-
PDF
- Split View
-
Views
-
Cite
Cite
Luca Ampollini, Denise Madeddu, Angela Falco, Caterina Frati, Bruno Lorusso, Gallia Graiani, Francesca Saccani, Andrea Gervasi, Pietro Rossetti, Sabrina Bonomini, Letizia Gnetti, Costanza Annamaria Lagrasta, Enrico Maria Silini, Eugenio Quaini, Piergiorgio Petronini, Roberta Alfieri, Michele Rusca, Paolo Carbognani, Federico Quaini, Lung mesenchymal cells function as an inductive microenvironment for human lung cancer propagating cells, European Journal of Cardio-Thoracic Surgery, Volume 46, Issue 6, December 2014, Pages e103–e112, https://doi.org/10.1093/ejcts/ezu359
- Share Icon Share
The aim of the present study was to characterize the biological properties and in vivo tumourigenic potential of mesenchymal cells (MCs) obtained from non-small-cell lung cancer (NSCLC) samples.
NSCLC samples (53 adenocarcinomas and 24 squamous-cell carcinomas) surgically removed from 46 males and 31 females were processed to identify mesenchymal cells from human lung cancer (hLc-MCs). hLc-MCs were separated from neoplastic epithelial cells, expanded and extensively characterized in vitro. Subsequently, female BALB/c nude mice were subcutaneously injected with either 106 or 2.5 × 106 Calu-3 (human adenocarcinoma cell line able to reproducibly induce xenografted tumours) alone or in combination with equal doses of hLc-MCs. Control animals were injected with the two doses of hLc-MCs only.
Primary cultures of hLc-MCs were obtained from >80% of NSCLC specimens. The typical MCs immunophenotype was documented by the expression of CD90, CD105, CD73, CD13 and CD44 at fluorescence-activated cell sorting analysis. CD45, CD14, CD34 and epithelial antigens were negative while CD117 (c-kit) and CD133 (prominin) were partially expressed. Interestingly, nuclear transcription factors octamer-binding transcription factor 3/4 and sex determining region Y-box 2 involved in stemness, thyroid transcription factor 1 in bronchoalveolar commitment, and ETS1 in carcinogenesis, were expressed in hLc-MCs isolated from NSCLC. Specific conditioned media and cocultures confirmed the supportive role of hLc-MCs for cancer cells. In vivo experiments showed that at both doses Calu-3 xenografts doubled in size when hLc-MCs were coinjected. Cell tracking in xenografted tumours, by immunofluorescence combined with fluorescence in situ hybridization analysis, documented hX-chromosome-labelled, Calu-3-derived cytokeratin-positive adenocarcinoma structures surrounded by hLc-MCs.
Tumour-propagating cells require the inductive interaction of resident mesenchymal cells to foster lung cancer development.
INTRODUCTION
Current treatments of lung cancer are strongly limited by the lack of knowledge on the biology and natural history of this specific tumour. Current proposed therapies have not been able to produce robust positive results on survival, or to prevent relapse, with rapid disease progression in patients with lung cancer [1, 2].
The discovery of the existence of a neoplastic counterpart of normal stem cells (SCs) that is responsible for the onset and development of tumours opens new pathogenetic and therapeutic approaches. Cancer initiating cells (CICs) better define than cancer stem cells a selected population of cancer cells that develops tumours upon serial xenotransplantation in immunosuppressed animals [3, 4]. These experiments demonstrated the self-renewal ability of CICs and revealed that CIC-derived tumours recapitulate the heterogeneous phenotype of the tumour of origin implying the differentiating capacity of these cells [3, 4]. The demonstration of CICs has also been based on similarities with normal stem cells (SCs). The niche, where SCs are protected from insults and through asymmetric and symmetric divisions determine their fate, represents one such relevant similarity [5, 6]. Thus, fundamental processes to maintain tissue homoeostasis, such as self-renewal, stemness and differentiation, preferentially occur within these niches. Endothelial cells (ECs) and stromal cells constitute an essential component of the niche and by cell-to-cell interaction and the secretion of multiple factors regulates SC function [7, 8].
The role of tumour stroma in carcinogenesis has been repeatedly proposed [8, 9]. Owing to the limitations in the precise phenotypic assessment, stromal fibroblasts or mesenchymal-like cells show some variability between tumours [10]; however, the clinical relevance of this cell population within tumours is undoubted. It has been shown in several cancers that the quantity of stromal cells and the expression levels of metalloproteinases correlated with a poor prognosis [11, 12]. Similarly, the risk to develop cancer is correlated to increased stromal adipose tissue [13]. Interestingly, the relationship between neoplastic cells and the cellular microenvironment may be bidirectional: as stromal cells are able to support the growth and renewal of CICs, CICs can contribute to the maintenance of the niche [6–8].
The understanding of these cellular events represents a significant advance in cancer biology and the clinical implication of these observations is unquestionable. For example, resistance of lung cancer to conventional or target therapies has extensively focused on tumour cells carrying epidermal growth factor receptor (EGFR) mutations [14] or mesenchymal-epithelial transition receptor amplification [15] that confers resistance to EGFR inhibitors in patients with non-small-cell lung cancer (NSCLC). However, therapeutic resistance has been recently attributed to the tumour environment indicating the importance of supportive elements of neoplastic cells on the natural history of lung cancer [16].
Our preliminary success in isolation and expansion of epithelial and stromal cell populations from the normal lung [17] prompted us to attempt to identify this cell population in neoplastic tissue and to investigate its potential implication in NSCLC.
The aim of the present study was to characterize the biological properties and in vivo tumourigenic role of mesenchymal stromal cells obtained from NSCLC and termed human lung cancer mesenchymal cells (hLc-MCs). Due to our difficulty to reproducibly grow CICs from epithelial cells freshly isolated from samples of lung cancer as a neoplastic cell target for the supportive action of hLc-MCs, a cell line of human NSCLC (Calu-3) was selected. Thus, the term cancer-propagating or cancer-maintaining cell [3] more accurately describes the characteristics of our tumourigenic cell population, at least in the context of xenotransplantation models.
Our results strongly support the notion that the tumour microenvironment plays a fundamental role in lung carcinogenesis and represents a potential target of new customized therapeutic strategies.
MATERIALS AND METHODS
Patient population
The study was performed on 77 patients affected by lung cancer, admitted to the Unit of Thoracic Surgery, University of Parma, undergoing lung resections and enrolled after informed consent for the employment of biological samples for research purposes. Human samples were obtained by means of a protocol approved by the Institutional Review Board and by the local Ethical Committee (Prot. No. 31231). The patient population (Table 1) consisted of 46 males and 31 females, aged between 42 and 88 years. Previous malignancies occurred in 21 subjects and were represented by colon adenocarcinoma (23.8%), bladder and prostate cancer (28.4%), breast cancer (9.5%), melanoma and other malignancies (38.3%). At the time of the diagnosis of NSCLC, 43% of patients had previously received chemotherapy or radiation therapy whereas hormonal treatment was instituted in 9.5% of patients.
. | No. of patients . |
---|---|
Age, years | |
Median | 68 |
Range | 42–88 |
Sex | |
Male | 46 |
Female | 31 |
Smoking history | |
Smoker (ex-smoker) | 69 (40) |
Pack/years | 59 |
Non-smoker | 8 |
Previous malignancy | |
Yes | 21 |
No | 56 |
Neoadjuvant chemotherapy | |
Yes | 3 |
No | 74 |
Histotype | |
Adenocarcinoma | 53 |
Squamous cell carcinoma | 24 |
Pathological staging | |
Ia | 25 |
Ib | 18 |
IIa | 11 |
IIb | 9 |
IIIa | 14 |
. | No. of patients . |
---|---|
Age, years | |
Median | 68 |
Range | 42–88 |
Sex | |
Male | 46 |
Female | 31 |
Smoking history | |
Smoker (ex-smoker) | 69 (40) |
Pack/years | 59 |
Non-smoker | 8 |
Previous malignancy | |
Yes | 21 |
No | 56 |
Neoadjuvant chemotherapy | |
Yes | 3 |
No | 74 |
Histotype | |
Adenocarcinoma | 53 |
Squamous cell carcinoma | 24 |
Pathological staging | |
Ia | 25 |
Ib | 18 |
IIa | 11 |
IIb | 9 |
IIIa | 14 |
. | No. of patients . |
---|---|
Age, years | |
Median | 68 |
Range | 42–88 |
Sex | |
Male | 46 |
Female | 31 |
Smoking history | |
Smoker (ex-smoker) | 69 (40) |
Pack/years | 59 |
Non-smoker | 8 |
Previous malignancy | |
Yes | 21 |
No | 56 |
Neoadjuvant chemotherapy | |
Yes | 3 |
No | 74 |
Histotype | |
Adenocarcinoma | 53 |
Squamous cell carcinoma | 24 |
Pathological staging | |
Ia | 25 |
Ib | 18 |
IIa | 11 |
IIb | 9 |
IIIa | 14 |
. | No. of patients . |
---|---|
Age, years | |
Median | 68 |
Range | 42–88 |
Sex | |
Male | 46 |
Female | 31 |
Smoking history | |
Smoker (ex-smoker) | 69 (40) |
Pack/years | 59 |
Non-smoker | 8 |
Previous malignancy | |
Yes | 21 |
No | 56 |
Neoadjuvant chemotherapy | |
Yes | 3 |
No | 74 |
Histotype | |
Adenocarcinoma | 53 |
Squamous cell carcinoma | 24 |
Pathological staging | |
Ia | 25 |
Ib | 18 |
IIa | 11 |
IIb | 9 |
IIIa | 14 |
The smoking status, histology and pathological staging of the disease are given in Table 1.
Cell isolation
Lung tissue was collected and transferred to the Department of Pathology, where, under sterile conditions, fragments of neoplastic lung tissue were sampled by the medical staff ensuring the priority of their use for diagnostic purposes. Sampling of neoplastic tissue was confirmed by subsequent histological analysis.
Cancer fragments of about 1-2 gr. were minced using surgical scissors and the micro-fragments put in a solution of collagenase/dispase (1 mg/ml) (Roche) for 75 min in a shaking bath at 37°C. The digested material was then purified from debris using a nylon filter with pores of 100 µm. The cell suspension was centrifuged at 240 g for 5 min. The pellet was then resuspended in culture medium dulbecco modified eagle medium (DMEM) (Sigma-Aldrich) added with 10% fetal bovine serum (FBS) and 1% pen-strep (P/S) and seeded in six well plates at 37C under 5% CO2 atmosphere.
Twenty-four hours after plating, the debris and the non-adherent cells (erythrocytes, leucocytes and death cells) were removed by washing twice with phosphate-buffered saline (PBS); fresh culture medium was then added to cell culture. The cell monolayer was observed daily using an inverted microscope (Olympus CK40, Japan) and fresh culture medium was changed twice a week.
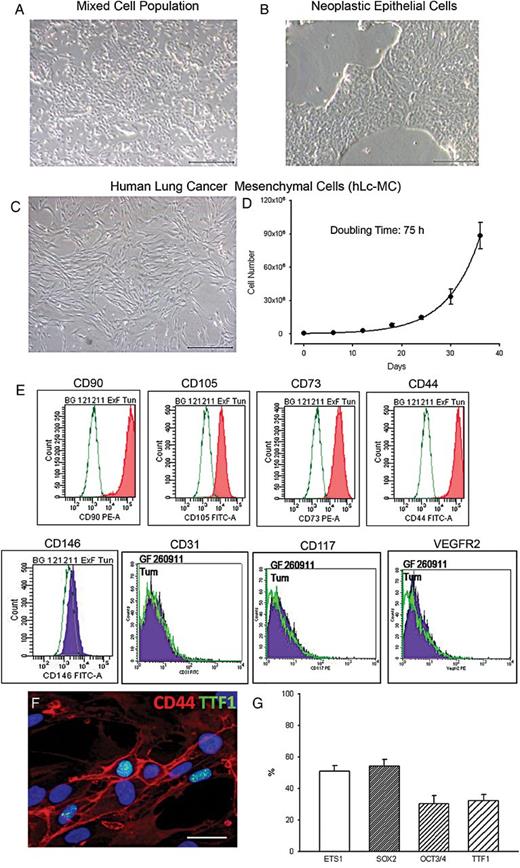
Characterization of human lung cancer mesenchymal cells (hLc-MCs). Phase contrast images of cell cultures documenting a mixed cell population (A) from which tumour epithelial cells (B) and mesenchymal cells (hLc-MCs, (C)) are isolated and expanded from samples of human lung cancer. The growth kinetic curve of hLc-MCs is shown in (D). An example of the immunophenotypic characterization of hLc-MCs by fluorescence-activated cell sorting analysis is illustrated in (E). (F) The nuclear expression of thyroid transcription factor 1 (TTF1) (green) in cultured CD44-positive (red) hLc-MCs is shown by immunofluorescence. Nuclei are recognized by the blue fluorescence of 4′,6-diamidino-2-phenylindole. (G) Bar graph of the quantification of ETS1, sex determining region Y-box 2, octamer-binding transcription factor 3/4 and TTF1 immunolabelling in hLc-MCs. Scale bars: A, B and C = 500 µm; F = 30 µm.
This approach made it possible, in >80% of lung samples, to enrich a population of stromal cells with mesenchymal features, as documented by subsequent immunophenotypic characterization, that were defined hLc-MCs.
Fluorescence-activated cell sorting analysis
hLc-MCs were harvested from culture flasks by slight trypsinization and suspended at a density of 1 × 106 cells/ml in sterile buffer.
To cell suspensions, 1 µg/106 cells of fluorochrome-conjugated [fluorescein isothiocyanate (FITC) or phycoerythrin] monoclonal antibodies with determined specificity against SCs antigens (CD117, CD133 and CD34), mesenchymal cell surface antigens (CD90, CD105, CD73, CD13 and CD44) and hematopoietic cell surface antigens (CD45) was added. Cell viability was assessed by adding to each cell suspension the DNA dye 7-amino actinomycin D (7AAD), while as negative controls fluorochrome-conjugated antibodies with irrelevant specificity was used.
Cells were analysed by a fluorescence-activated cell sorting (FACS)-Diva software (FACScanto II, BD Bioscence) acquiring at least 10 000 events. The percentage of cells positive for each surface antigen considered was obtained by subtracting the corresponding values of negative controls. The results were expressed as a mean ± standard deviation of data collected from the FACS analysis of eight hLc-MCs lines.
Immunocytochemistry
CD44, Vimentin, OCT 3/4, sex determining region Y-box 2 (SRY-box 2), ETS1 and thyroid transcription factor 1 (TTF1) were detected in hLc-MCs using immunofluorescence staining methods. Cells seeded at 10 000/cm2 concentration on suitable chamber slides were fixed with 4% paraformaldehyde and exposed to primary antibodies.
Samples were incubated with primary antibodies against CD44 (HCAM IM7, Santa Cruz, sc18849), Vimentin (Millipore, CBL202), SRY-box 2 (Abcam, ab75485), octamer-binding transcription factor (OCT3/4) (Sigma-Aldrich, O8389) ETS1 (Abcam, ab59217) and TTF1 (Abcam, ab76013). Specific FITC-, tetramethylrhodamine isothiocyanate (TRITC-) or Cy5 conjugated (Sigma-Aldrich) secondary antibodies were employed. Nuclei were counterstained by 4′,6-diamidino-2-phenylindole (DAPI).
The quantitative assessment of these markers was conducted on 10 hLc-MCs lines and included the analysis of a minimum of 1000 to a maximum of 10 000 cells for each epitope and expressed as a percentage. Negative controls were represented by samples in which the primary antibody was omitted from the immunoreaction.
Preparation of conditioned medium from hLc-MCs and Calu-3
Calu-3, a human lung adenocarcinoma cell line (ATCC® HTB-55™) and hLc-MCs were plated at a density of 40 000 cells per cm2 and incubated in complete growth medium for 1 day. Adherent cells were washed three times with PBS, and the medium was replaced with serum-free DMEM. After 48 h of culture, the supernatant was centrifuged at 240 g for 5 min, filtered and freshly used or stored at −20°C. By this procedure, conditioned medium (CM) from hLc-MCs cell lines isolated from NSCLC samples of 3 patients was then employed for our experiments.
Cell exposure to conditioned medium
hLc-MCs or Calu-3 were seeded in a 96 wells plates at a density of 10 000 cells/cm2 in complete growth medium DMEM (Sigma-Aldrich) added with 10% FBS, 1% NEAA and 1% P/S at 37°C in a 5% CO2 atmosphere. After 48 h, cells were reciprocally exposed for 24 h to CM either from hLc-MCs CM or Calu-3.
Morphology, viability and adhesion (Crystal Violet assay) were then evaluated on CM-exposed cells.
Crystal violet assay
Briefly, cells were fixed in 4% paraformaldehyde and stained with 0.2% crystal violet (CV) in PBS. The unbound dye was removed by washing with water. Bound crystal violet was solubilized with 0.2% TritonX-100 in PBS. Light extinction, which increases linearly with cell number, was analysed at 595 nm with Microplate Reader (Model 550, Bio-Rad).
DiI cell labelling
Cell Tracker ChloroMethyl-DiI (Invitrogen, C-7001) is a DiI derivative that is somewhat more water-soluble than DiI, thus facilitating the preparation of staining solutions for cell suspensions. Unlike other membrane stains, this label is well retained in the cells throughout several mitotic divisions and cell-to-cell contact does not allow dye diffusion.
hLc-MCs were incubated in 1–2 µM working solution for 15 min at 37°C, and then for an additional 15 min at 4°C.
Coculture of hLc-MCs with Calu-3
After DiI labelling, hLc-MCs were seeded on chamber slides at a 1:1 concentration with Calu-3, reaching the overall density of 20 000 cells/cm2, and cultured for 5 days in complete growth medium.
To assess the effect of coculture on hLc-MCs and Calu3, samples were incubated with anti-Vimentin (VIM, Ventana ROCHE) and anti-Cytokeratin (CK, Ventana ROCHE) antibodies, followed respectively by universal alkaline Phosphatase Red Detection Kit (Ventana, ROCHE) and simultaneously universal 3,3′-Diaminobenzidine (DAB) Detection Kit (Ventana, ROCHE). Finally, cells were counterstained with attenuated concentration of Mayer's haematoxylin to preserve intact the chromatic signals of the two reactions. By this approach, the red labelling of alkaline phosphatase corresponds to Vimentin staining of hLc-MCs, whereas CK-positive Calu-3 resulted in a brownish colour by peroxidase staining (DAB).
Immunofluorescence analysis was also performed to investigate stromal-to-cancer cell interaction. Briefly, samples were stained with primary antibodies anti-Vimentin (Millipore, CBL202), anti-E-Cadherin (E-CAD, DAKO) and Ki67 (Clone MIB-1, IR626, DAKO) followed by specific fluorescent secondary antibodies (Sigma-Aldrich). Nuclei were counterstained by DAPI.
Experimental model of xenotransplantation
The study was carried out in strict accordance with the recommendations in the Guide for the Care and Use of Laboratory Animals of the National Institute of Health. The protocol conforms to the National Ethical Guidelines of the Italian Ministry of Health.
To test the tumourigenic potential of hLc-MCs and Calu3, Balb-c NUDE female mice (CAnN.CG-Foxn1nu/Crl, Strain Code 194 Homozygous, Charles River Laboratory, Calco, Italy) were subcutaneously injected with 1 × 106 (n = 4) or 2.5 106 (n = 4) Calu-3, able to reproducibly induce xenografted tumours. Calu-3 were injected alone or in combination with 1 × 106 (n = 5) and 2.5 × 106 (n = 5) hLc-MCs from three different male donors, respectively. In four additional animals, different doses (1 × 106n = 2; 2.5 × 106n = 2) of hLc-MCs were injected alone.
Each animal was anaesthetized with inhalation of ether vapour; cell pools were suspended in 100 µl of saline buffer and the solution was added to an equal volume of Matrigel® (BD Bioscence, Franklin Lakes, NJ, USA) and subcutaneously injected on the right flank of each mouse using a 1 ml-siringe.
In vivo cell tracking
Quantum dots labelling
To track hLc-MCs within xenografted tumours, cells were pre-labelled with Quantum dots (QDots), semi-conductive nano-particles that, depending on their size, emit different colours far from the excitation length of ultraviolet (UV) light. hLc-MCs were loaded with QDots 585 nm, characterized by yellow fluorescent emission signals under UV excitation.
Specifically, cells were labelled following the manufacturer's suggestions with the Qtracker® Cell Labeling Kit (Invitrogen). Once inside the cells, the Qtracker® label provides intense, stable and specific fluorescence that can be traced through several generations.
FISH analysis of human sex chromosomes
Fluorescence in situ hybridization (FISH) analysis of human sex chromosomes was used to detect the injected human cells within the tumour xenograft samples.
A mix of human-specific chromosome enumeration probes, complementary to the centromeric region of the X chromosome (Cy3-Alpha-Satellite DNA, locus X p11.1-q11.1, Vysis, USA) and the Y chromosome (FITC-Satellite III DNA, locus Yq12, Abbott, USA) was employed.
Nuclei were counterstained with DAPI, and the analysis was performed using a fluorescence microscope (Olympus BX60) with Z-stack equipment at 100× magnification and an oil immersion objective.
Morphometric analysis of tumour xenografts
Sections from tumour nodules were stained with haematoxylin and eosin (H&E) or Masson's trichrome or used for immunohistochemistry.
To compare the histological features of xenografted tumours with the primary human cancer, a histological evaluation was performed on the biopsy of the corresponding patient.
The morphometric evaluation of nodules composition in terms of area occupied by collagen or neoplastic tissue was performed on Masson's trichrome-stained sections. Microphotographs of the entire nodule were captured by an optical microscope connected to a digital camera at a final magnification of 40×. Subsequently, the area occupied by collagen or neoplastic tissue was evaluated by the image analysis software (Image pro-plus 4.0, Media Cybernetics, USA). A macroscopic image of the whole nodule was captured to determine the total area. Moreover, within the neoplastic tissue, the percentage of epithelial glandular cells, including luminal areas, with respect to the total area of the nodule was measured.
Immunohistochemical analysis of tumour xenografts
Samples were incubated with primary antibodies against CK (Clones AE1/AE3, M3515, DAKO) and the mitotic marker phosphorylated Histone H3 (phH3, 06-570, Millipore) followed by specific TRITC- or FITC-conjugated secondary antibodies (Sigma-Aldrich). Nuclei were counterstained by DAPI.
Statistical analysis
The SPSS statistical package was used (SPSS, Chicago, IL, USA). The normal distribution of variables was checked by means of the Kolmogorov–Smirnov test. Statistics of variables included mean ± standard error (S.E.M.), paired Student's t-test, one-way analysis of variance (post hoc analyses: The Bonferroni test or Games–Howell test, when appropriate). A statistical significance was set at P < 0.05 or P < 0.001.
RESULTS
Characterization of hLc-MCs
In nearly 80% (61/77) of tumour samples a population of stromal cells was successfully obtained and separated from neoplastic epithelial cells (Fig. 1A–C). Following tissue digestion, within 5 days, an adherent cell population named hLc-MCs was isolated either by differential trypsinization or EpCAM microbeads-based negative selection. These cells could be expanded for several passages (p16) before showing a decline in growth properties. However, as much as nearly 30 × 106 and 90 × 106 cells were typically obtained after 5 and 6 passages, respectively, from each primary culture preparation (Fig. 1D).
The morphology and growth characteristics strongly suggesting a mesenchymal phenotype were supported by the immunophenotypic analysis performed by both FACS (Fig. 1E) and immunocytochemistry (Fig. 1F). Specifically, FACS documented that hLc-MCs consistently expressed CD90, CD105, CD73 and CD44 (Table 2) whereas CD34, CD31, CD45, CD106 and CD13 were undetectable.
Quantification of the fraction of hLc-MCs expressing mesenchymal associated markers as measured by FACS
. | Mean, % ± SE . |
---|---|
CD90 | 87.50 ± 8.61 |
CD105 | 80.83 ± 9.11 |
CD73 | 91.50 ± 4.52 |
CD44 | 97.00 ± 2.68 |
. | Mean, % ± SE . |
---|---|
CD90 | 87.50 ± 8.61 |
CD105 | 80.83 ± 9.11 |
CD73 | 91.50 ± 4.52 |
CD44 | 97.00 ± 2.68 |
hLc-MCs: human lung cancer mesenchymal cells; FACS: fluorescence-activated cell sorting.
Quantification of the fraction of hLc-MCs expressing mesenchymal associated markers as measured by FACS
. | Mean, % ± SE . |
---|---|
CD90 | 87.50 ± 8.61 |
CD105 | 80.83 ± 9.11 |
CD73 | 91.50 ± 4.52 |
CD44 | 97.00 ± 2.68 |
. | Mean, % ± SE . |
---|---|
CD90 | 87.50 ± 8.61 |
CD105 | 80.83 ± 9.11 |
CD73 | 91.50 ± 4.52 |
CD44 | 97.00 ± 2.68 |
hLc-MCs: human lung cancer mesenchymal cells; FACS: fluorescence-activated cell sorting.
Nearly 30% of hLc-MCs showed the receptor 2 for vascular endothelial growth factor (KDR) while CD146 and the SC associated antigens CD117 and CD133 were present in a small fraction of cells. The presence of CD44 in a large number of cells was also documented by immunocytochemistry (Fig. 1F) that also excluded the expression of epithelial-associated antigens as EpCAM and Pan-Cytokeratin (not shown).
Interestingly, transcription factors associated with stemness (Oct3/4 and SRY-box 2), proliferation and carcinogenesis (ETS1) and bronchoalveolar differentiation (TTF1) were variably expressed in nuclei of hLc-MCs (Fig. 1F). While ETS1 and SRY-box 2 involved ∼50% of cells, OCT3/4 and TTF1 were present in nearly 30% of hLc-MCs (Fig. 1G). The latter finding indicates that, in agreement with similar observations made in other organs, tissue lineage commitment is retained by stromal cells.
Interaction of hLc-MCs with human lung cancer cells
To investigate the role of the tumour stromal compartment in cancer growth, in vitro studies were performed utilizing two different approaches.
We first asked whether short exposure to CM from hLc-MCs or NSCLC cell (Calu-3) culture could reciprocally affect cell growth.
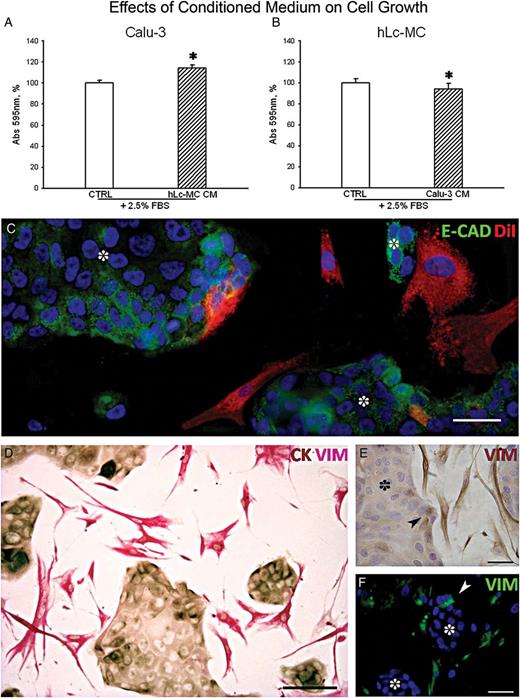
In vitro analysis of the interaction between hLc-MCs and human lung adenocarcinoma. Quantitative estimation of cell growth after short (24 h) exposure of Calu-3 to serum (FBS) supplemented conditioned medium (CM) from hLc-MCs (A) and the reciprocal outcome of CM from Calu-3 cells on hLc-MCs (B). Data are expressed as fraction of crystal violet absorbance at 595 nm (*P < 0.001). (C) Representative image of the typical membrane pattern of E-Cadherin (E-CAD, green) expression in clustering Calu-3 cells (dot) surrounded by DiI prelabelled hLc-MCs (red). (D) Double immunostaining documenting Vimentin (VIM, pinkish) labelled hLc-MCs surrounding CK-positive (brownish) Calu-3 clusters. Vimentin expression in cancer cells (arrowhead) located at the edge of Calu-3 clusters (dot) is shown by both immunoperoxidase in E (VIM, brownish) and immunofluorescence in F (VIM, green). (C and F) Nuclei are recognized by the blue fluorescence of DAPI. Scale bars: C, E and F = 50 µm; D = 100 µm.
Further experiments on stromal-to-cancer cell interaction were carried out by setting cocultures of the two cell populations. To this end, adherence junctions were analysed by immunofluorescence showing the membrane pattern of E-Cadherin (E-CAD) in clustering Calu-3 cells bordered by DiI- prelabelled hLc-MCs (Fig. 2C). Double immunostaining to detect Vimentin-positive hLc-MCs and CK-positive epithelial cancer cells was performed on 1:1 hLc-MC:Calu-3 cells cocultured for 5 days (Fig. 2D). Vimentin-positive stromal cells typically surrounded CK-positive cancer cells with which, at times, were in close contact. After 5 days of coculture, although few DiI-labelled stromal cells could be found within the Calu-3 cell clusters, a defined structural junction between hLc-MCs and epithelial cancer cells was undetectable, at least by the analysis of E-CAD. Further investigations on the presence of N-Cadherin, connexins and integrins are required to address this issue.
Intriguingly, Vimentin immunostaining was sometimes present in cells at the periphery of Calu-3 cell clusters (Fig. 2E), suggesting the possibility of the occurrence of epithelial-to-mesenchymal transition (EMT). This latter finding, as better defined by immunofluorescence, was confirmed by the expression of Vimentin in cancer cells mostly located on the outer layer of Calu-3 cell clusters (Fig. 2F). Although this relevant phenomenon involved in cancer progression and metastatization was not the aim of our study, we are strongly encouraged to use multiple immunolabelling of the involved epitopes to dissect the basic events implicated in EMT.
The effect of coculture on cell growth was investigated by the detection of Ki67, which labels the entire cell cycle progression including the mitotic phase. The growth advantage of Calu-3 cells cocultured for 5 days with hLc-MCs was clearly documented by a 10-fold higher nuclear expression of Ki67 in cancer cells (data not shown).
In vivo tumourigenic role of hLc-MCs
Experimentally, tumour xenografts induced in immunosuppressed mice allowed us to investigate the role of hLc-MCs on tumour formation and in vivo interactions with cancer cells.
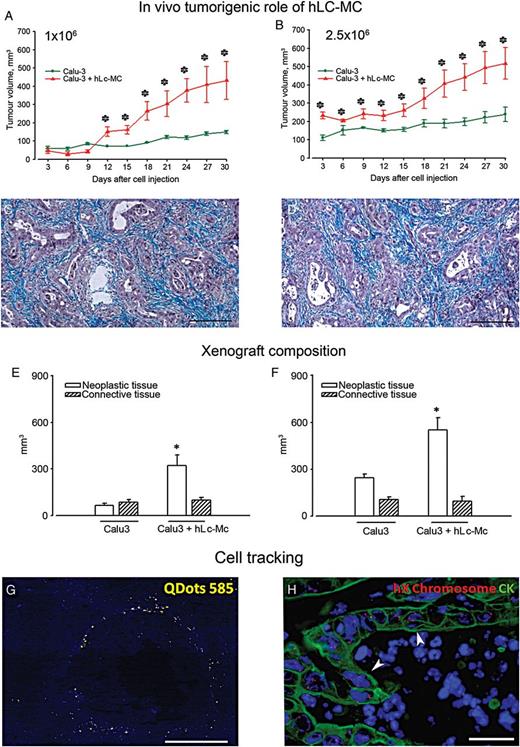
In vivo tumourigenic role of hLc-MCs. Line graphs highlighting the time dependent tumour growth following the injection of Calu-3 cells (green lines) or Calu-3 + hLc-MCs (red lines) at 1 × 106 (A) and 2.5 × 106 (B) cell dosage (*P < 0.05). Masson's trichrome stained sections of tumours generated by two doses of Calu-3 cells are shown in (C and D), respectively, to illustrate the neoplastic glandular epithelium (magenta) surrounded by connective tissue (bluish). Quantification of tissue composition in tumour xenografts as a result of the injection of 1 × 106 (E) and 2.5 × 106 (F) doses of Calu-3 cells alone or Calu-3 + hLc-MCs. *P < 0.001. (G) unstained section of Calu-3 + hLc-MCs derived tumour xenografts excited by ultraviolet light. Yellow fluorescent signals correspond to Quantum Dots (QDots) 585 nm pre-labelled hLc-MCs localized in stromal tissue (blue autofluorescence) surrounding empty black spaces, likely representing neoplastic glandular structures. (H) Fluorenscence in situ hybridization analysis of human X chromosome (red dots) in tumour xenografts generated by the coinjection of Calu-3 cells with hLc-MCs. X chromosome ploidy formation (arrowheads) in CK-positive (green) Calu-3 cells organized in neoplastic glands is apparent. Blue fluorescence corresponds to DAPI staining of nuclei (scale bars C and D = 200 µm; G = 150 µm; H = 40 µm).
No injection-related death occurred in mice and the autopsy performed after sacrifice did not show any metastatic site in the liver, lung, brain, spleen and kidney.
Characterization of tumour xenografts
Tumours generated either by Calu-3 or Calu-3 + hLc-MCs showed the typical architectural organization of human adenocarcinoma including single or multiple layers of proliferating CK-positive epithelial cells constituting glandular structures surrounded by connective tissue (Fig. 3C and D). Importantly, these findings closely mimic the histological features observed in biopsies from the corresponding primary NSCLC.
A morphometric evaluation of tumour samples was performed on H&E and Masson's trichrome stained sections. The contribution of neoplastic cells and interstitial compartments to the composition of tumour xenografts was assessed both as mm3 and volume fraction (%) on histological sections to compute the real effects of cell injection on neoplastic cells in different experimental animal groups. Quantitatively, in nodules formed by the injection of 1 × 106 Calu-3 cells, 56.5 ± 9.6% (84 ± 17 mm3) of the volume was occupied by connective tissue and 43.5 ± 6.4% (65 ± 11 mm3) by neoplastic structures (Fig. 3G). The addition of 1 × 106 hLc-MCs resulted in a statistically significant 3.8-fold increase (P < 0.001) in neoplastic volume, which reached 75.4 ± 3.6% (322 ± 27 mm3) without significantly affecting the amount of interstitium (24.5 ± 3.6%; 97 ± 18 mm3) (Fig. 3E). A similar effect of the coinjection of hLc-MCs with Calu-3 cells was documented when a 2.5 × 106 cell dosage was analysed (Fig. 3F). In particular, the morphometric analysis of tumours formed by the injection of hLc-MCs + Calu-3 cells showed that the increase in tumour volume was mainly due to the expansion of neoplastic tissue (2.2-fold; P < 0.01) while collagen deposition occupied 30.4 ± 6.2% (106 ± 15 mm3) and 13.9 ± 2.8% (97 ± 17 mm3) of xenografts formed by Calu-3 and Calu-3 added with hLc-MC, respectively.
Immunofluorescence and FISH analysis were performed on sections of tumour xenografts to study the fate and distribution of Calu-3 cells and hLc-MCs following their coinjection. QDots prelabelling of hLc-MCs allowed us to document that these cells were preferentially located at the periphery of rounded glandular structures (Fig. 3G).
The unequivocal evidence of the human origin of induced tumours was evaluated by FISH analysis of human sex chromosomes. The typical X polysomy associated with the Calu-3 cell line was present in vivo in epithelial cells constituting the glandular pattern of adenocarcinoma (Fig. 3H). Interestingly, the presence of the Y chromosome in xenografts generated by the coinjection of hLc-MCs from a male NSCLC donor was mostly observed in a limited number of cells surrounding neoplastic structures (not shown).
Thus, hLc-MCs promote the tumourigenic potential of NSCLC cells in vivo, strongly suggesting that the supportive role of stromal cells is essential for the development of human lung cancer.
DISCUSSION
The present study strongly supports the fundamental role played by tumour stromal compartments in lung carcinogenesis. The possibility to expand and characterize a population of mesenchymal cells (hLc-MCs) in a significant number of samples from primary human NSCLC allowed us to document the ability of these cells to promote in vitro and in vivo cancer growth. Xenografted tumours generated in immunosuppressed mice by Calu-3, a human adenocarcinoma cell line, doubled in size when equal amounts of hLc-MCs were co-injected. Cell tracking showed that hLc-MCs surrounded adenocarcinoma glands 4 weeks after injection. Studies on CM and cocultures also suggest that soluble factors secreted by hLc-MCs are implicated in the development of NSCLC.
Lung cancer remains the first cause of death among all human malignancies. The identification of several aetiological factors involved in NSCLC and new promising therapeutic strategies have not been able to significantly change the prognosis of this devastating disease [1, 2].
The molecular and biological mechanisms responsible for the generation and amplification of lung cancer cells are still partially unknown. The identification of a subpopulation of cancer cells with SC characteristics, called CICs, responsible for cancer growth and metastasis, opens new therapeutic targets potentially able to influence the development of the tumour [3, 4]. The current knowledge on CICs and their dominant role in cancer progression may further explain the high aggressiveness and invasive ability of some cancer types. It is also known that both mesenchymal and vascular stromal cells function as supportive elements for CICs activity [5–7].
The local microenvironment is involved in tumour initiation, as mutations in the stromal compartment may be able to alter the quiescence state of tissue SCs. Examples of such a contention have been demonstrated by inducing mutations either in osteo-progenitors resulting in myeloid leukaemia or on dermal fibroblasts leading to skin tumours [18, 19]. Moreover, recent data have shown that the influence of stromal cells on tumour growth is not a passive phenomenon [20]. Indeed, stromal and neoplastic cells are subjected to similar physical and biologic stresses implicating a necessary adaptation of stromal cells to unfavourable micro-environmental changes, such as hypoxia, metabolic derangement and therapy-mediated injury [20].
These observations propose stromal cells as central players of important scenarios: role in supporting tumour growth, and as targets for presensitization therapy and drug resistance, ‘sanctuaries’ of CICs.
The theory that tumours depend on a small population of CICs for their long-term growth and metastasis has profound implications for our understanding of cancer and its treatment. The assumption can be made that therapeutic failures in lung cancer are attributable to the failure of targeting CICs and their sanctuaries.
As in other tissues, MCs have also been demonstrated in the lung [21]. Interesting observations have drawn attention to the close relationship that exists in the lung between CICs and resident MCs, identifying the latter as a possible therapeutic target or vehicle to kill cancer cells and prevent the escape mechanisms by which the niche of neoplastic clones are protected, resulting in therapeutic failure [22].
Mesenchymal stromal cells have multilineage differentiation capacity limited to tissues of mesodermal origin. The discrepancy between nomenclature and biological characteristics seems to be more due to differences in the assays used in various works, rather than a real coexistence of more SCs of mesenchymal origin. A gradient exists in the differentiative potential of MCs and tissue factors appear to be able not only to increase the proliferation, but also to interfere with the differentiation of MCs, keeping them in the state of multipotentiality [21]. The documentation of the present investigation that hLc-MCs express several stemness-related markers as SRY-box 2 and OCT3/4 is in agreement with these observations.
According to the literature, MCs are devoid of unique specific markers, and so are identified through the analysis of a complex immunophenotype, which includes the lack of antigens typical of tissue SCs (CD45, CD34 and CD14) and the expression of a series of surface molecules, such as CD90, also called Thy-1, CD105 or endoglin, CD29 or β subunit of the receptor for fibronectin, CD44 or jaluronic acid receptor and CD73 or 5′-nucleotidase.
In agreement with these observations, our cell population of hLc-MCs isolated from NSCLC samples, even after in vitro expansion, maintained the expression of surface antigens such as CD105, CD90, CD73 and CD44. Importantly, we have previously documented that these markers are uniformly and strongly expressed on MCs isolated from tissues of different origin such as the heart and bone marrow [23]. These cells share, in vitro, many of the characteristics of mesenchymal SCs from bone marrow: adhesion to plastic, morphology, formation of colony-forming units fibroblast, some surface markers and differentiation potential into osteogenic, adipogenic and chondrogenic lineages following appropriate stimuli. Importantly, the consistent expression of TTF1 in our preparations of hLc-MCs isolated from NSCLC implies a constitutive lung-specific lineage commitment. This phenomenon has been repeatedly reported in MCs from other tissues [23].
In agreement with experimental studies supporting the role of tumour-associated stromal cells in stimulating tumour growth [5, 6, 8, 9], we have shown that hLc-MCs in vivo and in vitro are able to promote tumour development. Our study confirmed the role of MCs in fostering the growth of human NSCLC, suggesting a translational implication of these cells in tumour progression. On the other hand, some observations have proposed an inhibitory effect of healthy stromal cells on cancer cells [24]. However, cocultures and coinjections of hLc-MCs with adenocarcinoma cells showing a favourable effect on tumour growth do not confirm these data. Stromal cells from adenocarcinoma are phenotypically different from fibroblasts of normal bronchial parenchyma [21]. Thus, the possibility of the presence within the lung of two biologically distinct cell populations of stromal cells with opposite effects on cancer cells is highly speculative.
An additional biological characteristic of MCs, which makes them an attractive candidate for cell therapy, is their ability to at least partially avoid the recognition of immune cells. MCs are poorly immunogenic, and may escape the cytotoxic T and natural killer cell lysis. These cells are transplantable between human leukocyte antigen-mismatched individuals without the need for immunosuppressive therapy [22].
While mesenchymal cells infrequently form intercellular junctions, epithelial cells are characterized by well-developed intercellular contacts. EMT is a critical process activated during embryonic development and when compared with adult-differentiated epithelial cells, and in particular to lung cancer progression, some differences have been reported [25].
Two main phenomena occur in EMT represented by the loss of E-cadherin, a cell-to-cell junction and the expression of Vimentin, an intermediate motile filament [25]. Numerous studies have shown that the inverse relation between these two markers leads to tumour progression. Moreover, EMT in cancer cells has been linked to the acquisition of stemness properties and to a decrease of drug sensitivity [25], advancing the hypothesis that a cancer cell undergoing EMT acquires a metastatic CIC phenotype. Although EMT was not a principal aim of the present study, our in vitro assessment of the expression of E-CAD and Vimentin in cocultures of epithelial cancer cells and hLc-MCs opens the possibility to model the regulation of these two molecules in specific cellular targets.
Finally, recent observations have shown that drug resistance may be environmentally mediated. By this mechanism, tumour cells are protected from chemotherapy, radiotherapy or receptor-mediated cell death [14–16]. Soluble factors present in the tumour microenvironment and changes in tumour to stromal cell adhesion activate signalling events that are rapidly induced to initiate this form of drug resistance. Thus, cytokines, chemokines and growth factors secreted by tumour stroma and cell adhesion of tumour cells to stromal fibroblasts or to components of the extracellular matrix have been implicated in drug resistance.
In conclusion, mesenchymal cells resident in NSCLC promote cancer growth and represent a promising cellular substrate to dissect the precise role of the tumour microenvironment in lung carcinogenesis and to develop new target therapies.
Funding
This work was supported by EU FP-7 BIOSCENT project (ID number: 214539).
Conflict of interest: none declared.
ACKNOWLEDGEMENTS
The authors thank Gabriella Becchi, Nicoletta Campanini and Emilia Corradini for technical assistance and immunohistochemistry.
REFERENCES
Author notes
Presented at the 22nd European Conference on General Thoracic Surgery, Copenhagen, Denmark, 15–18 June 2014.
- cardiac support procedures
- lung
- non-small-cell lung carcinoma
- cell separation
- coculture techniques
- culture media, conditioned
- fluorescence
- mast-cell sarcoma
- transplantation, heterologous
- neoplasms
- lung cancer
- mesenchymal stem cells
- minimally conscious state
- millennium cohort study
- tumor cells, malignant