; PVAT, peri-vascular adipose tissue; SUV, standardized uptake values; TBR, target-to-background ratio.
Perivascular adipose tissue as sensor of disease signals from the vascular wall
As discussed earlier, in cardiovascular disease states, the human arterial wall secretes various mediators such as oxidation products (e.g. 4-HNE) which diffuse to PVAT, triggering ‘re-programming’ of adipocytes from quiet lipid-storage cells to active biosynthetic cells secreting more anti-oxidant adipokines such as adiponectin, back to the vascular wall as a ‘defense mechanism’ against vascular oxidative damage.6,33,35 Inflammatory molecules from the vascular wall diffuse into PVAT12 and stall the differentiation of pre-adipocytes into mature adipocytes in PVAT.12 Moreover, vessel-derived inflammatory molecules induce peri-vascular lipolysis and lead to a gradient of adipocyte size around the inflamed artery, with smaller and low fat-containing adipocytes close to the vessel, transiting into larger and fat-filled adipocytes further away from the outer vascular surface.6,12 This gradient in adipocyte size within PVAT surrounding the inflamed artery results into higher lipid/water ratio in PVAT’s layers close to the inflamed vascular wall.10,12 This gradient of PVAT’s structure and composition around inflamed arteries may serve as an internal ‘thermometer’ of vascular inflammation, if it can be visualized and quantified non-invasively. The creation of 3D gradients of adipocyte size around inflamed arteries is demonstrated in Figure 3.

Schematic representation of the biology underlying the detection of coronary inflammation by imaging perivascular adipose tissue (PVAT). Illustration of a healthy artery and surrounding PVAT (bottom) and the resulting PVAT phenotype in states of high vascular wall inflammation (top). Biopsies of PVAT surrounding inflamed vessels demonstrates high macrophage infiltration and contains small adipocytes that when cultured in the presence of inflammatory cytokines, do not store intracellular lipids. Indeed, in the presence of vascular inflammation there is a steep change in adipocyte size and lipid:water content with increasing distance from the vascular wall due to the paracrine effects of vascular inflammation on surrounding peri-vascular adipose tissue (PVAT). These changes in lipid:water balance can be detected by coronary computed tomography angiography (CCTA), visualized by the fat attenuation index (FAI) mapping of PVAT and quantified using FAI-Score. The latter is interpreted clinically by using age and gender nomograms, and when it is used in prognostic models with plaque and clinical risk factors, it provides a powerful was to calculate the patient’s specific risk for cardiovascular events. An example of a patient with high FAI-Score at baseline who reduced vascular inflammation after 1-year treatment with atorvastatin 40 mg once daily. Images obtained with permission from Antonopoulos et al.12 The animated images on the process by which vascular inflammation drives changes to PVAT visible by CCTA have been obtained with permission from this animated video web link.23
Using computed tomography imaging of perivascular adipose tissue to measure vascular inflammation
Computed tomography can be used to extract not only quantitative (volumetric) information about PVAT, but also qualitative information about its structure and composition (including its 3D texture).13 Indeed, the gradient in lipid accumulation and adipocyte size around inflamed coronary arteries can be visualized and quantified from routine coronary CT angiograms (CCTA).12 This continuum of morphological changes in PVAT can be detected as a gradient in the CT signal attenuation in the peri-vascular space (within the window of −30 to −190 HU).12 A metric developed to quantify these 3D attenuation gradients in CCTA imaging of PVAT is the perivascular fat attenuation index (FAI).12 This measurement incorporates corrections for technical scan parameters that affect the attenuation readings around human arteries, in a non-linear way. Perivascular FAI has been biologically and clinically validated and it is now considered as the main imaging biomarker of coronary inflammation extracted from CCTA (Figure 3).73 Perivascular FAI was originally measured around the proximal segment of the right coronary artery (RCA) over a 40 mm segment (10–50 mm from RCA origin) in a radial distance from the arterial wall equal to the diameter of the underlying artery, as it was technically easier to validate the method in that vessel.12 However, further validation studies have led to the development of appropriate algorithms that calculate peri-vascular FAI around the proximal segments of the left circumflex artery (LCx) as well as the left anterior descending artery (LAD).36,74 Analysis of the PVAT around the proximal segments of epicardial coronary arteries can be used as a measure of the overall background inflammatory burden of the respective coronary artery.10 Standardized FAI measurements could take into account any side branches, subtracting the PVAT volume of the side branch from the volume included into the calculation of FAI around the main epicardial artery. This becomes very important when attempting to measure FAI in mid-/distal segments of the coronary arteries.
A per-lesion analysis of PVAT may be also applied around individual coronary plaques to detect inflammatory signals. However, crude peri-coronary attenuation changes along the vessels (an effect driven by the number of branches as well as true biological reasons), and this is taken into account in the algorithm calculating FAI, especially when extending beyond the proximal vessels; this is an approach that is not available in the clinical version of the algorithm, as it is not fully validated yet. This restriction is particularly relevant when a lesion-specific analysis of FAI is performed, to discriminate between stable and unstable lesions; although the lesion-specific FAI analysis is a great experimental tool, it should not be used clinically until fully validated and regulatory cleared. Peri-vascular FAI increases significantly around vulnerable/ruptured plaques in patients with an ACS.10,12 The average peri-coronary AT attenuation is higher around atherosclerotic coronary segments compared to coronary segments without disease,75 predicts non-calcified plaque progression,76 while there are significant differences in average peri-coronary fat attenuation around culprit vs. non-culprit lesions of patients with ACS.19,77,78 The measurement of peri-coronary fat attenuation is also nearly colinear to 18FNaF coronary uptake, measured using PET–CT, which is considered the gold standard to measure micro-calcification in atherosclerotic plaques, an indirect metric of inflammation in vivo (Figure 4A).79 Although higher peri-coronary attenuation has been reported around lesions of intermediate luminal severity with a low fractional flow reserve (FFR),81,82 these findings should be interpreted with caution; FAI is a metric of vascular inflammation and any association with FFR could be explained by unaccounted confounders (e.g. atheroma burden). The two methods provide complementary results, as they capture different biology (local vascular inflammation vs. haemodynamic compromise due to local atheroma, inflamed or not), and associations between them are likely to be indirect.

Pericoronary adipose tissue attenuation for detecting vascular inflammation. (A) Peri-coronary fat attenuation index (FAI) is increased around culprit lesions in acute coronary syndrome patients with evidence of inflammation as assessed by 18F-sodium fluoride (18FNaF) by positron emission tomography/computed tomography (adapted from Kwiecinski et al.79). (B) Changes in FAI of perivascular adipose tissue (PVAT) around ruptured (culprit) atherosclerotic lesions of acute myocardial infarction patients, non-culprit lesions of the same patients, or lesions in stable CAD patients.12 (C) Temporal changes in FAI around a culprit lesion in patients with acute ST elevation myocardial infarction (n = 10). (D) Prognostic value of FAI for cardiac mortality and (E) cardiac mortality or non-fatal myocardial infarction in CRISP-CT study in a cohort of 2040 patients undergoing diagnostic CCTA in Cleveland Clinic, US.74 (F) Prognostic value of unadjusted coronary PVAT attenuation values in SCOT-HEART trial in 1697 evaluable participants.74 (G) The superior performance of fully weighted FAI vs. unadjusted PVAT density; unadjusted PVAT density had borderline predictive value for cardiac mortality, whereas fully weighted FAI led to improved risk prediction by 13.6% in CRISP-CT study.80 (H) Standardization of coronary peri-vascular FAI and nomograms for age (FAI-Score) for left anterior descending artery (LAD) right coronary artery (RCA) and left circumflex coronary artery (LCx). FAI-Score informs on the coronary vessel-specific inflammation burden and the associated relative risk for a fatal cardiac event compared to the age group of reference. For instance, a young individual with no traditional risk factors may be at low absolute risk for a fatal cardiac event; however, a high FAI-Score may indicate increased relative risk for cardiac events in the long-term as a result of sub-clinical vascular inflammation.36 All figure panels were reproduced with permission from the authors/publishers. CAD,coronary artery disease.
Using fat attenuation index measurements in clinical practice: challenges and opportunities
Measurement of peri-coronary FAI is derived from the post-processing of standard CCTA images, and therefore its calculation involves all typical requirements for acquiring a CCTA scan. These include medication (beta-blockers, nitroglycerine), ECG gating, and radiation exposure as per Society of Cardiovascular Computed Tomography guidelines,83 while it suffers from the same technical limitations of CCTA interpretation (motion artefacts, arrhythmias, etc.). Nonetheless in the Cardiovascular RISk Prediction using CT (CRISP-CT)74 study only 5.6% of the scans were not analysable for FAI because of poor scan quality (e.g. motion artefacts) and these were also clinically non-diagnostic. Peri-coronary FAI is a crude method to allow assessment of coronary inflammation, and it performs well as a research tool. However, translating this into a clinically meaningful reading of coronary inflammation that makes sense for individual patient management is challenging. Indeed, the measurement of attenuation on CCTA is influenced by various technical factors (e.g. tube voltage),84 biological factors (e.g. the background adipocyte size is driving the physiological range of attenuation measurements to more negative values, resulting into respective shifts of the expected normal values), and anatomical factors (e.g. the segment of the epicardial coronary tree where the measurements are performed affects the expected attenuation values, etc.).12 A recent study has presented a new algorithm (FAI-Score) which corrects the FAI values for a range of technical, biological, and anatomical factors, and provides a standardized metric of the degree of background coronary inflammation for each of the three epicardial coronary arteries separately. Indeed, the FAI-Score is expressed in arbitrary units, and to be clinically meaningful it needs to be interpreted on age- and sex-specific nomograms (Figure 3).36 This artery-specific measurement is currently the only regulatory cleared metric of coronary inflammation derived from CCTA in Europe, and it is measured separately for each epicardial coronary artery. However, the local measurement of peri-vascular FAI around specific atherosclerotic plaques (plaque-specific inflammatory burden, Figure 4B) remains a promising research tool to detect the inflamed plaque, but it is neither validated clinically nor regulatory cleared, so it cannot be used in clinical practice yet.13
Perivascular fat attenuation index and responsiveness to anti-inflammatory treatments
Evidence suggests that peri-vascular FAI changes significantly in response to anti-inflammatory treatments.15,85 A recent study demonstrated significant reduction in peri-coronary FAI around non-calcified and mixed coronary plaques (but not calcified plaques) at 1 year after the initiation of statin treatment,15 confirming the anti-inflammatory effect of statins. In a prospective clinical study involving patients with psoriasis, a condition which is associated with increased vascular inflammation, biological anti-inflammatory agents (i.e. anti-TNF-α or anti-IL-12/23 and anti-IL-17 therapies) reduced peri-vascular FAI within 1 year of starting treatment.85 In ACS, the culprit lesion inflammatory burden (calculated as the area under the curve of FAI changes across the atherosclerotic plaque, above the baseline value defined by a reference segment proximal to the plaque; Figure 4B and C) is elevated, and it is returning to a steady state within 6 months of the event, in at least 80% of the patients.10,12,13 The remaining ∼20% of the patients whose atherosclerotic plaques do not ‘cool down’ within 6 months, may be more likely to develop recurrent ACS, and could represent a target population for anti-inflammatory agents like colchicine or novel therapeutics targeting coronary inflammation.10 Further randomized clinical trials are needed to quantify the effect size of various anti-inflammatory treatments on coronary inflammation, measured using this method.
Using perivascular adipose tissue imaging for risk prediction: is it prime time for its use in clinical practice?
The CRISP-CT study explored the prognostic value of peri-coronary FAI in two independent cohorts in a total of 3912 patients, followed for up to a decade post-CCTA.74,86 Peri-vascular FAI around the three epicardial coronary arteries was strongly prognostic of both cardiac mortality [hazard ratio (HR) 5.6 and HR 9.0 in the two cohorts, above vs. below a FAI threshold of −70.1 HU, after correction for all risk factors, high-risk plaque (HRP), degree of coronary stenosis, etc.] and non-fatal myocardial infarction (HR 5.0 using the same FAI threshold and following the same corrections mentioned above) (Figure 4D and E).74 In the SCOT-HEART study,87 uncorrected measurement of peri-coronary attenuation above and below the −70 HU cut-off was significantly prognostic for non-fatal cardiac events but with a smaller effect size compared to the weighted FAI used in CRISP-CT (Figure 4F). These findings are consistent with previous observations suggesting a significantly lower prognostic value of uncorrected peri-coronary attenuation vs. the weighted FAI measurement (Figure 4G).88 Moreover, the FAI cut-off used in the CRISP-CT study (−70.1 HU) was derived from Cox-regression models, and it cannot be used as a meaningful cut-off in clinical practice. As mentioned earlier, the absolute FAI values are influenced by various technical, biological, and anatomical factors, and corrected measures like the FAI-Score projected on nomograms are are required for standardized measurements of coronary inflammation.89 Indeed, a FAI-Score above the 50th percentile in the RCA and the LAD is related to a two-fold increase in relative risk for fatal cardiac events, while increases over the 75th and 95th percentiles are related with a 2.4- and 3.2-fold increase of the relative risk, respectively (Figure 4H).89 Interestingly, for the LCx, a FAI-Score above the 95th percentile is needed to achieve ∼2.4 times increase of the relative risk for fatal cardiac events, possibly because the LCx is often a small vessel and accounts for fewer fatal cardiac events compared to the other two main coronary branches.89
Using peri-coronary fat imaging in clinical practice
The information provided by the FAI-Score is complementary to existing risk factors as well as the atherosclerotic plaque volume (calcified or non-calcified plaque burden) and/or the characteristics of vulnerable plaque detected on CCTA (e.g. HRP features like low-attenuation plaque, positive remodelling, spotty calcification, and napkin ring sign).90 A prognostic model that includes the FAI-Score, atherosclerotic plaque burden, as well as clinical risk factors was developed in the US population of the CRISP-CT study and validated in the European population against hard endpoints, reclassifying ∼16% of the patients to a higher and ∼20% to a lower risk category for cardiac mortality.36 Such a model has been proposed for use in conjunction with the European Society of Cardiology (ESC) SCORE, to provide more sophisticated and accurate risk prediction in those patients with CCTA information available, and has formed part of a regulatory cleared medical device used in clinical practice. This revised absolute risk calculator has been proposed as a tool to optimize the treatment of these patients according to the ESC guidelines on cardiovascular disease prevention in clinical practice.91,92 It has also been proposed that in patients with at least ∼2.4-fold increase of their relative risk for a fatal cardiac event (i.e. when FAI-Score in LAD or RCA >75th percentile), intense statin or other anti-inflammatory treatments may be able to reduce long-term risk.36
Although the current risk factor-based scores (like the ESC SCORE91) work well in primary prevention, their value could be markedly increased if they were combined with information from imaging that includes PVAT phenotyping and plaque characteristics. Indeed, combining perivascular FAI analysis with HRP features, identifies a group of patients at very high risk for future cardiac events (i.e. those with both high peri-coronary FAI and HRP, with ∼seven-fold increase of the 10-year risk of fatal cardiac events) and up to 11-fold increase of the risk of non-fatal myocardial infarction.87 This approach also identifies a group of intermediate/high-risk patients, with high peri-coronary FAI and no HRP (whose relative risk for fatal cardiac event over the decade is ∼5).17 Whether a FAI-based approach is superior to functional tests of myocardial ischaemia for risk stratification remains to be seen, as studies have published both negative14 and positive93 results. However, there is strong evidence that adding FAI into a model that includes risk factors, HRP, and calcium score increased the prognostic performance of the model.74 Similarly, a risk prediction model with peri-vascular FAI performed much better compared to a risk factor-based model in predicting cardiac risk.36 It is therefore important to develop prognostic models that combine classic risk factors with information on plaque and peri-vascular fat phenotyping, which can be used in clinical practice to calculate the absolute risk of a patient for a cardiac event. Because such models combine information from imaging as well as prognostic modelling, they need to meet the appropriate regulatory standards as medical devices and be cleared by the respective regulatory authorities (i.e. CE mark under the European Medical Device Regulations, Food and Drug Administration clearance for the USA, etc.), before they are implemented in clinical practice.80 The performance of such a model is expected to be significantly superior to the risk factor only-based risk stratification models, but they need to be trained against appropriate clinical cohorts with significant follow-up after their CCTA. Currently, such models can only be created for patients having CCTA as part of routine clinical care, because they can only be developed only in such cohorts of patients linked with prospective 10-year outcomes (e.g. in the CRISP-CT study74). Studies like the Swedish CArdioPulmonary bioImage Study (SCAPIS),94 linking CCTA information from primary prevention populations with outcome data, will allow the development of such prognostic models for asymptomatic individuals, enabling community screening.
Using artificial intelligence for radiotranscriptomic phenotyping of perivascular adipose tissue in risk prediction
Extraction of PVAT radiomic features and application of machine learning algorithms can generate more sophisticated biomarkers for the deep phenotyping of PVAT.13 Different types of vascular inflammation can give different imaging texture changes in PVAT, driven by variable degrees of peri-vascular lipolysis, adipogenesis, oedema, fibrosis, and angiogenesis.6 By using tissue biopsies and basic science tools (like RNA sequencing or histology) to generate the ‘ground truth’ for these changes, one can train radiomic signatures of PVAT from CCTA images to best describe the type of vascular inflammation of interest. The term ‘radiotranscriptomic’ has been introduced in cardiovascular imaging to describe the process of training radiomic signatures against the transcriptomic profile of the tissue.13 Indeed, by using AT biopsies from patients undergoing cardiac surgery and available coupled CCTA scans, machine learning algorithms were trained to identify AT inflammation, fibrosis, and vascularity from the radiomic phenotype of fat and to generate the fat radiomic profile (FRP) (Figure 5). Fat radiomic profile was subsequently tested in a cohort of 5487 participants from the CRISP-CT74 and SCOT-HEART studies81 and was able to independently predict major adverse cardiovascular events beyond traditional risk factors, coronary calcium score, coronary stenosis, and HRP features on CCTA.13 Such an approach was tested with comparable success in identifying unstable coronary plaques and predicting outcomes, in other cohorts.95–97
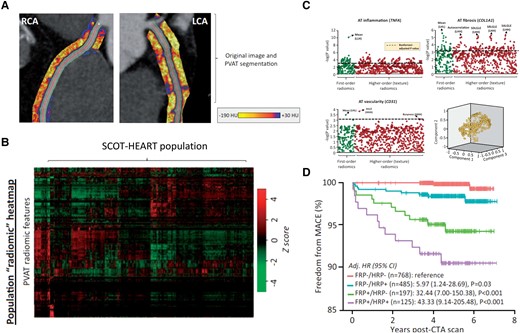
Radiomic phenotyping of coronary perivascular adipose tissue (PVAT). (A) Coronary PVAT imaging features can be used to extract a high number of shape-, attenuation-, and texture-related statistics (i.e. radiomics). (B) Heatmap of scaled radiomic features (right) of all 1391 stable radiomic features in the SCOT-HEART population (n = 1575 patients). (C) Extracted radiomic features can be tested against the transcriptome profile of PVAT to identify features informing on distinct biological processes such as adipose tissue inflammation, vascularity, and fibrosis. (D) Selected radiomic features can form distinct radiomic signatures of PVAT, in this case fat radiomic profile adipose tissue inflammation, vascularity, and fibrosis, which had strong independent predictive value for major adverse cardiac events (MACE) in the SCOT-HEART population. Obtained with permission from Oikonomou et al.13
Recently, a radiotranscriptomic signature of acute cytokine-driven vascular inflammation was trained using radiomic features of PVAT around the internal mammary arteries against RNA sequencing data extracted from the same arteries.98 That signature was then tested in patients with COVID-19, and found to change significantly during acute infection. This radiotranscriptomic signature had a striking prognostic value for in-hospital mortality in acute COVID-19, even when applied in non-gated CT angiograms of the pulmonary artery. Texture radiotranscriptomics can also be used to capture and quantify micro-circulation in the peri-vascular space, in addition to lipolysis/adipogenesis, fibrosis, and oedema, providing incremental prognostic value over FAI for cardiac events.13,98 Such machine learning/radiotranscriptomic approaches are expected to revolutionize our capacity to use PVAT as a window into vascular biology.
Perivascular adipose tissue imaging vs. circulating biomarkers of inflammation
The non-invasive detection of vascular inflammation was hailed as the ‘Holy Grail’ in the field of cardiovascular medicine.36 The detection of residual inflammatory risk is particularly relevant in the light of recent clinical evidence supporting the reduction of coronary artery disease risk by anti-inflammatory treatments.99–101 Therefore, the use of inflammatory biomarkers has been proposed as a way to guide the deployment anti-inflammatory treatments in primary and secondary prevention.99,102
Plasma biomarkers of inflammation, such as CRP or IL-6, are not specific for vascular inflammation; for example, it is estimated that approximately 50% of patients in secondary prevention have high inflammatory risk based on serum CRP levels.103 Although HRP characteristics on CCTA provide indirect information about the potential of a plaque to be inflamed, the combination of HRP with newer metrics of inflammation such as the peri-vascular FAI-Score allows for a further refinement of the risk for cardiovascular events.17,104 A recent meta-analysis has shown that CCTA-based biomarkers such as HRP or peri-coronary FAI provide much higher added prognostic value on top of clinical risk profile and atherosclerosis extent for major adverse cardiovascular events, compared to plasma biomarkers (Figure 6).105
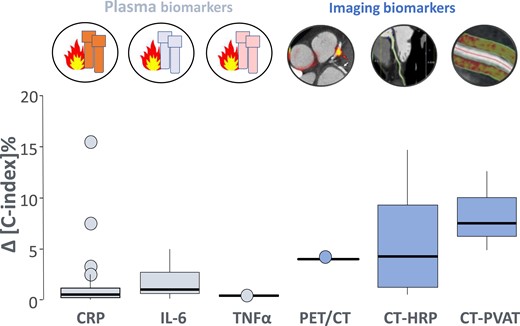
Prognostic value of vascular inflammation biomarkers for major adverse cardiovascular events. The added prognostic value of imaging biomarkers on top of patient risk profile and coronary atherosclerosis extent is greater than that of plasma biomarkers according to a meta-analysis of available published evidence from clinical studies (n = 175 778 individuals). Perivascular adipose tissue (PVAT) imaging by CT was associated with the maximum added prognostic information among the studied vascular inflammation biomarkers. Obtained with permission from Antonopoulos et al.105
Other imaging techniques to assess perivascular adipose tissue: advantages, disadvantages, and future perspectives
Other non-invasive imaging modalities such as MRI, PET, or even ultrasound could potentially add value in assessing PVAT. Indeed, MRI can quantify PVAT volume around large arteries like the aorta and this is independently associated with measures of sub-clinical atherosclerosis.106 However, MRI does not allow accurate visualization of PVAT around the coronaries. Another major limitation is the standardized image quality to allow assessment of AT quality that is required to provide between-patient comparisons. Crude measurements of the total epicardial and pericardial AT volume by MRI provides some useful information about the predictive value of visceral obesity in the general population, as demonstrated in the UK Biobank recently,107 which is however significantly lower compared to the volumetric quantification of EAT using CT.72
Positron emission tomography is another promising imaging modality that provides functional information regarding AT metabolic activity.18 Indeed, fluorodeoxyglucose (FDG) uptake in large adipose tissue depots offers useful information about the inflammatory and metabolic activity of AT.108 When PET is coupled with anatomical information from CT imaging (PET–CT), it allows better understanding of the anatomical distribution of the radiotracer uptake, particularly around large vessels like the aorta.10 However, the low spatial resolution of PET, as well as the uptake of FDG by the myocardium, do not allow reliable assessment of peri-coronary AT.10 Future improvements in the spatial resolution of the methods as well as the development of novel radiotracers that are able to identify specific metabolic or inflammatory pathways in the AT (adipocytes of AT-infiltrating inflammatory cells) could potentially transform this method into a valuable tool to assess PVAT biology.
Ultrasound may also be of some value in obtaining surrogate measurements of EAT20 but its limited ability to differentiate AT from other structures, as well as its low penetration and the operator-dependent nature of the technique, limit its value in the assessment of PVAT around the coronaries. Improvements in the post-processing of ultrasound images may change the ability of this method to assess PVAT in the future.
Finally, the advent of the revolutionary technology of photon-counting CT with its superior image resolution and capacity for tissue characterization by using subtraction techniques is also expected to open up new possibilities in the field of PVAT imaging.109 For now, there is a significant amount of technical work that needs to be done for the calibration of this technology. Indeed, the established definitions of PVAT using contemporary CT (quantitative, e.g. EAT or qualitative, e.g. FAI-Score) will need to be re-defined in an environment of changing energies (keV) and higher spatial resolution, while the diagnostic/prognostic value of radiomic features in photon-counting CT scanners will also need to be re-evaluated. Validation studies against tissue biopsies110 as well as against contemporary CT analysis of PVAT111 are ongoing, and are expected to be reported in the coming months. At present, however, photon-counting CT images are incompatible with the existing clinical tools used for the qualitative assessment of PVAT.
Future perspectives and remaining challenges
The potential benefits of targeting inflammation in the PVAT as a treatment for cardiovascular disease are theoretically significant but still unclear. Interventions that could enhance the production of adiponectin from PVAT would be expected to be protective against vascular disease.33,35 Since there are complex interactions between the vascular wall and PVAT, with multiple messengers involved in their cross-talk, temporally and spatially regulated by vascular disease development, the identification of those bioactive molecules or signalling pathways amenable to treatment remains an open challenge.
Coronary PVAT has been in the spotlight of research over the last years, but the role of PVAT in other vascular beds is less well studied. The study of peri-aortic112 or peri-femoral AT113 may provide new insights into the development, prevention, and treatment of aortic aneurysms or peripheral arterial disease, respectively.
Therapeutic interventions could be potentially deployed either by targeting PVAT itself or by using PVAT imaging as a tool of precision medicine. Indeed, the peri-coronary FAI-Score provides a regulatory cleared metric of coronary inflammation and it can be used for personalized absolute risk prediction.36 It has therefore been suggested that patients with high residual inflammatory risk identified by this approach could be candidates for anti-inflammatory strategies targeting vascular inflammation, like statins, colchicine, or other novel therapeutics.36,114
The pipeline for the validation of new imaging biomarkers must be rigorous before their clinical use. First and foremost is the technical validation of any new imaging biomarker, i.e. the repeatability and accuracy of its measurement as well as a steady diagnostic performance across platforms and acquisition parameters.72 Equally important are the issues of biological and clinical validation of any new biomarker, and the confirmation of its generalizability, and diagnostic/prognostic value in large clinical datasets.89,115
All these issues are even more pressing in the case of artificial intelligence (AI)-derived imaging biomarkers, which are typically derived out of deep learning techniques and the mining of high-dimensional data, in a ‘black-box’ process.115 The use of AI biomarkers in clinical practice requires rigorous validation and the use of very large datasets to ensure worldwide applicability, as mandated by the Medical Device Regulations in Europe and the Food and Drug Administration in the USA.
Conclusions
This Clinical Consensus Statement presents the optimum terminology for the definition of the various AT depots, and particularly consensus definitions for PVAT. It provides an overview of the range of adipokines secreted by PVAT, with either protective or detrimental effects on the cardiovascular system, which can be used as therapeutic targets for the prevention and treatment of cardiovascular diseases. Although existing pharmacotherapies affect the biology of AT, targeting PVAT specifically is more challenging, as it requires local delivery of the intervention. This position paper highlights the role of PVAT as a ‘biosensor’ of vascular inflammation and the value of recent imaging technologies that visualize PVAT around the coronary arteries and indirectly quantify the degree of coronary inflammation from routine CCTA scans, predicting future cardiovascular events. The regulatory clearance for any medical device that provides these measurements of imaging biomarkers is required before clinical applicability.
In conclusion, PVAT is currently used as a source of imaging biomarkers directly applicable in clinical practice (Graphical Abstract), and this role will be further enhanced with the advance of AI-powered image analysis in the near future. However, further research is needed before we can use PVAT as a therapeutic target in cardiovascular diseases.
Supplementary data
Supplementary data are available at European Heart Journal online.
Declarations
Disclosure of Interest
C.A. declares several patents (US10,695,023B2, PCT/GB2017/053262, GB2018/1818049.7, GR20180100490, GR20180100510) licensed to Caristo Diagnostics. C.A. is the Chair of the British Atherosclerosis Society, as well as Founder, shareholder, and director of Caristo Diagnostics, a University of Oxford Spinout company. C.A. declares past honoraria from Amarin, Silence Therapeutics, and Caristo Diagnostics. A.S.A. declares patents (PCT/GB2015/052359, PCT/GB2019/052632, PCT/GB2019/053058) licensed to Caristo Diagnostics. F.C. reports speaker fees from Amgen, Astra Zeneca, Servier, BMS, other from GlyCardial Diagnostics, outside the submitted work.
Data Availability
No data were generated or analysed for this manuscript.
Funding
C.A. declares funding from the British Heart Foundation (CH/F/21/90009 and RG/F/21/110040), and the British National Institute for Health and Care Research Oxford Biomedical Research Centre (BRC). D.J.D. was supported by the Dutch CardioVascular Alliance, an initiative with support of the Dutch Heart Foundation (Grant 2020B008 RECONNEXT) and is supported by the Netherlands CardioVascular Research Initiative: Dutch Heart Foundation, Dutch Federation of University Medical Centers, ZonMW, and Royal Netherlands Academy of Arts & Sciences (Grant CVON2017-ARENA PRIME). A.S.A. is supported by the Hellenic Foundation for Research and Innovation (Grant number 00468).