-
PDF
- Split View
-
Views
-
Cite
Cite
Lei Yu, Tianxiang Gu, Enyi Shi, Yongchao Wang, Qin Fang, Chun Wang, Dysregulation of renal microRNA expression after deep hypothermic circulatory arrest in rats, European Journal of Cardio-Thoracic Surgery, Volume 49, Issue 6, June 2016, Pages 1725–1731, https://doi.org/10.1093/ejcts/ezv460
- Share Icon Share
Abstract
Acute kidney injury (AKI) is a severe complication of cardiopulmonary bypass-deep hypothermic circulatory arrest (DHCA) surgery. Non-coding microRNAs (miRNAs) are considered as key players in kidney physiology and pathology. However, whether they are implicated in DHCA-induced AKI at the early stage post-surgery is less studied, and requires for further investigation.
In this study, kidney tissues were removed at 2 h post-surgery from Sprague-Dawley rats that underwent a 60-min DHCA (18°C), with samples from sham-operated rats as control. Renal RNA isolates were analysed with Affymetrix miRNA microarray 4.0 containing 728 mature rat miRNA probes.
Seventy-one miRNAs were down-regulated and 4 were up-regulated in the kidneys of DHCA rats [log2 (fold change, FC) > 1, P < 0.05]. Novel differentially expressed miRNAs, such as miRNA-3068, miR-1949 and miR-3473, were identified in the injured kidney tissues. Putative target genes of the down-regulated miR-30b-5p, miR-199a-5p, miR-148b-3p and miR-10a-3p were subjected to analyses of gene ontology (GO) categories and Kyoto Encyclopedia of Genes and Genomes (KEGG) pathways. The results indicated that these miRNAs targeted a large set of genes involved in essential biological processes related to AKI pathogenesis, such as apoptotic process and response to hypoxia, as well as genes implicated in critical signalling pathways, such as chemokine, lysosome and FoxO signalling pathways (false discovery rate-corrected, P < 0.05).
The identified 75 differentially expressed miRNAs hold the potential to serve as novel early markers and novel therapeutic targets for DHCA-AKI.
INTRODUCTION
Deep hypothermia has been proved as an effective technique for protecting various organs from circulatory arrest-induced ischaemic damage, and is applied in aortic arch surgery (AAS) [1]. Deep hypothermic circulatory arrest (DHCA) provokes serious side-effects, such as coagulopathy and organ dysfunction, although it renders the brain resistant to ischaemia [2]. The overall incidence of acute kidney injury (AKI) is higher in aortic surgery than in other cardiac surgeries [3, 4]. AKI is a common postoperative complication (18–55%) encountered in patients undergoing AAS under DHCA [2, 5, 6]. Though mild-to-moderate degrees of AKI are common in patients after aortic surgery, 2–8% of these patients require renal replacement therapy, and the short-term mortality is increased to 64% [5, 6]. The long-term mortality after AKI is high, even for patients with complete renal recovery [3]. In the last decade, AKI diagnosis and therapy have not notably improved probably due to the delay in diagnosis. Identification of early and novel AKI biomarkers is crucial for timely and proper treatment.
MicroRNAs (miRNAs) are a class of short non-coding RNAs that can regulate gene expression at post-transcriptional level, and the dysregulation of their expression is linked to diverse kidney disease states, including renal fibrosis, diabetic nephropathy and renal ischaemia–reperfusion injury etc. Nowadays, miRNAs are recognized as a novel type of biomarkers for a variety of diseases, and the regulation of their expression with specific antagomirs or mimics represents novel therapeutic approaches [7]. Earlier studies were mainly focused on the brain lesions after DHCA surgery [8], but not on kidney damages. Our group has previously demonstrated that 60-min DHCA surgery can initiate renal dysfunction in rabbits, and induce increases in serum creatinine (sCr), blood urea nitrogen (BUN), serum cystatin C and neutrophil gelatinase-associated lipocalin (NGAL) [9]. However, these traditional biomarkers for AKI are increased slowly in response to renal damage (at 12 or 24 h post-surgery), possibly leading to a diagnostic delay. Interestingly, while cardiopulmonary bypass (CPB) alone yielded no neurological injury in dogs, CPB with DHCA caused severe neurological damage in canine brains at 8 h post-surgery and changed cerebral gene expression [10]. Such findings suggest that the cerebral gene expression in animals can be modified after a short period of DHCA surgery (<12 h). Regarding the pivotal roles of miRNAs in regulating their target genes, our study was performed to investigate whether miRNA expression patterns in kidneys were affected by DHCA at the early stage post-surgery.
In this study, microarray analysis was utilized to assess the miRNA expression levels in rat kidney tissues. The microarray used in our study is miRNA microarray 4.0, which is designed to interrogate all mature miRNA sequences in miRBase Release 20.0 (http://mirbase.org/ftp.shtml). A total of 728 mature miRNA sequences of rats are listed in miRBase release 20.0, which are substantially more than that of pigs (326) and dogs (291). In addition, rats are widely used as an experimental model to study the pathogenesis of kidney diseases, such as AKI. Therefore, to gain a comprehensive understanding on how DHCA surgery affected renal miRNA expression in vivo, CPB with 60-min DHCA procedure was performed in rats, and their kidney tissues were removed for microarray analysis. We here for the first time found aberrant renal miRNA expression patterns in DHCA rats and further discussed whether the differentially expressed miRNAs were linked to DHCA-AKI pathogenesis.
MATERIALS AND METHODS
Animals
Male Sprague-Dawley rats weighing ∼350 g were obtained from the Experimental Animal Center of China Medical University (Shenyang, China). The animal experimental procedures have been approved by the Ethics Review Committee for Animal Experimentation of China Medical University.
Establishment of rat model of cardiopulmonary bypass and deep hypothermic circulatory arrest
A total of 36 rats were subjected to CPB with 60 min of DHCA (DHCA group) according to the protocols in our laboratory (n = 18 per group) [11]. In short, the rats were anaesthetized with chloral hydrate and isoflurane and maintained with 2–2.5% isoflurane and 25 mg/kg fentanyl (intravenous). These animals were orotracheally intubated with a 14G cannula, ventilated with a tidal volume of 10 ml/kg and a respiratory rate of 60–65 beats min−1 and given a systemic heparinization (150 IU). Then, the right carotid artery was cannulated, and a multistaged venous return cannula was placed next to the junction of the inferior vena cava and right atrium through the right external jugular vein.
The CPB circuit included a venous reservoir, a peristaltic pump and a custom-designed small-volume oxygenator. CPB was instituted at a flow rate of 160–180 ml kg−1 min−1 and then decreased by half during the cooling period. By using a heat exchanger, a cooling blanket and ice bags, rats were cooled to a pericranial temperature of 18°C over 30 min. Thereafter, the CPB was stopped, and venous blood was drained into the reservoir. The left common carotid artery was catheterized distally for anterograde cerebral perfusion. The distal aspect of the right common carotid artery and the proximal aspect of the left common carotid artery were clamped during systemic DHCA and antegrade cerebral perfusion via the left carotid catheter. The absence of systolic and measurable blood pressure confirmed the successful establishment of rat CPB + DHCA model. Sixty minutes later, the CPB was reinstituted (160–180 ml kg−1 min−1). Rats were re-warmed to a pericranial temperature of 34°C over 30 min, and then CPB was stopped. Then, these rats were maintained with 0.5–1.2% isoflurane, intubated and ventilated for additional 2 h, and gradually re-warmed to a pericranial temperature of 36–37°C. After spontaneous ventilation had resumed, animals were extubated and recovered in an oxygen-enriched environment for 12 h. Rats underwent the same anaesthetic management without CPB and DHCA were used as control (Sham group).
Sampling and assessment of rat renal functions
Blood samples were collected from the tail artery at 2 and 24 h after surgery. Half animals in each group were sacrificed at 2 h after surgery, and the kidney tissues were removed, and three tissue samples were used for micoRNA microarray analysis. The rest rats were sacrificed at 24 h after surgery, and kidney tissues were also collected. Contents of serum cystatin C and serum/tissue NGAL were assessed with commercial kits (Jiancheng Bioengineering Institute, Nanjing, China). Wherever appropriate, data were subjected to un-paired t-test. No statistical calculation was performed in determining the cohort size.
MicroRNAs microarray procedures
Affymetrix GeneChip miRNA 4.0 array (Affymetrix, Santa Clara, CA, USA) containing 728 mature rat miRNA probes was used to detect miRNA expression levels. In brief, RNA samples were isolated from rat kidney tissues, quantified on NanoDrop ND-2100 (Thermo Scientific, Pittsburgh, PA, USA), and assessed on Agilent 2100 (Agilent Technologies, Santa Clara, CA, USA). After being tailed with poly A and labelled with biotin, RNA samples were hybridized onto the microarrays according to the manufacturer's standard protocols. Affymetrix Scanner 3000 (Affymetrix) was used to scan the microarrays.
Data analysis
Microarray raw data were first analysed by Affymetrix GeneChip Command Console software (Version 4.0, Affymetrix). After robust multiarray average, data were analysed with GeneSpring software (Version 12.5; Agilent Technologies). The principal component analysis (PCA) algorithm was run to reduce data dimensionality. FC was calculated by comparing the expression levels of a certain miRNA in DHCA group with that of Sham group. MiRNAs with absolute log2 (FC) > 1 and P-value <0.05 (unpaired t-test) were considered as differentially expressed miRNAs. All dysregulated miRNAs were subjected to hierarchical clustering analysis, and the results were exhibited in a heat map. TargetScan and microRNAorg were used to predict putative targets of certain miRNAs. Functional analyses including gene ontology (GO) and Kyoto Encyclopedia of Genes and Genomes (KEGG) annotations were performed in putative target genes.
Quantitative real-time polymerase chain reaction and western blot analysis
To confirm the accuracy of microarray data, quantitative real-time polymerase chain reaction (qRT-PCR) analysis was performed by using the same tissue samples used in the microarray assay. The miRNA expression levels were determined on Exicycler™ 96, and calculated with 2−ΔCt (Ct, threshold cycle) [12] (n = 3). The expression levels of several putative target genes were also assessed in this study (n = 3). All the primer sequences could be found in Supplementary Table 1. U6 was used as an endogenous control for miRNAs; β-actin for genes. Protein expression levels of phosphatase and tensin homolog (PTEN) were further determined with western blot analysis (n = 8) by using polyclonal antibodies against them (1:1000 dilution; Boster, Wuhan, China).
RESULTS
Cardiopulmonary bypass with deep hypothermic circulatory arrest-induced renal dysfunction in rats
Two rats were excluded from each group because of death during the surgical process. Half of the rest rats were sacrificed at 2 h and half at 24 h post-surgery (n = 8/group/time point). The haemodynamic and physiological indices of rats were monitored (data not shown). Serum cystatin C of DHCA group was increased by 2.73-fold at 24 h post-surgery versus sham groups, whereas NGAL was increased by 2.88-fold in serum and 2.78-fold in kidney tissues (Fig. 1). No significant alterations were observed at 2 h between the two groups (All P > 0.05) (Fig. 1). Collectively, renal dysfunction in rats after DHCA was confirmed by the increased serum cystatin C and serum/kidney NGAL at 24 h post-surgery.
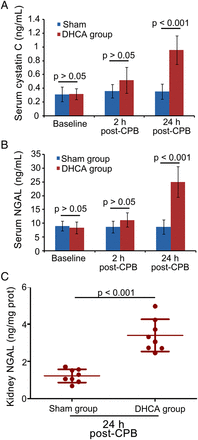
Effects of DHCA on renal functions. (A) Serum cystatin C and (B) NGAL levels were detected at 2 and 24 h post-surgery (n = 8). (C) NGAL levels in kidney tissues were determined at 24 h post-surgery (n = 8). DHCA: deep hypothermic circulatory arrest; NGAL: neutrophil gelatinase-associated lipocalin; CPB: cardiopulmonary bypass.
Renal microRNA expression profiling in deep hypothermic circulatory arrest rats
Renal miRNA expression patterns at 2 h post-surgery (n = 3/group) were assessed with Affymetrix microarrays. The complete data of rno-miRNAs were first analysed through PCA algorithm. As shown in the coordinate system, the six samples were classified into two distinct groups, revealing the appropriate animal grouping (Fig. 2A).
![Results of PCA, volcano plot and heat maps of the differentially expressed renal miRNAs in rats after DHCA surgery. (A) Normalized microarray results were analysed with PCA algorithm, and (B) the analysed miRNAs were plotted in a volcano plot based on the FC and P-value. A blue or red dot represented an miRNA with an absolute log2 (FC) > 1 and P < 0.05. Unsupervised hierarchical cluster analysis was performed in miRNAs with dysregulated expression, and the results are shown in heat maps [C for miRNAs with FC > 1 and P < 0.05; D for miRNAs with log2 (FC) > 1 and P < 0.05]. Each row represented a miRNA and each column represented a sample. Differential expression levels were illustrated by the pseudocolour. Red colour indicated the transcript levels higher than the median; green, lower; black, equal. The miRNA clustering tree could be found on the left. PCA: principal component analysis ; DHCA: deep hypothermic circulatory arrest; FC: fold change.](https://oup.silverchair-cdn.com/oup/backfile/Content_public/Journal/ejcts/49/6/10.1093_ejcts_ezv460/1/m_ezv46002.gif?Expires=1750454596&Signature=nlc67tUzTmiz80IiZTLvo4DD7hTvYu8seAFxLjd5GHaq21UcQ74vNe7bKGSouMa9JL-A9NqGiRlXp1RnDe-o6V65XUTOcybf7ksLvAG0r7jZA0ZqVzc9cwlXNopuoWgplygmDGseEjsjQrhA9mYnEAvUpzbA8pfW8IRgQsdfdTaSb6EqbLK~dJw8OQGFlQOmSScwbxn6iay8TUBsubBdm2QP3Mm8FkiMzriIDyTwd5Mo5ifQxysLyk1iuRt1FJ32NNyQ1dAo9NfD6VqJAuIKzTifX2Xs2XkfxMeJ17Qa6kJ49Cb5~B9N8iYV8Tdd3FvFTIAo~zlbQh77ZjndNGnF5Q__&Key-Pair-Id=APKAIE5G5CRDK6RD3PGA)
Results of PCA, volcano plot and heat maps of the differentially expressed renal miRNAs in rats after DHCA surgery. (A) Normalized microarray results were analysed with PCA algorithm, and (B) the analysed miRNAs were plotted in a volcano plot based on the FC and P-value. A blue or red dot represented an miRNA with an absolute log2 (FC) > 1 and P < 0.05. Unsupervised hierarchical cluster analysis was performed in miRNAs with dysregulated expression, and the results are shown in heat maps [C for miRNAs with FC > 1 and P < 0.05; D for miRNAs with log2 (FC) > 1 and P < 0.05]. Each row represented a miRNA and each column represented a sample. Differential expression levels were illustrated by the pseudocolour. Red colour indicated the transcript levels higher than the median; green, lower; black, equal. The miRNA clustering tree could be found on the left. PCA: principal component analysis ; DHCA: deep hypothermic circulatory arrest; FC: fold change.
All analysed miRNAs were visually presented in a volcano plot (Fig. 2B). When compared with the sham group, 126 of 728 miRNAs had aberrant expression (P < 0.05), and 75 with log2 (FC) > 1 were considered as differentially expressed. Then, the miRNAs differing in expression were subjected to unsupervised hierarchical cluster analysis, and the data were presented in two heat maps (Fig. 2C for 126 miRNAs and Fig. 2D for 75). We noted that samples obtained from rats that underwent the same surgical procedure had similar miRNA expression patterns. Detailed data of the 75 miRNAs are summarized in Supplementary Table 2. Among the differentially expressed miRNAs, 71 miRNAs were down-regulated [40 had a log2 (FC) > 2] and 4 were up-regulated.
Validation of the microarray data in selected microRNA through quantitative real-time polymerase chain reaction
Twelve miRNAs (miR-382-5p, miR-134-5p, miR-10a-3p, miR-29c-3p, miR-148b-3p, miR-29b-3p, 106b-5p, miR-199a-5p, miR-181c-5p and miR-30b-5p) out of the 40 significantly down-regulated miRNAs [log2 (FC) > 2] were subjected to qRT-PCR analysis. The expression levels of two up-regulated miRNAs (miR-155-5p and miR-1224) were detected as well. Results obtained from qRT-PCR analysis were in accordance with microarray data (Fig. 3A).

Validation of differentially expressed miRNAs and their target genes. The expression levels of the indicated miRNAs (A) and genes (B) were assessed with qRT-PCR method (n = 3). (C) The expression levels of PTEN were determined with western blot analysis (n = 8). U6 was used as an endogenous control for miRNAs; β-actin for genes. miRNAs: microRNAs; DHCA: deep hypothermic circulatory arrest; FC: fold change; qRT-PCR: quantitative real-time polymerase chain reaction.
The expression levels of several putative genes implicated in ischaemic injuries were further determined with qRT-PCR analysis. Significant expression alterations of DNMT3a, PTEN, BCL2L14, BECN1, SOD2 and tumor necrosis factor (TNF)-α were observed (Fig. 3B). Moreover, PTEN protein expression was further validated by western blot assay in all rats sacrificed at 2 h post-CPB. An up-regulation of PTEN protein expression was confirmed (Fig. 3C).
Functional enrichment analysis of putative target genes
In the validated 10 down-regulated miRNAs, miR-10a-3p, miR-30b-5p, miR-148b-3p and miR-199a-5p had a log2 (qRT-PCR FC) > 1 and P-value <0.05. Therefore, target prediction was performed in these miRNAs with TargetScan and microRNAorg (see Supplementary Table 3). The obtained genes were then subjected to KEGG pathway analysis and functional enrichment analysis within biological process GO category. Many putative genes were enriched in biological processes or signalling pathways related to ischaemic kidney injuries, such as the response to hypoxia, apoptotic process, negative regulation of apoptotic process, chemokine pathway and FoxO signalling pathway etc. (Table 1). All KEGG pathway items with a false discovery rate (FDR) corrected P-value <0.05 are given in Table 1, whereas the complete data for GO items are summarized in Supplementary Table 4.
Term description . | List hits . | Involved genes . | P-value . | FDR-corrected P-value . |
---|---|---|---|---|
GO terms | ||||
Transcription, DNA-templated | 55 | YBX1, HIF-1α, SMAD1, SMAD3, STAT1, STAT2, DMTF1 etc. | 9.3E−05 | 0.017 |
Negative regulation of apoptotic process | 46 | YBX1, YBX3, TGF-α, TGF-β2, HIF-1α, CDK1, CDKN1B, SMAD3 etc. | 9.1E−07 | 0.002 |
Response to drug | 43 | SOD2, HIF-1α, BECN1, LPL, FZD1 etc. | 1.1E−05 | 0.006 |
Positive regulation of cell proliferation | 38 | PDGFc, STAT1, PTK-2β, TIMP1, CTGF etc. | 2.0E−04 | 0.025 |
Response to organic cyclic compound | 30 | DUSP6, AACS, CASR, PCSK1, TAF9B etc. | 1.6E−06 | 0.002 |
Protein transport | 28 | ARF4, CALCR, NUPL1, RAP1A, TMED3 etc. | 3.2E−06 | 0.002 |
Apoptotic process | 27 | TP63, Bcl2l14, CDK1, MAP3K8, AKTIP etc. | 4.1E−04 | 0.037 |
Response to lipopolysaccharide | 25 | SOD2, ATP4β, CXCL9, PCSK1, ALPL etc. | 6.3E−05 | 0.015 |
Intracellular protein transport | 24 | SNX27, BCAP29, KPNB1, AP3M1 etc. | 2.2E−04 | 0.026 |
Response to hypoxia | 24 | HIF-1α, SOD2, TGF-β2, ADAM17, BECN1 etc. | 3.8E−04 | 0.036 |
Positive regulation of cell migration | 21 | SNAI1, SMAD3, CXCL10, CXCL12, ETS1 etc. | 1.7E−05 | 0.006 |
KEGG pathways | ||||
Chemokine signalling pathway | 24 | CXCL9/10/11/12/13, CXCR2/3, CCR1 etc. | 2.6E−05 | 0.004 |
Lysosome | 19 | CTSK, CTSE, LGMN, GM2A, TPP1, ACP2 etc. | 5.9E−05 | 0.004 |
FoxO signalling pathway | 19 | FoxG1, IRS1, PIK3R2, GLUT4, PRKAG2, GADD45α, SOD2 etc. | 1.1E−04 | 0.006 |
GABAergic synapse | 16 | GABA receptors, CACNα1B, PRKCγ etc. | 3.5E−05 | 0.004 |
Morphine addiction | 15 | GABA receptors, PDE1C, PRKCγ etc. | 1.4E−04 | 0.006 |
Retrograde endocannabinoid signalling | 15 | GABA receptors, vGLUT2, PRKCγ etc. | 4.3E−04 | 0.014 |
Circadian rhythm | 9 | BTRC, BHLHE40, RBX1, PER2, SKP1 etc. | 6.1E−05 | 0.004 |
Nicotine addiction | 9 | GABA receptors, vGLUT2, GRIN2A etc. | 3.9E−04 | 0.014 |
Term description . | List hits . | Involved genes . | P-value . | FDR-corrected P-value . |
---|---|---|---|---|
GO terms | ||||
Transcription, DNA-templated | 55 | YBX1, HIF-1α, SMAD1, SMAD3, STAT1, STAT2, DMTF1 etc. | 9.3E−05 | 0.017 |
Negative regulation of apoptotic process | 46 | YBX1, YBX3, TGF-α, TGF-β2, HIF-1α, CDK1, CDKN1B, SMAD3 etc. | 9.1E−07 | 0.002 |
Response to drug | 43 | SOD2, HIF-1α, BECN1, LPL, FZD1 etc. | 1.1E−05 | 0.006 |
Positive regulation of cell proliferation | 38 | PDGFc, STAT1, PTK-2β, TIMP1, CTGF etc. | 2.0E−04 | 0.025 |
Response to organic cyclic compound | 30 | DUSP6, AACS, CASR, PCSK1, TAF9B etc. | 1.6E−06 | 0.002 |
Protein transport | 28 | ARF4, CALCR, NUPL1, RAP1A, TMED3 etc. | 3.2E−06 | 0.002 |
Apoptotic process | 27 | TP63, Bcl2l14, CDK1, MAP3K8, AKTIP etc. | 4.1E−04 | 0.037 |
Response to lipopolysaccharide | 25 | SOD2, ATP4β, CXCL9, PCSK1, ALPL etc. | 6.3E−05 | 0.015 |
Intracellular protein transport | 24 | SNX27, BCAP29, KPNB1, AP3M1 etc. | 2.2E−04 | 0.026 |
Response to hypoxia | 24 | HIF-1α, SOD2, TGF-β2, ADAM17, BECN1 etc. | 3.8E−04 | 0.036 |
Positive regulation of cell migration | 21 | SNAI1, SMAD3, CXCL10, CXCL12, ETS1 etc. | 1.7E−05 | 0.006 |
KEGG pathways | ||||
Chemokine signalling pathway | 24 | CXCL9/10/11/12/13, CXCR2/3, CCR1 etc. | 2.6E−05 | 0.004 |
Lysosome | 19 | CTSK, CTSE, LGMN, GM2A, TPP1, ACP2 etc. | 5.9E−05 | 0.004 |
FoxO signalling pathway | 19 | FoxG1, IRS1, PIK3R2, GLUT4, PRKAG2, GADD45α, SOD2 etc. | 1.1E−04 | 0.006 |
GABAergic synapse | 16 | GABA receptors, CACNα1B, PRKCγ etc. | 3.5E−05 | 0.004 |
Morphine addiction | 15 | GABA receptors, PDE1C, PRKCγ etc. | 1.4E−04 | 0.006 |
Retrograde endocannabinoid signalling | 15 | GABA receptors, vGLUT2, PRKCγ etc. | 4.3E−04 | 0.014 |
Circadian rhythm | 9 | BTRC, BHLHE40, RBX1, PER2, SKP1 etc. | 6.1E−05 | 0.004 |
Nicotine addiction | 9 | GABA receptors, vGLUT2, GRIN2A etc. | 3.9E−04 | 0.014 |
FDR-corrected P-value, P-values are corrected by FDR Benjamini–Hochberg method. List hits, the number of putative genes that belong to the indicated term. GO items with list hits >20 are present in the table.
GO: gene ontology.
Term description . | List hits . | Involved genes . | P-value . | FDR-corrected P-value . |
---|---|---|---|---|
GO terms | ||||
Transcription, DNA-templated | 55 | YBX1, HIF-1α, SMAD1, SMAD3, STAT1, STAT2, DMTF1 etc. | 9.3E−05 | 0.017 |
Negative regulation of apoptotic process | 46 | YBX1, YBX3, TGF-α, TGF-β2, HIF-1α, CDK1, CDKN1B, SMAD3 etc. | 9.1E−07 | 0.002 |
Response to drug | 43 | SOD2, HIF-1α, BECN1, LPL, FZD1 etc. | 1.1E−05 | 0.006 |
Positive regulation of cell proliferation | 38 | PDGFc, STAT1, PTK-2β, TIMP1, CTGF etc. | 2.0E−04 | 0.025 |
Response to organic cyclic compound | 30 | DUSP6, AACS, CASR, PCSK1, TAF9B etc. | 1.6E−06 | 0.002 |
Protein transport | 28 | ARF4, CALCR, NUPL1, RAP1A, TMED3 etc. | 3.2E−06 | 0.002 |
Apoptotic process | 27 | TP63, Bcl2l14, CDK1, MAP3K8, AKTIP etc. | 4.1E−04 | 0.037 |
Response to lipopolysaccharide | 25 | SOD2, ATP4β, CXCL9, PCSK1, ALPL etc. | 6.3E−05 | 0.015 |
Intracellular protein transport | 24 | SNX27, BCAP29, KPNB1, AP3M1 etc. | 2.2E−04 | 0.026 |
Response to hypoxia | 24 | HIF-1α, SOD2, TGF-β2, ADAM17, BECN1 etc. | 3.8E−04 | 0.036 |
Positive regulation of cell migration | 21 | SNAI1, SMAD3, CXCL10, CXCL12, ETS1 etc. | 1.7E−05 | 0.006 |
KEGG pathways | ||||
Chemokine signalling pathway | 24 | CXCL9/10/11/12/13, CXCR2/3, CCR1 etc. | 2.6E−05 | 0.004 |
Lysosome | 19 | CTSK, CTSE, LGMN, GM2A, TPP1, ACP2 etc. | 5.9E−05 | 0.004 |
FoxO signalling pathway | 19 | FoxG1, IRS1, PIK3R2, GLUT4, PRKAG2, GADD45α, SOD2 etc. | 1.1E−04 | 0.006 |
GABAergic synapse | 16 | GABA receptors, CACNα1B, PRKCγ etc. | 3.5E−05 | 0.004 |
Morphine addiction | 15 | GABA receptors, PDE1C, PRKCγ etc. | 1.4E−04 | 0.006 |
Retrograde endocannabinoid signalling | 15 | GABA receptors, vGLUT2, PRKCγ etc. | 4.3E−04 | 0.014 |
Circadian rhythm | 9 | BTRC, BHLHE40, RBX1, PER2, SKP1 etc. | 6.1E−05 | 0.004 |
Nicotine addiction | 9 | GABA receptors, vGLUT2, GRIN2A etc. | 3.9E−04 | 0.014 |
Term description . | List hits . | Involved genes . | P-value . | FDR-corrected P-value . |
---|---|---|---|---|
GO terms | ||||
Transcription, DNA-templated | 55 | YBX1, HIF-1α, SMAD1, SMAD3, STAT1, STAT2, DMTF1 etc. | 9.3E−05 | 0.017 |
Negative regulation of apoptotic process | 46 | YBX1, YBX3, TGF-α, TGF-β2, HIF-1α, CDK1, CDKN1B, SMAD3 etc. | 9.1E−07 | 0.002 |
Response to drug | 43 | SOD2, HIF-1α, BECN1, LPL, FZD1 etc. | 1.1E−05 | 0.006 |
Positive regulation of cell proliferation | 38 | PDGFc, STAT1, PTK-2β, TIMP1, CTGF etc. | 2.0E−04 | 0.025 |
Response to organic cyclic compound | 30 | DUSP6, AACS, CASR, PCSK1, TAF9B etc. | 1.6E−06 | 0.002 |
Protein transport | 28 | ARF4, CALCR, NUPL1, RAP1A, TMED3 etc. | 3.2E−06 | 0.002 |
Apoptotic process | 27 | TP63, Bcl2l14, CDK1, MAP3K8, AKTIP etc. | 4.1E−04 | 0.037 |
Response to lipopolysaccharide | 25 | SOD2, ATP4β, CXCL9, PCSK1, ALPL etc. | 6.3E−05 | 0.015 |
Intracellular protein transport | 24 | SNX27, BCAP29, KPNB1, AP3M1 etc. | 2.2E−04 | 0.026 |
Response to hypoxia | 24 | HIF-1α, SOD2, TGF-β2, ADAM17, BECN1 etc. | 3.8E−04 | 0.036 |
Positive regulation of cell migration | 21 | SNAI1, SMAD3, CXCL10, CXCL12, ETS1 etc. | 1.7E−05 | 0.006 |
KEGG pathways | ||||
Chemokine signalling pathway | 24 | CXCL9/10/11/12/13, CXCR2/3, CCR1 etc. | 2.6E−05 | 0.004 |
Lysosome | 19 | CTSK, CTSE, LGMN, GM2A, TPP1, ACP2 etc. | 5.9E−05 | 0.004 |
FoxO signalling pathway | 19 | FoxG1, IRS1, PIK3R2, GLUT4, PRKAG2, GADD45α, SOD2 etc. | 1.1E−04 | 0.006 |
GABAergic synapse | 16 | GABA receptors, CACNα1B, PRKCγ etc. | 3.5E−05 | 0.004 |
Morphine addiction | 15 | GABA receptors, PDE1C, PRKCγ etc. | 1.4E−04 | 0.006 |
Retrograde endocannabinoid signalling | 15 | GABA receptors, vGLUT2, PRKCγ etc. | 4.3E−04 | 0.014 |
Circadian rhythm | 9 | BTRC, BHLHE40, RBX1, PER2, SKP1 etc. | 6.1E−05 | 0.004 |
Nicotine addiction | 9 | GABA receptors, vGLUT2, GRIN2A etc. | 3.9E−04 | 0.014 |
FDR-corrected P-value, P-values are corrected by FDR Benjamini–Hochberg method. List hits, the number of putative genes that belong to the indicated term. GO items with list hits >20 are present in the table.
GO: gene ontology.
DISCUSSION
It has been reported that AKI is associated with the increased postoperative mortality after cardiothoracic surgeries, such as AAS [5, 6]. There are two possible mechanisms for the development of AKI during AAS under DHCA: one is ischaemic injury [13], and the other is atheroembolic burden [14]. Despite great advances achieved in prevention strategies and patient classification, no efficient therapy is available once AKI occurs. sCr (a traditional marker for kidney dysfunction) has limitations for assessing glomerular rate filtration because its level can be modified by drugs. Novel biomarkers such as NGAL or cystatin C did not exhibit the diagnostic value expected in AKI populations. We have previously found that, in rabbits, serum cystatin C and NGAL increased at 12 h post-DHCA, whereas sCr and BUN increased at 24 h [9]. These findings suggested that the former two indices were more sensitive than the latter two. Thus, the rat kidney functions were first evaluated by analysing serum and/or kidney cystatin C and NGAL levels in this study. We observed significant elevation of cystatin C and NGAL at 24 h post-surgery in DHCA rats, but failed to detect significant changes at 2 h. Therefore, in order to improve AKI diagnosis and patient management, there is an urgent need to identify early biomarkers for DHCA-related AKI.
Regulating the expression of a certain miRNA represents novel therapeutic approaches for various diseases, and we are currently doing research in this field. In earlier studies performed in our laboratory, lentiviruses containing antisense oligonucleotides against miR-29c [11] or miR-320 [15] were directly administrated in the injured areas (intracerebroventricular or intrathecal injection) in rats previously to prolonged DHCA or ischaemia–reperfusion surgery. The two anti-miRNAs were found to alleviate the neurological damage. To initiate miRNA-based therapies in DHCA-AKI, identification of miRNAs implicated in DHCA-induced renal lesions is pivotal. By using miRNA microarrays, we here found that 71 miRNAs were down-regulated and 4 were up-regulated in the kidney tissues from DHCA rats. These data helped us to understand the DHCA-induced changes in the kidney at the molecular level.
PCA algorithm can decompose the raw data obtained from array analysis by a matrix technique, reducing data dimensionality [16]. Samples from similar conditions or miRNAs expressed in a coordinated manner appear in a cluster after unsupervised hierarchical cluster analysis. In this study, results from PCA and cluster analyses (heat map) revealed that the experimental animals that underwent the same surgical procedure were classified into one group, and that the miRNAs with similar expression pattern were clustered. Some of the differentially expressed miRNAs have been reported to be implicated in ischaemic kidney injury before, such as miR-187-3p, miR-192-3p, miR-199a-3p, miR-29s and miR-1224, whereas some of them were found as novel dysregulated miRNAs, such as miRNA-3068, miR-1949 and miR-3473. To our best knowledge, our study was the first to demonstrate a dysregulation in renal miRNAs at the early stage post-DHCA surgery.
DHCA surgery induces ischaemia-related damages in kidney to different extent, although it reduces the renal metabolism by lowering the demand for glucose and suppressing the hypoxic effects. MiRNAs can silence the expression of their target mRNAs and prevent protein production. By using miRNA target prediction databases, genes potentially regulated by the differentially expressed miRNAs were identified in the present study. Those implicated in ischaemic injury-related apoptosis (PTEN, BCL2L14), gene methylation (DNMT3a), inflammation (TNFα), oxidative stress response (SOD2) and autophagy (BECN1) and their corresponding regulatory miRNAs were chosen as our study focus. PTEN is a well-known tumour suppressor and can inhibit the phosphorylated serine-threonine kinase (AKT), leading to cell apoptosis or death [17]. Renal PTEN is decreased in animals with nephrectomy-induced AKI [18], but oppositely increased in those with rhabdomyolysis-induced AKI [19], revealing that different stimuli may evoke discrepant responses in PTEN in the kidney. Reportedly, de-repression of DNMT3a (a DNA methyltransferase) deteriorates ischaemic brain damages [20], and TNFα (a pro-inflammatory cytokine) contributes significantly to ischaemic inflammatory response. Our study here showed increases in renal PTEN, DNMT3a and TNFα in rats that underwent DHCA. Though PTEN, DNMT3a and TNFα have been demonstrated as the direct target for miR-106b [21], miR-29c [20] and miR-181s, respectively, whether their quick increases were attributed to the reduction of these miRNAs requires further investigation. Moreover, the antioxidant SOD2 [22] had a decreased mRNA expression, although it was predicted as a target for the down-regulated miR-148b-3p. BECN1 gene encoding the beclin-1 protein is a key regulator in autophagic pathway [23] and has been predicted as a putative target for the down-regulated miR-30b-5p (Position: 236–243) and the up-regulated miR-1224 (Position: 118–124 and 552–558). MiRNA-30a sharing the same seed sequence with miRNA-30b-5p targets BECN1 mRNA, and its down-regulation mitigates cerebral ischaemic injury by promoting beclin 1-mediated autophagy [24]. No evidence has demonstrated the regulatory effects of miR-1224 on BECN1, we therefore proposed that renal BECN1 expression was up-regulated. Unexpectedly, BECN1 expression was decreased in DHCA rats. Such novel findings encouraged us to investigate the correlation between dysregulated miR-1224 and BECN1-related autophagy in AKI.
Since a large portion of differentially expressed miRNAs were down-regulated in DHCA rat kidneys, we next performed bioinformatics analysis with an emphasis on the down-regulated miRNAs. In the validated 10 down-regulated miRNAs, miR-10a-3p, miR-30b-5p, miR-148b-3p and miR-199a-5p had log2 (qRT-PCR FC) > 1 and P-value <0.05. Therefore, their putative targets were subjected to bioinformatics analysis. We found that the predicted genes were involved in renal pathological changes-related apoptotic process, negative regulation of apoptotic process, response to hypoxia, chemokine, lysosome-dependent autophagy and FoxO signalling pathways [25]. The disparity among species might limit the application of the obtained results from rats to human beings. Nevertheless, we gained interesting insights into miRNA dysregulation-related pathogenesis of DHCA-AKI based on the sequence conservation of miRNAs and genes.
Our study was aimed at identifying miRNAs with the potential to serve as early markers and therapeutic targets for DHCA-AKI. Although beyond the scope of this paper, it will be interesting to perform functional studies on these DHCA-related miRNAs. There are several challenges that hamper the development of miRNA-based therapies in kidney injuries. One is how to deliver the oligonucleotides effectively into the kidney. It has been reported that synthesized miRNA antagomirs or mimics can be administered in experimental animals through subcutaneous or intraperitoneal injection before inducing damages in kidney and subsequently mitigate kidney injuries. Mesenchymal stem cells are also suggested as a promising miRNA delivery platform. As a matter of fact, the delivery means, time and doses of miRNAs or anti-miRNAs in experimental animals are currently being evaluated in laboratory. Moreover, determining the expression patterns of the identified candidate miRNAs in easily accessible body fluids, such as plasma and urine, will help to evaluate their potential as clinical biomarkers for DHCA-AKI.
In conclusion, a dysregulated expression profile of renal miRNAs (containing 71 down-regulated and 4 up-regulated miRNAs) is obtained from rats that underwent DHCA at the early stage post-surgery. The identified differentially expressed miRNAs hold the potential to serve as novel early markers and become novel therapeutic targets for DHCA-AKI.
SUPPLEMENTARY MATERIAL
Supplementary Material is available at EJCTS online.
Conflict of interest: none declared.
REFERENCES